DOI:
10.1039/C7RA01009E
(Paper)
RSC Adv., 2017,
7, 19604-19610
Near infrared laser-controlled drug release of thermoresponsive microgel encapsulated with Fe3O4 nanoparticles
Received
23rd January 2017
, Accepted 18th March 2017
First published on 3rd April 2017
Abstract
One major issue in thermosensitive drug delivery systems is the remote, repeatable control of temperature in vivo through external stimuli such as light, ultrasound, and magnetic field. In this study, an Fe3O4/p(NIPAM-co-MAA) composite microgel (Nms) was fabricated via copolymerizing NIPAM with MAA in water-containing Fe3O4 nanoparticles modified by oleic acid. The photothermal effect and thermal-responsibility of Nms were investigated by adjusting the amount of MAA and Fe3O4 in the reaction, and the result shows an LCST of 37.2 °C, which could be elevated to 45.8 °C under laser treatment at 808 nm. In the drug release experiment, the data show that the drug can be released at an expected rate by controlling the temperature using an 808 nm laser irradiation in vivo. The release rate was fast and the final cumulative drug release rate increased by about 25%. Thus, these properties of the Fe3O4/p(NIPAM-co-MAA) Nms microgel indicate its promising application in multi-responsive microgels, especially in photothermal drug carriers.
1. Introduction
Stimuli-responsive microgels have been well-studied and widely applied in many fields such as tissue engineering, biomedical implants,1 drug delivery,2 and bionanotechnology,3 owing to their three-dimensional (3D) physical structure,4 good mechanical properties,5 and high water content.6 Especially, thermal-responsive microgels based on poly(N-isopropylacrylamide) (PNIPAM) exhibit a lower critical solution temperature (LCST) at about 32 °C,7 which is approximate to the physiological temperature of the human body.8 Moreover, when the surrounding temperature increases, polymeric microgels undergo a phase transformation from a swollen structure to collapse aggregation,9 which provides an opportunity to load and/or release small molecules and thus potential application in cancer therapy.
However, once heated, PNIPAM microgels collapse from the swollen gel in an uncontrollable way, which limits its application in drug-controlled release. Some other stimuli such as pH (AAc),10 oxidoreduction (GSH),11 and near-infrared (NIR) light (CuS)12 were introduced to adjust the drug release rate. Among all these strategies, NIR absorbing materials can be used as a photothermal ablation agency by transforming light into thermal energy13 to kill cancer cells or release drugs.14 NIR photothermal semiconductor nanocystals such as MoS2,13 WS2,15 and Cu9S5 (ref. 16) have become popular because they are inexpensive and readily available. However, some researchers have reported that the potential toxicity,17 undegradability,18 and low hemolytic activity16 impair their application in biomedicine to some extent. Although Fe3O4 nanoparticles could avoid these problems and effectively absorb NIR light, and have been clinically applied in hyperthermia cancer therapy using NIR,19 poor dispersion stability and easy aggregation usually lead to low activity of Fe3O4 nanoparticles.10 One way to overcome these disadvantages is to put them in a polymeric microgel for the stabilization of Fe3O4 nanoparticles. Moreover, the composite microgel would not only exhibit stronger NIR light absorption but also achieve excellent controlled drug release by adjusting the NIR irradiation power.
