DOI:
10.1039/C7RA01498H
(Paper)
RSC Adv., 2017,
7, 26559-26565
Sensing of hydrogen peroxide and glucose in human serum via quenching fluorescence of biomolecule-stabilized Au nanoclusters assisted by the Fenton reaction†
Received
6th February 2017
, Accepted 10th May 2017
First published on 17th May 2017
Abstract
Fe2+ can act as a catalyst to disproportionate hydrogen peroxide (H2O2) to produce extremely reactive hydroxyl radicals (˙OH) through the so-called Fenton reaction. Combining this reaction with the prominent sensitive nature of gold nanoclusters (Au NCs), we present herein a simple strategy of sensitive and rapid detection of H2O2. Compared with H2O2, the produced hydroxyl radical exhibits a much stronger oxidizing ability, and therefore could lead to a more efficient oxidation of the Au NCs and an improved sensitivity and oxidation rate. The results indicate that the detection limit for the determination of H2O2 was 0.2 μM (signal/noise = 3) and the linear range was 0.4–12 μM. Furthermore, in combination with the specific catalytic effect of glucose oxidase, the present sensing strategy can be successfully expanded to detect glucose in blood. The preliminary results are in good agreement with those provided by the hospital, which suggests the generalizability and great potential of the Au NCs/Fenton hybrid system for research and clinical diagnosis of diabetes.
1. Introduction
Glucose, as a major energy source for living systems and metabolic intermediates, is closely associated with human health.1–4 High levels of glucose in blood can lead to diabetes, a very common disease that seriously threatens the health of human beings. For effective management of diabetes and reduction of associated complications, frequent monitoring and tight control of blood glucose levels are highly required. To date, various approaches to glucose sensing in diabetes have been actively explored. Among these methods, the optical spectrum analysis of hydrogen peroxide (H2O2) produced from glucose oxidase catalysis of glucose has proved to be a popular and effective way.5–10 As one of the most reactive oxygen species, H2O2 is not only associated with many physiological processes but also is an important mediator in food, pharmaceutical, clinical, industrial and environmental analyses.11–13 Thus, the determination of hydrogen peroxide has also been an important task in the field of bio-imaging, healthcare and anti-terrorism. Continuing efforts in detecting H2O2 have been focused on different strategies including infrared/Raman spectroscopy, chromatography and electrochemical methods.14–16 Although these methods made great contributions in H2O2 and glucose detection, they often suffer from drawbacks, such as the requirement of expensive bulky equipment, long analysis time, complicate procedures and high detection limit. In this regard, it is still in great need of an inexpensive platform for reliable and rapid detection of H2O2.
H2O2, with an electrochemical potential of 1.77v, can serve as an oxidizing agent. This property has been well studied and employed to control particle size and shape in the synthesis of nanomaterials.17–20 Most importantly, the prominent oxidizing nature of H2O2 has also been highlighted for successful development of effective H2O2 sensors. For example, oxidation induced dissolution of silver/gold nanoparticles (Ag NPs/Au NPs) in the presence of H2O2 has been demonstrated in several kinetic and mechanistic studies.21–24 Notably, these oxidation processes often accompanied by a visible color and surface plasmon resonance (SPR) changes due to the unique size- and shape-dependent SPR properties of Ag NPs/Au NPs. Although these methods enable naked-eye detection of H2O2, the stabilization of Ag NPs/Au NPs is a challenging topic in practical application due to their easy oxidation (for Ag NPs) and aggregation. To address this issue, fluorescent quantum dots (QDs) have attracted wide attention.25–28 Comparing with the colorimetric analysis, the QDs-based fluorescent detection method exhibits more advantages, such as high sensitivity and selectivity. However, the heavy metal ion-containing QDs commonly suffer from intrinsic limitations, such as complicate modification, potential toxicity, intrinsic blinking and chemical instability.29,30
