DOI:
10.1039/C7RA02136D
(Paper)
RSC Adv., 2017,
7, 18851-18860
Investigation of the dinuclear effect of aluminum complexes in the ring-opening polymerization of ε-caprolactone†
Received
21st February 2017
, Accepted 22nd March 2017
First published on 28th March 2017
Abstract
A series of aluminum (Al) complexes bearing hydrazine-bridging Schiff base and salen ligands were synthesized and investigated as catalysts for the ring-opening polymerization of ε-caprolactone (CL). The introduction of steric bulky groups increases the catalytic activity of the corresponding mononuclear aluminum complex. However, the opposite phenomenon was observed in dinuclear Al complexes bearing salen ligands because the steric repulsion reduced the cooperative activation mechanism in the dinuclear Al system. Among these Al complexes, LN2Bu-Al2Me4 bearing a hydrazine-bridging Schiff base ligand had the highest catalytic activity, approximately 3- to 11-fold higher than that of dinuclear Al complexes bearing salen ligands and mononuclear Al complexes bearing Schiff base ligands. Density functional theory calculations revealed that the mechanism of the coordination of CL to one Al center was initiated by the benzyl alkoxide of another Al center.
1. Introduction
Biodegradable polymers such as poly-ε-caprolactone (PCL) were designed not only to resolve the pollution problem caused by petrochemical plastics, but also as biomaterials for various fields such as tissue engineering,1a–c bone regeneration,1d drug carriers,1e and biomedical application1f–i because of its biodegradability, biocompatibility, and permeability. The use metal complexes2 as catalysts for the ring-opening polymerization (ROP) of ε-caprolactone (CL) leads to efficient synthesis of PCL, and aluminum complexes3,4 are common catalysts for ROP because of their ease of synthesis, the low cost of their precursor materials, and the highly catalytic conversion rate of their CL polymerization. The catalytic activity of aluminum (Al) complexes in cycloester ROP may be improved by ligand modification because the influences of ligands including steric, electronic, and chelating effects, directly change the catalytic activity of metal complexes.3f Recently, dinuclear Al catalysts5,6 were used in cycloesters ROP, and some ROPs,6 shown in Fig. 1, proved that dinuclear Al complexes have higher catalytic activity than do mononuclear Al complexes.
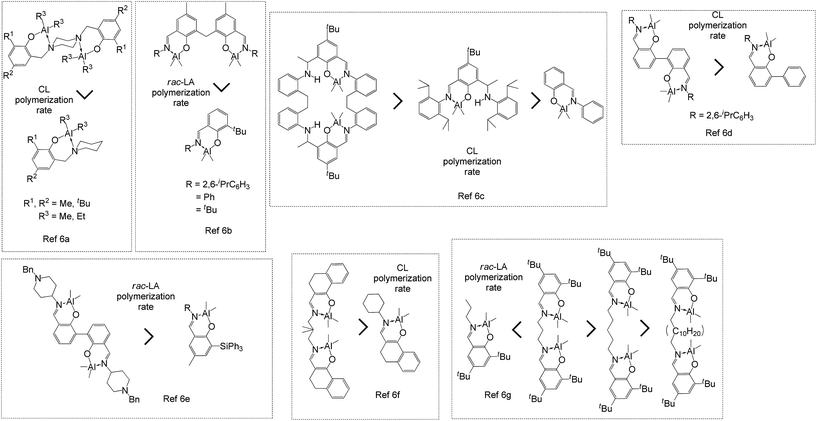 |
| Fig. 1 Structures and their catalytic activity of Al complexes bearing Schiff base ligands. | |
A possible coordination–insertion mechanism6a (Fig. 2) was reported, in which one Al atom serves as the Lewis acid to activate the monomer by the monomer coordination. An alkoxy group on the second Al atom initiates the carbonyl group of the monomer, and monomer ring opens into another alkoxy group. The polymer chain alternates between two Al atoms, making it an extremely efficient polymerization process. Carpentier6e reported that the Al–Al distance in his study is 2.8–3.0 Å, which could open up a cooperatively activation mechanism.
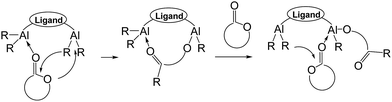 |
| Fig. 2 Possible coordination–insertion mechanism of cyclic ester ROP by using dinuclear Al complexes as catalysts. | |
Dinuclear Ti complexes7 bearing hydrazine-bridging Schiff base ligands shown in Fig. 3, have been used as catalysts in L-lactide polymerization, where they had higher catalytic activity than did mononuclear Ti complexes bearing Schiff base ligands. The Ti–Ti distance in that study was 3.244 Å with two bridging isopropoxides. Hydrazine-bridging Schiff base ligands appear to be the perfect ligands for use in a dinuclear Al system in CL polymerization. Herein, a series of hydrazine-bridging Schiff base ligands, salen ligands, Schiff base ligands, and associated Al complexes were synthesized. The catalytic comparison in CL polymerization between di- and mononuclear Al complexes and the possible mechanism using density functional theory (DFT) were studied.