Additionally, some similar systems including Au@SiO2/P(NIPAM-co-AAc),14 PANI/PNIPAM/HPC,11 and rNGO@mSiO2@pNIPAM-co-pAAm12 have been reported. Inspired by these composite systems and the related assembly methods, we prepared an Fe3O4/p(NIPAM-co-MAA) microgel by compositing a layer of PNIPAM on the surface of Fe3O4 nanoparticles. As shown in Fig. 1, we first synthesized Fe3O4 nanoparticles modified with oleic acid. After this, the nanoparticles were copolymerized with NIPAM and MAA monomers via polymerization in an emulsion. Finally, the photothermal effect and thermal-responsibility of Fe3O4/p(NIPAM-co-MAA) were investigated by determining its drug control release capacity under 808 nm laser irradiation. The irradiation results indicate that the composite microgel could increase the temperature from 30 to 45.8 °C and the cumulative drug released amount increased by about 25%. The results suggest that the composite microgel Nms exhibits great advantages for photothermal/chemotherapy.19
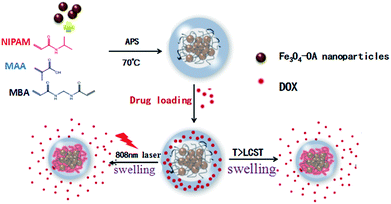 |
| Fig. 1 Schematic for the preparation process and NIR light-triggered drug release of Fe3O4/p(NIPAM-co-MAA) Nms. | |
2. Experimental
2.1 Materials
Ferric chloride hexahydrate (FeCl3·6H2O), ferrous chloride tetrahydrate (FeCl2·4H2O), ammonia water (NH3·H2O), oleic acid (OA), doxorubicin (DOX), absolute ethyl alcohol, sodium hydrogen phosphate (Na2HPO4), sodium chloride (NaCl), and isopropyl alcohol were purchased from Xilong Chemical Co., Ltd (Guangdong, China) and used without any further purification. N-isopropylacrylamide (NIPAM), methacrylic acid (MAA), ammonium persulfate (APS), N,N-methylene bis-acrylamide (MBA), and sodium dodecyl sulphate (SDS) were all analytically pure and were purchased from Aladdin Co., Ltd.
2.2 Synthesis of OA-modified Fe3O4 nanoparticles
Fe3O4 nanoparticles were synthesized by the co-precipitation of iron chloride salts with ammonia.19 In a typical synthesis process,20,21 4.43 g FeCl3·6H2O and 1.625 g FeCl2·4H2O were dissolved in 190 mL deionized water under mechanical stirring at 60 °C under a nitrogen atmosphere. Under the conditions of vigorous stirring, 10 mL NH3·H2O was poured into the abovementioned solution. After stirring for 30 min, the solution was precipitated by centrifugation and black precipitates obtained were washed three times with ethanol and water. Then, the Fe3O4 nanoparticles were redispersed in 180 mL deionized water and heated to 60 °C under the protection of nitrogen gas, and mixture solutions of 0.9 g oleic acid and 3 mL ammonia water were added within 30 min. After this, the solution was heated at 80 °C and precipitation occurred. The precipitate was washed with acetone 3 times and then dried in a vacuum oven at 70 °C for 24 h. The Fe3O4 nanoparticles modified by oleic acid were labelled as OA–Fe3O4.
2.3 Fabrication of p(NIPAM-co-MAA) with OA-modified Fe3O4 nanoparticles
The Fe3O4/p(NIPAM-co-MAA) composite microgel was synthesized via a emulsion polymerization method, as previously described.22 Herein, 0.5 mg of OA–Fe3O4 nanoparticles was mixed with 15 mL sodium dodecyl sulfate (SDS, 1.0 mg mL−1) containing 300 mg N-isopropylacrylamide (NIPAM, monomer), 15 μL methacrylic acid (MAA, monomer), and 10 mg bis-acrylamide (MBA, cross-linker). This mixture was vigorously stirred and ultrasonicated, and a brown emulsion was obtained. This mixture was then heated to 70 °C and bubbled with nitrogen to remove the residual oxygen. Thereafter, 1.0 mL (10 mg mL−1) ammonium persulfate (APS), as an initiator, was rapidly added, and polymerization was allowed to proceed at 70 °C for 6 h. Finally, the obtained Fe3O4/p(NIPAM-co-MAA) composite microgel was further purified (Spectra/Por molecular porous membrane tubing, cut-off 12
000–14
000 Da), dialyzed for four weeks against very frequently changing water at room temperature (25 °C), and then dried under vacuum at 50 °C. This Fe3O4/p(NIPAM-co-MAA) nanocomposite was labeled as Nms.
2.4 DOX loading and controlled release from Fe3O4/p(NIPAM-co-MAA)
The composite microgel (24 mg) was dispersed in 10 mL deionized water. The resulting composite microgel solution was poured into a dialysis bag (cut-off molecular weight of 8000–14
000 Da), the dialysis bag was placed in a 50.0 mL solution containing the anticancer drug DOX, and then left at room temperature for 24 h. A UV-spectrophotometer was used to measure the transmittance of the dialysate at 480 nm and to obtain the loading amount according to the DOX standard curve. Moreover, the products were cleaned using deionized water, which was repeated at least five times until a colorless supernatant was obtained.