In order to avoid these disadvantages, we focused on gold-based nanoclusters (Au NCs). Compared to silver/gold nanoparticles and QDs, Au NCs, consisting of several to tens of gold atoms, have obvious superiority in sensing applications since they are non-toxic, high fluorescent, and have improved biocompatibility and stability. More importantly, the fluorescence of the Au NCs is highly sensitive toward sizes and changes of protecting agents.31,32 To date, various types of H2O2 sensors have been developed based on AuNCs. For example, Zhang has reported the first photoelectrochemical H2O2 sensor based on mercaptoundecanoic acid protected Au NCs (MUA-Au NCs), but it suffers from some darwbacks including limited sensitivity and poor linearity.33 Molaabasi has used haemoglobin capped Au NCs (Hb-Au NCs) for the detection of H2O2 by taking advantages of the sensitive nature of Hb-Au NCs and the oxidizing property of H2O2.34 This method offered an improved sensitivity and linearity, however, it needed long analysis time. Besides, the blue emission of the Hb-Au NCs may be interfered by the background autofluorescence of the serum samples. Recently, horseradish peroxidase (HRP) functioned fluorescent Au NCs that possess dual functions including catalysis ability and fluorescence have been designed for H2O2 detection.35 In this case, H2O2 can be catalyzed by the HRP shell, leading to a significant quenching of the fluorescent gold core. This method offers a good sensitivity and fast response, but it pose a great challenge when using in complex system such as serum, since the activity of HRP can be easily affected. By combining the prominent sensitive nature of the Au NCs with Fenton reaction, we propose herein a rapid and effective strategy for H2O2 and glucose detection. In the present case, Fe2+ served as a catalyst to disproportionate H2O2 to produce hydroxyl radical (˙OH) through the so-called Fenton reaction.36–38 The produced radical species are extremely reactive which can oxidize not only the thiol group of the protecting agent, but also the Au atoms in Au NCs. Therefore, sensitive detection of H2O2 can be achieved. Moreover, since the oxidizing ability of the produced radical species is much stronger than H2O2, the response rate could thus be significantly improved.
Compared with the known optical H2O2 detection strategies, our proposed method possesses some remarkable features. First, the Au NCs were fabricated with a simple, environment friendly method, thus minimizing cost and avoiding the use of toxic ions or organic reagents. Second, the fluorescence behavior of Au NCs are highly size- and surface protecting agent-dependent, hence making the present sensing strategy theoretically simple and low technical demands. In particular, the red emission of the Au NCs can decrease the interference from the background autofluorescence of the serum samples effectively. Third, the oxidizing ability of ˙OH produced from the Fenton reaction is much stronger than that of H2O2, and therefore affording a better sensitivity and fast response. Forth, only 100 to 200 μL of serum could well meet the detection requirement owing to the high sensitivity. The whole processes could be accomplished within minutes. We believe such simple and low-cost H2O2 and glucose sensor has great potential in applications of point-of-care diagnostics.
2. Experimental
2.1 Reagents and apparatus
All reagents were of analytical grade and used without further purification. Ferrous sulfate (FeSO4·7H2O), HAuCl4·3H2O and glucose oxidase (EC 1.1.3.4) were bought from Sigma-Aldrich. Bull Serum Albumin (BSA), hydrogen peroxide (H2O2, 30 wt%), sodium hydroxide (NaOH), glucose, fructose, lactose, sucrose, maltose, mannose and other salts were purchased from Aladdin Chemical Company (Shanghai, China). Water was purified through a millipore system.
The fluorescence intensity spectra were recorded on an F-4600 fluorescence spectrometer (Hitachi Co., Japan). XPS was performed using a VGESCALAB MKII spectrometer. The XPSPEAK software was used to deconvolute the narrow-scan XPS spectra of the Au 4f of the Au NCs, using adventitious carbon to calibrate the C1S binding energy (284.5 eV). A PHS-3C pH (Shanghai Analytical Instrument Factory, Shanghai, China) meter was used to adjust pH values.
2.2 Fluorescence detection of H2O2 and glucose
The BSA-stabilized Au NCs were synthesized according to a method described in a previous report.39 Briefly, HAuCl4 solution (5 mL, 10 mM) was added to BSA solution (5 mL, 50 mg mL−1) under vigorous stirring at 37 °C. Two minutes later, NaOH solution (0.5 mL, 1 M) was introduced and the reaction was allowed to proceed under vigorous stirring for 12 h at 37 °C. A typical H2O2 detection procedure by using the as-prepared Au NCs was conducted as follows: 50 μL of as-prepared Au NCs solution were diluted by 950 μL water, and then HCl was added to adjust the pH value. 8 μL Fe2+ (40 mM) were then added as catalysis to induce the production of radical species. Subsequently, 2 μL of H2O2 solution with different concentrations was added to the above mixture and was incubated at room temperature for 8 min. Finally, the fluorescence emission spectra were collected.
For glucose detection, different concentrations of glucose (100 μL) were mixed with 400 μL of 0.5 mg mL−1 GOx formulated with HAc-NaAc (pH 5.1) and incubated at 37 °C for an hour. Then 10 μL of the mixture was added to the sensing system and incubate for 8 min. The fluorescence intensities were recorded in the wavelength range of 550–800 nm.