For photothermal-triggered release, according to Huang's method,22 1.0 mL PBS (pH = 7.4) containing Nms@DOX (1.0 mg mL−1) in a 10 mL test tube was incubated at 37 °C. At predetermined time intervals, the samples were irradiated using an 808 nm NIR laser with an output power density of 1.0 W cm−2 for a certain period of time. The samples were centrifuged (8000 rpm, 10 min) and DOX was quantified via UV-spectrophotometer analysis using the supernatants. Finally, fresh PBS (5 mL) was added to the residual mixture.
2.5 Characterization
FTIR spectra were obtained at room temperature by a spectrometer (AVATAR 360, Nicolet, USA) and measured in the wavenumber range from 4000 to 400 cm−1 by dispersion in KBr matrices to elucidate the key structural features. The surface of the composite microgel was observed using a field emission electron microscope (SEM) (Helios Nanolab 600i, FEI, USA) after coating with gold.23 Transmission electron microscopy (TEM, JEOL, Japan) images were acquired using a JEOL JEM-1400 TEM operating at the acceleration voltage of 120 kV. The thermoresponsive behavior of the composite microgel was investigated using the transmittance of a temperature-controlled UV-spectrophotometer (TU-1901, Purkinje, China). Drug release data were monitored using a UV-spectrometer at the wavelength of 480 nm. The light scattering (DLS) size of Nms was measured using Zetasizer Nano (Enigma Business Park, Grovewood Road, Malvern, Worcestershire. WR141XZ, United Kingdom). The structures of OA–Fe3O4 nanoparticles were identified by XRD (Bruker D8, Bruker AXS, Germany).
3. Results and discussion
3.1 Morphology and structures
The transmission electron microscopy (TEM) images depicted in Fig. 2a and b show that the size of the OA–Fe3O4 sample is about 10 nm. Clearly, most of the Fe3O4 nanoparticles are coated with OA. The higher magnification TEM image (Fig. 2c) reveals that the lattice spacing is 0.253 nm, which is consistent with the d value of the (311) planes of Fe3O4. In Fig. 2d, the SEM image of the composite microgel Nms-MAA-20, prepared with a MAA comonomer amount of 20 μL, shows that the sample is a spherical microgel with a diameter of about 400 nm. To further investigate whether Fe3O4 nanoparticles were successfully coated with the thermosensitive polymer, the composite microgel was observed via TEM (Fig. 2e and f). It was found that the composite microgel had agglomerations with a diameter of about 400 nm, in which the black center denotes the OA–Fe3O4 core, whereas the gray layer around the center is the copolymer shell. During the reaction, the existence of electrostatic repulsion between oleic acid and COO− led to unsatisfactory coating of the microgel on the OA–Fe3O4 nanoparticles. Through ultrasonication, this situation was obviously improved. As shown in Fig. 2f, Fe3O4 nanoparticles are encapsulated into microgel with the overall size of about 180 nm and the thickness of about 80 nm. Therefore, we conclude that the Fe3O4 nanoparticles were successfully coated with the temperature-responsive polymer.
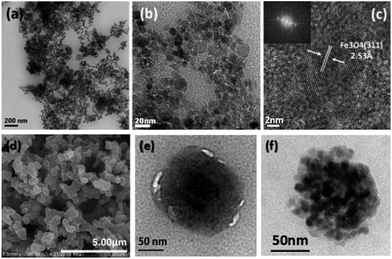 |
| Fig. 2 (a)–(c) TEM images of OA–Fe3O4, (d) SEM images of Nms, and (e) and (f) TEM images of Nms with 0.5 mg and 5 mg OA–Fe3O4 nanoparticles, respectively. | |
The samples with different amounts of Fe3O4 nanoparticles (0.5 mg and 5 mg) are displayed in Fig. 2e and f, respectively, in which obvious differences can be observed. However, it was found that the Fe3O4 fraction around the composite microgel (Fig. 2f) is relative in the sample with less Fe3O4 nanoparticles. This suggests that only a few monomers were involved in the polymerization reaction, such that a lower content of Fe3O4 nanoparticles was in the microgel and the 5 mg Fe3O4 sample reached saturation within the reaction system solution. To further increase the content of Fe3O4 nanoparticles, the electrostatic interaction between inorganic nanoparticles and polymer microgel could be used in the process of the ultrasound irradiation method25 since the prepared OA–Fe3O4, initiator APS, and comonomer are charged. Thus, the second capsulation could improve the amount of Fe3O4 nanoparticles in the composite microgel by ultrasound treatment. As shown in Fig. 3a and b, the composite microgel containing 0.5 mg of Fe3O4 nanoparticles was observed by SEM before and after the second capsulation. The results indicate that they have a similar morphology; however, we were unable to determine whether the Fe3O4 nanoparticles were absorbed into the composite microgel. By investigating the photothermal efficiency of the second capsulation composite microgel, it was observed that the temperature of the composite microgel after the second capsulation exhibited a pronounced increase under an 808 nm laser irradiation, and the highest elevated temperature was about 42 °C, which is higher than that in the previous capsulation.
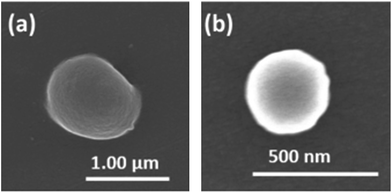 |
| Fig. 3 SEM of Nms (a) before and (b) after the second capsulation. | |
3.2 Characterization of Fe3O4 and OA–Fe3O4 nanoparticles
3.2.1 VSM. The magnetic properties of the OA–Fe3O4 nanoparticles were investigated using a SQUID magnetometer. The Fe3O4 and OA–Fe3O4 hysteresis loops and magnetic optical images are depicted in Fig. 4. This sample exhibited the superparamagnetic behavior of Fe3O4 (Ms = 70 emu g−1) and OA–Fe3O4 (Ms = 60 emu g−1) due to its near zero coercivity and remanence, which indicate that OA–Fe3O4 does not change the magnetism compared with Fe3O4, and these nanoparticles would be an important candidate for biomedical applications.
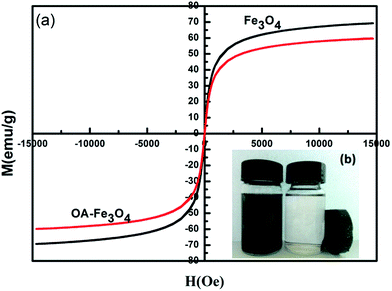 |
| Fig. 4 (a) Magnetic hysteresis loops of OA–Fe3O4 and Fe3O4 and (b) magnetic optical image of OA–Fe3O4 and Fe3O4 nanoparticles. | |
3.2.2 XRD. To further ensure the structure of the OA–Fe3O4 nanoparticles, its XRD patterns (Fig. 5) were obtained and they matched well with the XRD patterns of the standard spinel type of Fe3O4 (JCPDS card file no: 19-0629).2 The characteristic peaks of Fe3O4 crystals marked by their indices (220), (311), (400), (422), (440), and (533) can be observed in the patterns of the samples. Compared with Fe3O4, the peak intensity of OA–Fe3O4 remains unchanged, suggesting that the diffraction peaks of the two samples are identical. Furthermore, the result shows that the diffraction peaks of the two samples are all certainly broadened, and the prepared nanoparticles are smaller in size. However, the average particle sizes are corrected from those obtained via TEM as approximately 127 nm as a result of the higher crystallinity of the prepared OA–Fe3O4 nanoparticles.