The selectivity of the sensing system toward glucose was evaluated by using sucrose, mannose, cellobiose, lactose, fructose, maltose, folic acid and typisn. Moreover, the selectivity of the sensing system toward common cations (e.g., K+, Ca2+, Mg2+, Zn2+ and Cu2+) was also evaluated by using their corresponding nitrates. In the case of Cu2+, polyethyleneimine (PEI, Mw = 25
000) was used to chelate and separate Cu2+.
The procedure for glucose detection in serum was as follows: human serum samples were obtained from healthy volunteers treated in local hospital. The samples were centrifuged at 12
000 rpm for 10 min to remove the possible interference of proteins in human serum. 100 μL of serum samples and 400 μL of 0.5 mg mL−1 GOx were incubated at 37 °C for an hour. Then 10 μL of the above mixture was added to the Au NCs sensing system as mentioned above and incubate for 8 min. Finally, the fluorescence intensity was collected at room temperature. All fluorescence detections were performed under the same conditions.
2.3 Live subject statement
The author state that the blood related experiments were performed in strict accordance with the WHO guidelines on blood drawing (WHO Publication ISBN-13: 978-92-4-159922-1, 2010) and was approved by Jiangsu Normal University. The authors also state that informed consent was obtained for any experimentation with human subjects and the Jiangsu Normal University is committed to the protection and safety of human subjects involved in research.
3. Results and discussion
3.1 Mechanism for fluorescence quenching of Au NCs
Bovine serum albumin (BSA) stabilized Au NCs were prepared according to a method described previously.39 Transmission electron microscopy (TEM) images reveal that the as-prepared Au NCs have an average diameter of ca. 1.2 nm (Fig. S1†). As shown in Fig. 1A, the initial solution of the as-prepared Au NCs exhibited a bright red fluorescence with the maximum emission spectra centered at 660 nm upon excitation at 529 nm. It is well known that H2O2 can generate extremely oxidative hydroxyl radicals in the presence of Fe2+, so called Fenton reaction, which has been widely applied in wastewater treatment.36 Herein, we take advantage of Fenton reaction to accelerate fluorescence quenching of Au NCs in the presence of H2O2 to sensitive and selective detect H2O2 and glucose (Scheme 1). After 20 μM H2O2 was added to the Au NCs–Fe2+-HCl system, the corresponding emission intensity of the sensing system was completely quenched (Fig. 1A). Notably, this quenching efficiency was much larger than that of H2O2. Dong et al. reported that 0.5 mM H2O2 could induce 45% quenching of the fluorescence of Au NCs in 30 min.40 Impressively, our experiments showed that 10 μM H2O2 could reduce 76% of the fluorescence of Au NCs within 8 min. This increased quenching efficiency was reasonable considering the fact that the oxidizing ability of ˙OH produced from Fenton reaction is much stronger than that of H2O2. Therefore, ˙OH could lead to a more efficient quenching of the Au NCs and provide an improved sensitivity and oxidation rate. To understand the origin of the outstanding sensing performance of the present sensing system toward H2O2, possible interactions between Au NCs and H2O2 in the presence of Fe2+ were further investigated by X-ray photoelectron spectroscopy (XPS) analysis. For the as-prepared Au NCs, the Au 4f7/2 peak in the XPS spectrum at 83.7 eV is in good agreement with previous literature value.41 Whereas, this peak shifted to a higher binding energy (84.7 eV) upon the addition of H2O2 (Fig. S2†), which indicated the oxidation of the Au atoms in Au NCs.42 Besides, previous studies have confirmed that Au–S bond can be degraded in the presence of H2O2 as an oxidant to form disulfides and sulfonates which can be easily removed from the Au surface.40,43 Considering the above facts and the stronger oxidizing ability of ˙OH, it is reasonable to believe that oxidation of the Au–S bond may also occur during the H2O2 recognition process. To verify this assumption, the absorption spectra of the Au NCs in the presence and absence of H2O2 were recorded, respectively. As shown in Fig. S3,† the original Au NCs exhibited a characteristic absorbance at 278 nm, which mainly originates from the aromatic residues and disulfide bonds in BSA, the stabilizing protein in Au NCs.44 However, this peak shifted distinctly in the presence of 10 μM H2O2, which could be assigned to the oxidation of the composition of BSA as suggested by previous reports.40,45 The above results clearly indicated that both the protecting BSA molecule and the Au atoms were oxidized during the course of H2O2 response. Thus, it is reasonable to conclude that the effective fluorescence quenching of the sensing system in the presence of H2O2 could ascribe to the oxidation-induced destruction of the Au NCs.
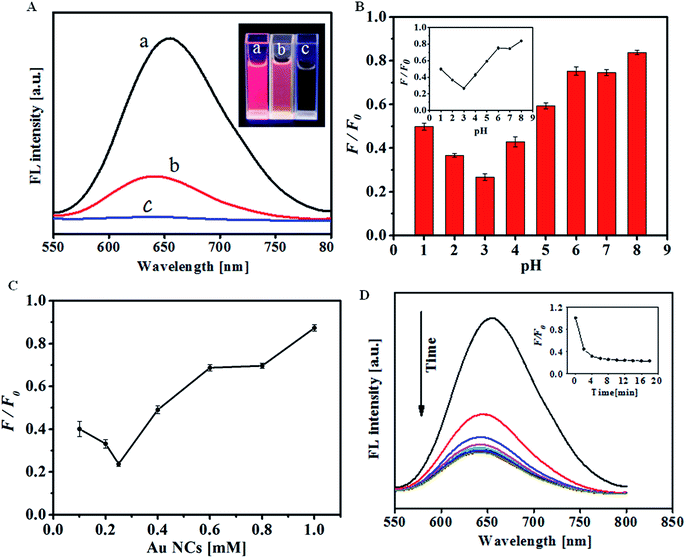 |
| Fig. 1 (A) Fluorescence response of the Au NCs in the absence (a) and presence of 10 μM H2O2 (b) and 20 μM H2O2 (c) respectively; (B) fluorescence response of the Au NCs toward 10 μM H2O2 at different pH values; (C) relationship between F/F0 and the concentration of Au NCs in the presence of 10 μM H2O2; (D) time-dependent fluorescence response of the Au NCs to10 μM H2O2 (F0 and F are the fluorescence intensity of the H2O2 at 660 nm in the absence and presence of H2O2, respectively). | |
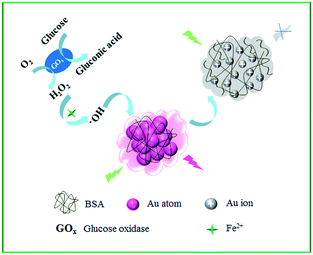 |
| Scheme 1 Principle representation of the developed method for H2O2 and glucose sensing. | |
3.2 Optimization of the sensing parameters and detection of H2O2
It is known that the catalytic decomposition of H2O2 by Fe2+ depends on the pH value of the reaction media. In order to achieve sensitive detection of H2O2 and glucose, effect of the pH value on the fluorescence response of H2O2 was studied and optimized. According to previous reports, the Fenton reaction is more favored in acidic medium36 and thus, pH value higher than 8.0 was not considered. Fig. 1B shows the quenching efficiency as a function of pH value. In the studied pH range of 1.0–8.0, the quenching efficiency of the sensing system in the presence of H2O2 was gradually increased with increasing pH from 1 to 3, reached the maximum at pH 3.0, and then gradually decreased at higher pH (4–8). Based on the above results, pH 3.0 was chosen for further determination assays. Furthermore, the quenching efficiency of H2O2 in the presence of Fe2+ as a function of the concentration of Au NCs was assessed. It was found that the fluorescence quenching is more efficient at lower concentration of the fluorescence probe (Fig. 1C) in the presence of a given concentration of H2O2. In the present case, 0.25 mM Au NCs was selected for the subsequent experiments. Moreover, to quantify the response rate of the sensing system, the time dependent fluorescence response of the sensing system to 10 μM H2O2 was monitored at 660 nm with an excitation wavelength of 529 nm (Fig. 1D). The curve shows a rapid decrease of the fluorescence intensity in the first 6 min, and kept almost unchanged after 8 min, which suggests that 8 min is enough for the detection of H2O2.
Under the optimized conditions, the capability of the proposed strategy to sensitively and selectively detect H2O2 was evaluated. Plotting F/F0 (F0 and F refer to the fluorescence intensity of the sensing system in the absence and presence of H2O2, respectively) as a function of H2O2 concentration shows a good linear relationship over the concentration range from 0.4 to 12 μM, which clearly validates the sensing performance of the proposed strategy toward H2O2 (Fig. 2). The limit of the detection for H2O2, at a signal-to-noise ratio of 3, is 0.2 μM, which is lower than some other optical methods (Table S1†). The percent relative standard deviation was 3.32% with five replicate detections of 10 μM H2O2 (Table S2†), which indicated a good reproducibility of the present method.