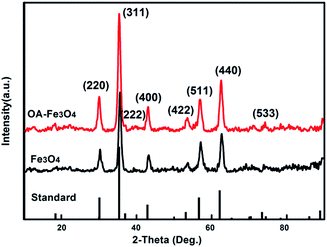 |
| Fig. 5 XRD patterns of OA–Fe3O4 and Fe3O4 nanoparticles. | |
3.3 Characterization of Fe3O4/p(NIPAM-co-MAA)
The structures of the as-obtained composite microgel Nms and p(NIPAM-co-MAA) microgel were further characterized by Fourier transform infrared (FTIR) spectroscopy (Fig. 6a). The peak at 570 cm−1 is characteristic of the stretching vibrations of Fe–O. The absorption bands at 2976, 2937, and 2877 cm−1 correspond to the symmetric and asymmetric stretching vibrations of –CH3 and –CH2, respectively. In the infrared spectrum of OA–Fe3O4, it can be observed that COO− was chemically adsorbed on the surface of Fe3O4, and the stretching vibration peak was shifted to 1631 cm−1 due to the coupling of Fe3+. Compared with the p(NIPAM-co-MAA) and Nms curves, the peaks at 369 cm−1 and 1386 cm−1 are the bending vibrations of –C (CH3)2, 1637 cm−1 and 1548 cm−1 are the characteristic absorption peaks of amide I and amide II, respectively, and the broad absorption peak near 3478 cm−1 occurred due to the overlapping of –OH and N–H bonds. Specifically, the dynamic light scattering (DLS) size of the Nms prepared with 20 μL MAA was 318.3 nm at 25 °C (Fig. 6b), which is comparable to that reported in earlier studies,24 and good dispersion could be observed from the figure without larger or smaller particles. Underwent reversible shrinks and swells, well cycles of these Nms by switching the temperature between 25 °C and 50 °C (Fig. 6c) were achieved, suggesting their applicability in long-term stable thermosensitive drug release. To adjust its response to temperature and pH in cancer-tumor, the hydrophilic and acidic monomer MAA was introduced into the PNIPAM-based microgel. Fig. 6d depicts the LCST measured using a turbidity curve, which shows that the LCST of Nms with the comonomer MAA amounts of 10 μL, 20 μL, and 30 μL was 35.4 °C, 37.2 °C, and 38.3 °C, respectively. The LCST of the composite microgel with MAA was higher than that of pure PNIPAM (which was about 32 °C). This result indicates that the MAA monomer could increase the LCST of the composite microgel. TG measurement could roughly determine the content of Fe3O4 nanoparticles in the synthesized microgel. The TG/DTG curves of Nms are shown in Fig. 6e. The DTG derivative curves from room temperature to 690.8 °C show two distinct transitions for the composite microgel, and the TG curves indicate three weight loss processes (Nms lost ∼2% residual water from room temperature to 307.5 °C; ∼93.8% due to the decomposition of organic components from 310.5 °C to 502.6 °C, which suggests the existence of a small amount of Fe3O4 nanoparticles; and no remarkable weight loss in the range of 502.6–700 °C, which suggests the remaining Fe3O4 nanoparticles of about 0.36%)
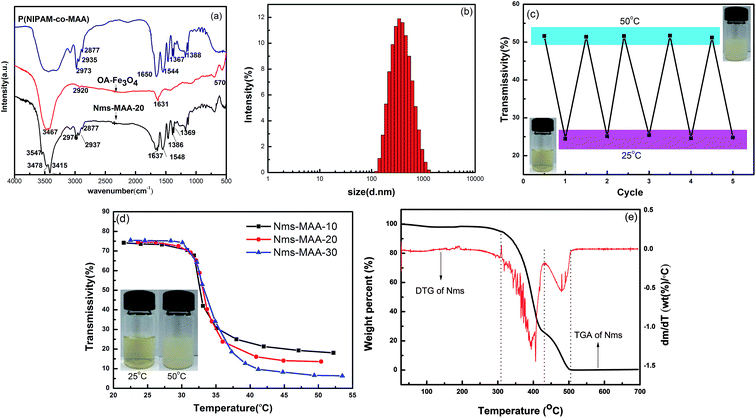 |
| Fig. 6 (a) FT-IR spectra of p(NIPAM-co-MAA), OA–Fe3O4, and Nms, (b) DLS size and distribution of Nms, (c) transmissivity change of Nms by switching the temperature between 25 and 50 °C, (d) LCST of Nms by testing the change in transmissivity with temperature, and (e) TGA and DTG of Nms. | |
3.4 Photothermal conversion of OA–Fe3O4 and Fe3O4/p(NIPAM-co-MAA)
The OA–Fe3O4 nanoparticles within an aqueous suspension show a broad and continuous absorption spectrum19 and the NIR absorption intensity increased with the concentration of OA–Fe3O4 nanoparticles (Fig. 7a). The photothermal conversion effect was also initially studied on dry OA–Fe3O4 nanoparticle powders, which were exposed to an 808 nm laser irradiation at a relatively low power density (1 W cm−2) (Fig. 7b). The heating curves of these solutions were similar to those of OA–Fe3O4 nanoparticles and showed rapid heating and large temperature increments depending on the concentrations. The photothermal effects of the