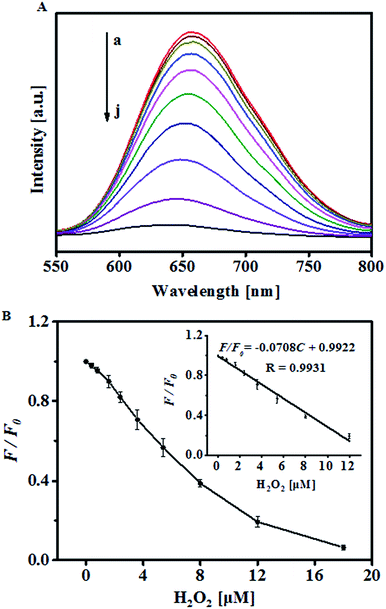 |
| Fig. 2 (A) Fluorescence response of the Au NCs upon addition of various concentrations of H2O2 at a pH value of 3 (H2O2 concentrations from a to j (μM): 0; 0.4; 0.8; 1.6; 2.4; 3.6; 5.4; 8; 12 and 18); (B) the relationship between F/F0 and the concentration of H2O2. The inset is the linear plot in the range of 0 to 12 μM H2O2. | |
3.3 Glucose sensing based on the Au NCs/Fenton and glucose oxidase system
Considering that GOx can catalyze the oxidation of glucose to produce H2O2, the successful sensitive detection of H2O2 was, then, implemented for the analysis of glucose. The process for detecting glucose includes two steps: firstly, H2O2 is generated from the biocatalyzed reaction between varied concentration of glucose and excess amount of GOx. Secondly, a certain volume of the resulted mixture was introduced into the sensing system for 8 min and then the corresponding fluorescence spectra were measured. Fig. 3 displays that the fluorescence intensity of Au NCs reduced gradually with increasing concentration of glucose. Controlled experiments showed that O2, glucose oxidase, and glucose were all essential to the quenching of Au NCs (Fig. 3A inset) since the exclusion of either component would yield no H2O2. After optimizing the experimental parameters, a linear calibration curve is achieved by plotting F/F0 versus glucose concentration in the range of 2–60 μM (Fig. 3B). A detection limit of 0.8 μM was calculated from the equation (signal/noise = 3). To investigate whether this sensing system is specific for glucose detection, the effect of some other carbohydrates and metal ions that commonly present in human blood on the fluorescence of the sensing system were evaluated under the optimum conditions. As demonstrated in Fig. 4, the addition of glucose could induce a significant quenching of the Au NCs fluorescence due to the high substrate specificity of GOx. However, other carbohydrates and metal ions had a negligible effect under the identical conditions. Considering that trypsin, folic acid (FA) and Cu2+ were reported to quench the fluorescence of the Au NCs,46–49 thus, the fluorescence response of our sensing system towards these substance were further examined. As shown in Fig. S4,† no obvious fluorescence change was observed even in the presence of high concentrations of trypsin and FA. This results is explainable since the low pH condition (pH = 3.0) selected in our system can not only weaken the interaction between FA and BSA via protonating both of them but also denature the trypsin. Also, it is noted that Cu2+ with high concentration do have an effect on the fluorescence intensity of the BSA-Au NCs as reported by Lin,48 but such interference could be evaded by adding polyethyleneimine (PEI) as scavengers (Fig. S4c†). Therefore, sensitive and selective detection of glucose can be achieved through our proposed method. Moreover, comparable fluorescence response toward 10 μM H2O2 were performed by using Au NCs stored at 4 °C for one and two weeks, respectively. The negligible change (the percent relative standard deviation is 2.76%) of the fluorescence response as presented in Table S3† indicated the good stability and reproducibility of our sensing system. Due to the above merits, the present method is expected to have a general applicability for detecting glucose in blood.