Fe3O4/p(NIPAM-co-MAA) Nms solution with different amounts of OA–Fe3O4 nanoparticles at 0.5, 3.0, and 5.0 mg were exposed to an 808 nm NIR laser irradiation at the power density of 1.0 W cm−2 for 5 min (Fig. 8). In contrast to water, Nms showed a concentration-dependent temperature increase. We also clearly observed similar temperature increase processes of Nms at different points of times, which could be directly attributed to the efficient absorption of the 808 nm NIR laser irradiation by Nms. The photothermal conversion efficiency could reach up to ∼30%. After this, the photothermal efficiency of the composite microgel after second capsulation was found to increase under the 808 nm laser irradiation (Fig. 9a), and the highest elevated temperature was about 42 °C, which is higher than that in the previous capsulation. Moreover, the composite microgels with 0.5 mg and 5.0 mg Fe3O4 nanoparticles, obtained through second capsulation, were irradiated under the 808 nm laser irradiation (Fig. 9b). The composite microgel with 5.0 mg Fe3O4 nanoparticles obtained through second capsulation had significantly higher photothermal efficiency as compared to the former, and the temperature could reach up to 45.8 °C within 5 min under an irradiation power density of 1.0 W cm−2. Therefore, all our outcomes reveal that the optimized Fe3O4/p(NIPAM-co-MAA) Nms displays excellent photothermal conversion efficiency, which is favorable for controlled photo-thermosensitive drug release.
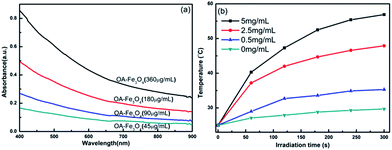 |
| Fig. 7 (a) UV-vis absorption spectra of OA–Fe3O4 nanoparticles at different concentrations and (b) temperature evaluation of OA–Fe3O4 nanoparticles at different concentrations under 808 nm laser irradiation for 5 min. | |
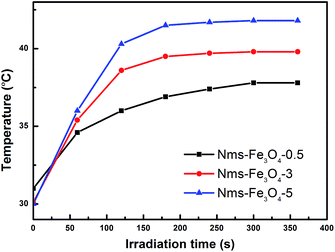 |
| Fig. 8 Temperature evaluation of Nms with different amounts of OA–Fe3O4 under an 808 nm laser irradiation for 5 min. | |
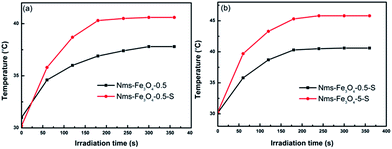 |
| Fig. 9 (a) Temperature evaluation of Nms at an OA–Fe3O4 content of 0.5 mg before and after the second capsulation under an 808 nm laser irradiation for 5 min and (b) temperature evaluation of Nms with different amounts of OA–Fe3O4 under an 808 nm laser irradiation for 5 min by second capsulation. | |
3.5 NIR photothermally controlled drug release
Encouraged by the excellent photothermal properties of Nms, DOX (a drug model) was loaded into Nms via electrostatic interaction and hydrogen bonds. The quantitative analysis of DOX as a result of drug release was studied using different concentrations of DOX standard solutions, and the transmittance of a UV-vis spectrophotometer was used at the wavenumber of 480 nm. The different concentrations of DOX in aqueous solutions following the Lambert–Beer law are presented in Fig. 10a, and the R2 value of the fitted curve is 0.997. Then, the cumulative release rate was investigated. Details of the analysis include incubation of the DOX-loaded composite microgels in a PBS solution at pH 7.4 and keeping the samples at 30 °C for 1 h before the release. The DOX concentration was measured every 30 min and the 808 nm laser irradiation was turned on and the samples were irradiated at the power density of 1.0 W cm−2. The sample was analyzed after 10 minutes, and the abovementioned steps were repeated until the DOX concentration was not significantly increased. The cumulative release rate formula (equation) could be represented as follows:
where v1 is the total volume of the PBS solution (200 mL), ci is the concentration of the drug in the PBS solution, v2 is the volume of the sample (3 mL), and m is the total quality of the composite microgel. The calculated total amount of DOX loading within 24 mg composite microgel was 3.7 mg. As shown in Fig. 10b, the slow drug release of the composite microgels at 30 °C was investigated under dark conditions and an 808 nm laser irradiation. The cumulative release rate of the drug-loaded microgel under an 808 nm laser irradiation is faster than that without laser irradiation. The two curves indicate that DOX was quickly released in the first 5 h, then gradually released, and finally there was no obvious drug release after 16 h (within the PBS solution at the pH value of 7.4). The drug release of the composite microgel was tested under an 808 nm laser, as shown in Fig. 10b and c. The composite microgel containing Fe3O4 nanoparticles without the irradiation of the 808 nm laser only released about 40% of the drug, which is far lower than that by the composite microgel irradiated with the 808 nm laser (Fig. 10b). In the Fe3O4/p(NIPAM-co-MAA) microgel, Fe3O4 nanoparticles works as the photothermal agent and could transform light into thermal energy to increase the temperature of the composite microgel upon laser irradiation. However, the microgel without Fe3O4 nanoparticles could not be affected and changed by 808 nm laser irradiation, and thus the tiny release amount of drug, as shown in Fig. 10c, is mostly induced from the diffusion effect of the laser. As shown in Fig. 10d, the cumulative release curve of the drug indicates that the drug release rate under the 808 nm laser irradiation is extremely faster than that in the absence of the laser. Moreover, the final cumulative release rate was about 25%, which is higher than the cumulative release rate without 808 nm laser irradiation. Thus, we conclude that the prepared composite microgel could absorb NIR light and convert it into heat energy, and the promotion of the drug loaded and released rate are significantly increased under laser irradiation. The increase in the final cumulative release rate originated from the repeated heating and dropping of the composite microgel structure under intermittent 808 nm laser irradiation, which led to the composite microgel shrinking and swelling, respectively. The high cumulative release rate originated from the shrinking and swelling process of the microgel, which were driven by the intermittent irradiation of the NIR laser.
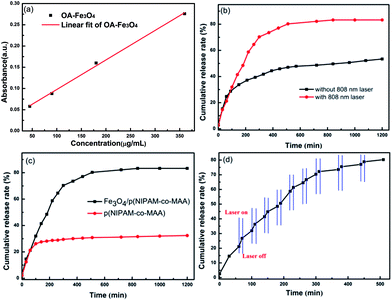 |
| Fig. 10 (a) Standard curve of DOX, (b) DOX cumulative release with time under dark conditions and an 808 nm laser irradiation, (c) DOX cumulative release of p(NIPAM-co-MAA) and Fe3O4/p(NIPAM-co-MAA) with time under an 808 nm laser irradiation, and (d) DOX cumulative release with time by on/off 808 nm laser irradiation. | |
4. Conclusion
In summary, an Fe3O4/p(NIPAM-co-MAA) Nms composite microgel was prepared by the emulsion polymerization of NIPAM and MAA in the presence of OA–Fe3O4 nanoparticles. Nms exhibits both photothermal effects and thermo/PH field sensitivities, and its drug release rate could be increased by 25%. Further, after adjusting the amount of MAA, the LCST of the composite microgel could be increased to 37.2 °C and the temperature of Nms could be increased to 45.8 °C under an 808 nm laser irradiation by the second capsulation method. Due to its good biocompatibility and degradability, Fe3O4 nanoparticles show great promise in meeting the clinical requirements of mild irradiation and low dosage. Moreover, the magnetic properties of Fe3O4 nanoparticles could be used to separate proteins and enzymes as well as in nuclear magnetic resonance imaging.
Acknowledgements
The authors are grateful for the Excellent Academic Leaders Foundation of Harbin, China (No. 2014RFXXJ017), and the Open Project of State Key Laboratory of Urban Water Resource and Environment, Harbin Institute of Technology (No. QA201610-02).
References
- S. S. Aravind, J. Costa, M. Pereira, V. Mugweru, A. Ramanujachary and T. D. Vaden, Int. J. Hydrogen Energy, 2014, 39, 11528–11536 CrossRef CAS.
- H. Wu, G. P. Liu, Y. Zhuang, D. Wu, H. Zhang, H. Yang and S. Yang, Biomaterials, 2011, 32, 4867–4876 CrossRef CAS PubMed.
- J. K. Oh, R. Drumright, D. J. Siegwart and P. Matyjaszewski, Polym. Sci., 2008, 33, 448–477 CAS.
- A. S. Hoffman, J. Controlled Release, 1987, 6, 297–305 CrossRef CAS.
- R. Langer and J. P. Vacanti, Science, 1993, 260, 920–926 CAS.
- R. Langer, Science, 2001, 293, 58–59 CrossRef CAS PubMed.
- D. Crespy and R. M. Rossi, Polym. Int., 2007, 56, 1461–1468 CrossRef CAS.
- M. Ballauff and Y. Lu, Polymer, 2007, 48, 1815–1823 CrossRef CAS.
- M. Quesada-Pérez, J. A. Maroto-Centeno, J. Forcada and R. Hidalgo-Alvarez, Soft Matter, 2011, 7, 10536 RSC.
- H. Kawaguchi, Polym. Int., 2014, 63, 925–932 CrossRef CAS.
- S. B. Abel, S. B. Abel, M. A. Molina, C. R. Rivarola, M. J. Kogan and Barbero, Nanotechnology, 2014, 25, 495602 CrossRef PubMed.
- H. Wan, Y. Zhang, Z. Liu, G. Xu, G. Huang, Y. Ji and H. zou, Nanoscale, 2014, 6, 8743–8753 RSC.
- S. S. Chou, B. Kaehr, J. Kim, B. M. Foley, M. De, P. E. Hopkins and V. P. Dravid, Angew. Chem., Int. Ed., 2013, 52, 4160–4170 CrossRef CAS PubMed.
- Z. Zhang, J. Wang, X. R. Nie, T. Wen, Y. Ji, X. Wu and R. Chen, J. Am. Chem. Soc., 2014, 7317–7326 CrossRef CAS PubMed.
- G. S. Song, F. Jiang, R. Hu, W. P. Li and J. Hu, ACS Appl. Mater. Interfaces, 2014, 6, 3915–3922 CAS.
- Q. Tian, F. Z. Jiang, Q. Liu, Z. Chen, M. Zhu and J. Hu, ACS Nano, 2011, 5, 9761–9771 CrossRef CAS PubMed.
- G. Song, Q. Wang, Y. Wang, G. N. Lv, R. Zou and J. Hu, Adv. Funct. Mater., 2013, 23, 4281–4292 CrossRef CAS.
- Q. Liu, C. Sun, Q. He, D. Liu, A. Khalil, T. Xiang and L. Song, Chem. Commun., 2015, 51, 10054–10057 RSC.
- M. Chu, Y. Shao, J. Peng, X. Dai, H. Li, Q. Wu and D. Shi, Biomaterials, 2013, 34, 4078–4088 CrossRef CAS PubMed.
- A. Bumb, M. W. Brechbiel, P. L. Choyke, L. Fugger and A. Eggeman, Nanotechnology, 2008, 19, 335601 CrossRef CAS PubMed.
- J. Park, K. An, Y. H. Wang, J. G. Park, H. J. Noh, J. Y. Kim and T. Hyeon, Nat. Mater., 2004, 3, 891–895 CrossRef CAS PubMed.
- S. Huang, J. J. Liu, Q. He, H. C. Chen, S. Xu and L. Wang, Nano Res., 2015, 8, 4038–4047 CrossRef CAS.
- X. L. Lin, D. Y. Tang, Z. Q. Yu and Q. Feng, J. Mater. Chem. B, 2014, 2, 651–658 RSC.
- Y. S. Kang, S. Risbud, J. F. Rabolt and P. Stroeve, Chem. Mater., 1996, 8, 2209–2215 CrossRef CAS.
- J. Chen, C. Burger, C. V. Krishnan and B. J. Chu, J. Am. Chem. Soc., 2005, 127, 4140–4141 CrossRef PubMed.
|
This journal is © The Royal Society of Chemistry 2017 |
Click here to see how this site uses Cookies. View our privacy policy here.