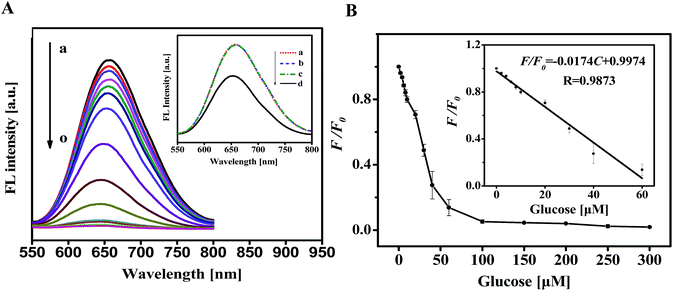 |
| Fig. 3 (A) Fluorescence spectra represent the quenching effect of glucose–GOx system with different glucose concentrations on the fluorescence of Au NCs, glucose concentration from a to o (μM): 0; 2; 4; 6; 8; 10; 20; 30; 40; 60; 100; 150; 200; 250; 300. Inset: fluorescence spectra of Au NCs (a); Au NCs with glucose oxidase (0.4 mg L−1) (b); Au NCs with glucose (20 μM) (c); Au NCs with glucose (20 μM) and glucose oxidase (0.4 mg L−1) (d); (B) the relationship between F/F0 and the concentration of glucose. The inset is the linear plot in the range of 0 to 60 μM glucose. | |
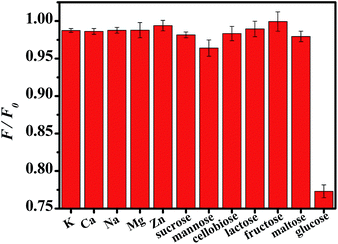 |
| Fig. 4 The relative fluorescence intensity of the Au NCs in the presence of 10 μM various carbohydrates and some metal ions commonly exist in serum. | |
3.4 Determination of glucose in real serum samples
Glucose levels in blood are associated closely with diabetes or hypoglycemia. Thus it is of great importance to accurately monitor blood glucose levels for diagnosis and management of diabetes. Encouraged by the above promising results, the sensing system was further applied to monitor glucose in real serum samples. In this case, the fresh human serum samples were obtained from a local hospital and used as testing samples after simple centrifugation treatment. Taking into consideration of the normal fasting blood glucose (FBG) level in the healthy human blood (3.9–6.1 mmol L−1) as well as the linear range of our method, 100 μL of serum should well meet one-time glucose measurement. In a proof of concept experiment, 100 μL of the testing samples were firstly incubated with 400 μL GOx (0.5 mg mL−1) for an hour, then 10 μL of the resulted mixture was added to the sensing system (1 mL) and the fluorescence spectra were measured. It is worthy to note that the serum samples were 500-fold diluted during the process and therefore, the possible interference from the serum matrix could be significantly reduced. The concentrations of the clinical samples were calculated from the standard curve and the regression equation. Impressively, the glucose concentration obtained by our method is in good agreement with those provided by the hospital (Table 1), which further confirms the general applicability of the proposed method for the analysis of glucose in real physiological clinical samples.
Table 1 Analytical results of glucose in serum samples
Sample no. |
Local hospital (mM) |
Proposed method (mM) |
Relative deviation (%) |
1 |
4.59 |
4.65 |
1.30 |
2 |
4.96 |
4.86 |
−2.02 |
3 |
5.25 |
5.34 |
1.71 |
4 |
12.59 |
12.12 |
−3.73 |
5 |
5.09 |
4.94 |
−2.95 |
4. Conclusion
By combining the Fenton reaction with the prominent sensitive nature of the Au NCs, a rapid, highly sensitive, selective, and cost-efficient sensing approach for H2O2 and glucose detection has been designed. The sensing approach was successfully applied to monitor glucose levels in human serum with satisfactory results. The sensing strategy proposed in this study is very promising in pharmaceutical and clinical detection of H2O2 and glucose due to the advantages of easy fabrication and operation. We anticipate that the designed strategy can also be extended to the detection of various H2O2-involved analytes. This may open up a new avenue in developing low-cost and sensitive method for biological and clinical diagnostics application.
Acknowledgements
The authors would like to acknowledge the financial support from the Natural Science Foundation of China (NSFC 21601072), Natural Science Foundation of Jiangsu Province (BK20150228), Natural Science Foundation of the Higher Education Institutions of Jiangsu Province (16KJA150006) and Priority Academic Program Development of Jiangsu Higher Education Institutions.
References
- M. A. Pleitez, T. Lieblein, A. Bauer, O. Hertzberg, H. von Lilienfeld-Toal and W. Mantele, Anal. Chem., 2013, 85, 1013–1020 CrossRef CAS PubMed.
- Y. Ling, N. Zhang, F. Qu, T. Wen, Z. F. Gao, N. B. Li and H. Q. Luo, Spectrochim. Acta, Part A, 2014, 118, 315–320 CrossRef CAS PubMed.
- Y. Yi, J. Deng, Y. Zhang, H. Li and S. Yao, Chem. Commun., 2013, 49, 612–614 RSC.
- H. B. Wang, H. D. Zhang, Y. Chen, Y. Li and T. Gan, RSC Adv., 2015, 5, 77906–77912 RSC.
- L. Wang, J. Zheng, Y. Li, S. Yang, C. Liu, Y. Xiao, J. Li, Z. Cao and R. Yang, Anal. Chem., 2014, 86, 12348–12354 CrossRef CAS PubMed.
- O. S. Wolfbeis, A. Durkop, M. Wu and Z. H. Lin, Angew. Chem., Int. Ed., 2002, 41, 4495–4498 CrossRef CAS PubMed.
- S. Chen, X. Hai, X. W. Chen and J. H. Wang, Anal. Chem., 2014, 86, 6689–6694 CrossRef CAS PubMed.
- L. P. Lin, X. H. Song, Y. Y. Chen, M. C. Rong, T. T. Zhao, Y. R. Wang, Y. Q. Jiang and X. Chen, Anal. Chim. Acta, 2015, 869, 89–95 CrossRef CAS PubMed.
- L. Z. Hu, Y. L. Yuan, L. Zhang, J. M. Zhao, S. Majeed and G. B. Xu, Anal. Chim. Acta, 2013, 762, 83–86 CrossRef CAS PubMed.
- H. B. Wang, Y. Chen, N. Li and Y. M. Liu, Microchim. Acta, 2017, 184, 515–523 CrossRef CAS.
- D. J. Rossi, C. H. M. Jamieson and I. L. Weissman, Cell, 2008, 132, 681–696 CrossRef CAS PubMed.
- N. E. Sharpless and R. A. Depinho, Nat. Rev. Mol. Cell Biol., 2007, 8, 703–713 CrossRef CAS PubMed.
- D. Srikun, A. E. Albers, C. I. Nam, A. T. Iavaron and C. J. Chang, J. Am. Chem. Soc., 2010, 132, 4455–4465 CrossRef CAS PubMed.
- W. Chen, S. Cai, Q.-Q. Ren, W. Wen and Y.-D. Zhao, Analyst, 2012, 137, 49–58 RSC.
- T. H. Huang, M. E. Garceau and P. Gao, J. Pharm. Biomed. Anal., 2003, 31, 1203–1210 CrossRef CAS PubMed.
- L. L. Qu, Y. Y. Liu, S. H. He, J. Q. Chen, Y. Liang and H. T. Li, Biosens. Bioelectron., 2016, 77, 292–298 CrossRef CAS PubMed.
- W. Ni, X. Kou, Z. Yang and J. Wang, ACS Nano, 2008, 2, 677–686 CrossRef CAS PubMed.
- M. Tsuji, S. Gomi, Y. Maeda, M. Matsunaga, S. Hikino, K. Uto, T. Tsuji and H. Kawazumi, Langmuir, 2012, 28, 8845–8861 CrossRef CAS PubMed.
- Q. Zhang, C. M. Cobley, J. Zeng, L. P. Wen, J. Y. Chen and Y. N. Xia, J. Phys. Chem. C, 2010, 114, 6396–6400 CAS.
- Q. Zhang, N. Li, J. Goebl, Z. Lu and Y. Yin, J. Am. Chem. Soc., 2011, 133, 18931–18939 CrossRef CAS PubMed.
- K. Nitinaivinij, T. Parnklang, C. Thammacharoen, S. Ekgasit and K. Wongravee, Anal. Methods, 2014, 6, 9816–9824 RSC.
- Y. Xia, J. Ye, K. Tan, J. Wang and G. Yang, Anal. Chem., 2013, 85, 6241–6247 CrossRef CAS PubMed.
- X. M. Ma, Z. T. Chen, P. Kannan, Z. Y. Lin, B. Qiu and L. H. Guo, Anal. Chem., 2016, 88, 3227–3234 CrossRef CAS PubMed.
- G. Y. Shan, S. J. Zheng, S. P. Chen, Y. W. Chen and Y. C. Liu, Colloids Surf., B, 2013, 102, 327–330 CrossRef CAS PubMed.
- R. Gill, L. Bahshi, R. Freeman and I. Willner, Angew. Chem., Int. Ed., 2008, 47, 1676–1679 CrossRef CAS PubMed.
- L. H. Cao, J. Ye, L. L. Tong and B. Tang, Chem.–Eur. J., 2008, 14, 9633–9640 CrossRef CAS PubMed.
- M. Hu, J. Tian, H. T. Lu, L. X. Weng and L. H. Wang, Talanta, 2010, 82, 997–1002 CrossRef CAS PubMed.
- J. J. Ge, X. L. Ren, X. Z. Qiu, H. T. Shi, X. W. Meng and F. Q. Tang, J. Mater. Chem. B, 2015, 3, 6385–6390 RSC.
- A. M. Derfus, W. C. W. Chan and S. N. Bhatia, Nano Lett., 2004, 4, 11–18 CrossRef CAS.
- S. F. Lee and M. A. Osborne, J. Am. Chem. Soc., 2007, 129, 8936–8937 CrossRef CAS PubMed.
- P. C. Chen, A. P. Periasamy, S. G. Harroun, W. P. Wu and H. T. Chang, Coord. Chem. Rev., 2016, 320, 129–138 CrossRef.
- L. Y. Chen, C. W. Wang, Z. Q. Yuan and H. T. Chang, Anal. Chem., 2015, 87, 216–229 CrossRef CAS PubMed.
- J. Zhang, L. Tu, S. Zhao, G. Liu, Y. Wang, Y. Wang and Z. Yue, Biosens. Bioelectron., 2015, 67, 296–302 CrossRef CAS PubMed.
- F. Molaabasi, S. Hosseinkhani, A. A. Moosavi-Movahedi and M. Shamsipu, RSC Adv., 2015, 5, 33123–33135 RSC.
- F. Wen, Y. Dong, L. Feng, S. Wang, S. Zhang and X. Zhang, Anal. Chem., 2011, 83, 1193–1196 CrossRef CAS PubMed.
- E. F. Olasehinde, S. Makino, H. Kondo, K. Takeda and H. Sakugawa, Anal. Chim. Acta, 2008, 627, 270–276 CrossRef CAS PubMed.
- H. H. Deng, G. W. Wu, D. He, H. P. Peng, A. L. Liu, X. H. Xia and W. Chen, Analyst, 2015, 140, 7650–7656 RSC.
- L. Zhang, R. P. Liang, S. J. Xiao, J. M. Bai, L. L. Zheng, L. Zhan, X. J. Zhao, J. D. Qiu and C. Z. Huang, Talanta, 2014, 118, 339–347 CrossRef CAS PubMed.
- J. P. Xie, Y. G. Zheng and J. Y. Ying, J. Am. Chem. Soc., 2009, 131, 888–890 CrossRef CAS PubMed.
- L. H. Jin, L. Shang, S. J. Guo, Y. X. Fang, D. Wen, L. Wang, J. Y. Yin and S. J. Dong, Biosens. Bioelectron., 2011, 26, 1965–1969 CrossRef CAS PubMed.
- M. Brust, M. Walker, D. Bethell, D. J. Schiffrin and R. Whyman, J. Chem. Soc., Chem. Commun., 1994, 801–802, 10.1039/c39940000801.
- M. Dasog and R. W. J. Scott, Langmuir, 2007, 23, 3381–3387 CrossRef CAS PubMed.
- C. Vericat, M. E. Vela, G. Benitez, P. Carro and R. C. Salvarezza, Chem. Soc. Rev., 2010, 39, 1805–1834 RSC.
- L. Shang, Y. Z. Wang, J. G. Jiang and S. J. Dong, Langmuir, 2007, 23, 2714–2721 CrossRef CAS PubMed.
- D. Y. Luo, S. W. Smith and B. D. Anderson, J. Pharm. Sci., 2005, 94, 304–316 CrossRef CAS PubMed.
- B. Hemmateenejad, F. Shakerizadeh-shirazi and F. Samari, Sens. Actuators, B, 2014, 199, 42–46 CrossRef CAS.
- L. Hu, S. Han, S. Parveen, Y. Yuan, L. Zhang and G. Xu, Biosens. Bioelectron., 2012, 32, 297–299 CrossRef CAS PubMed.
- Z. Lin, F. Luo, T. Dong, L. Zheng, Y. Wang, Y. Chi and G. Chen, Analyst, 2012, 137, 2394–2399 RSC.
- Y. Tao, Y. Lin, J. Ren and X. Qu, Biosens. Bioelectron., 2013, 42, 41–46 CrossRef CAS PubMed.
Footnote |
† Electronic supplementary information (ESI) available. See DOI: 10.1039/c7ra01498h |
|
This journal is © The Royal Society of Chemistry 2017 |
Click here to see how this site uses Cookies. View our privacy policy here.