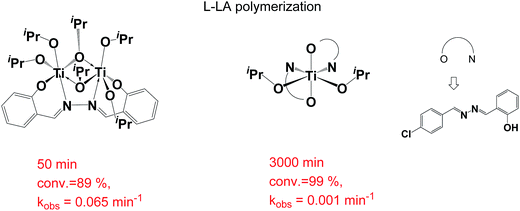 |
| Fig. 3 Comparison of L-LA polymerization between di- and mononuclear Ti complexes. | |
2. Results and discussion
2.1. Synthesis and characterization of Al complexes
Symmetrical and asymmetrical hydrazine-bridging Schiff base ligands,7 and salen ligands8 were synthesized using acid-catalyzed condensation following the literature procedures. The synthesis of Al complexes was described in Experimental section, and Al complexes were obtained as evidenced by the appearance of methyl groups showed a singlet peak in the upfield region (−0.5 to −0.75 ppm) and an 6
:
1 integral ratio (two methyl groups
:
one imine proton) in 1H NMR spectra. The crystal of LClBu-AlMe2 (5) was observed in CDCl3 in the nuclear magnetic resonance (NMR) tube at −20 °C after 1 month.
The X-ray structure of LClBu-AlMe2 (5) (Fig. 4, CCDC 1482938) illustrates the distorted tetrahedral geometry of the Al complex with two methyl groups. The angles of the N(1)–Al(1)–O(1) and C(23)–Al(1)–C(24) planes are 93.32(6)° and 119.59(8)°, respectively. The distances of Al(1)–O(1), Al(1)–N(1), Al(1)–C(23), and Al(1)–C(24) are 1.7624(12), 1.9923(15), 1.9563(19), and 1.9587(19) Å, respectively.
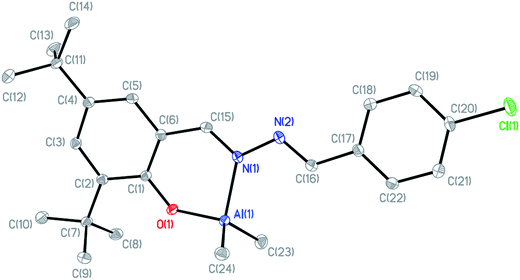 |
| Fig. 4 Molecular structure of LClBu-AlMe2 (5) as 30% probability ellipsoids (all of the hydrogen atoms were omitted for clarity). | |
2.2. Polymerization of ε-caprolactone
CL polymerization in toluene using Al complexes as catalysts with BnOH as the initiator was investigated in a nitrogen atmosphere at room temperature (Table 1 and Fig. 5). As shown in entries 1–8 of Table 1, all Al complexes were active in CL polymerization, and dinuclear Al complexes, LN–NH-Al2Me4 (2) and LN–NBu-Al2Me4 (3), and mononuclear Al complex, LClH-AlMe2 (2), exhibited a low controllability of larger MnGPC and a broad polydispersity index (PDI, 1.70–2.18) compared with other Al complexes, and it may be ascribed to the transesterification9e or produce inactive impurity after the reaction of BnOH and Al complexes. In dinuclear Al complexes, the catalytic activity of LN2Bu-Al2Me4 (1) bearing a hydrazine-bridging Schiff base ligand was higher (approximately 3- to 11-fold) than that of Al complexes bearing salen and Schiff base ligands. This reveals that the dinuclear Al complex bearing a hydrazine-bridging Schiff base ligand has greater cooperation than those bearing salen ligands. LN–NBu-Al2Me4 (1) exhibited a lower polymerization rate than did LN–NH-Al2Me4 (2), revealing that a steric bulky ligand decreased the catalytic activity of Al complexes. However, in mononuclear Al complexes, the opposite phenomenon was observed, similar to in the literature.4f This may be because the cooperation between the two Al centers was blocked by the steric bulky ligand in the dinuclear system. In addition, mononuclear Al complexes bearing hydrazine-bridging Schiff base ligands, LClH-AlMe2 (4) and LClBu-AlMe2 (5), revealed the greater catalytic activity than those, LHBu-AlMe2 (6), LBu-AlMe2 (7), and LBn-AlMe2 (8), bearing normal Schiff base ligands. This may be attributed to hydrazine group, as an electron withdrawing group, increasing the Lewis acidity of the Al center. On the basis of the linear relationship between MnGPC and ([CL]0 × conv.)/[BnOH] exhibited in Fig. 6, polymerizing CL by using LN2Bu-Al2Me4 (1) as the catalyst demonstrated high controllability with a narrow PDI.
Table 1 ε-Caprolactone polymerization by using each of the Al complexes as catalysts at room temperatured
Entry |
Catalyst |
Time (min) |
Conv.a (%) |
MnCalb |
MnGPCc |
MnNMRb |
PDIc |
kobs (error)/min |
Obtained from 1H NMR analysis. Calculated from the molecular weight of monomer × [monomer]0/[BnOH]0 × conversion yield + Mw(BnOH). Obtained from GPC analysis and calibration based on the polystyrene standard. Values of MnGPC are the values obtained from GPC times 0.56. Reaction condition: toluene (5 mL), [CL] = 2.0 M, [CL] : [Cat] : [BnOH] = 100 : 0.5 : 2. Reaction condition: toluene (5 mL), [CL] = 2.0 M, [CL] : [Cat] : [BnOH] = 100 : 1 : 2. Reaction condition: toluene (5 mL), [CL] = 2.0 M, [CL] : [Cat] : [BnOH] = 100 : 0.25 : 1.0. Reaction condition: toluene (5 mL), [CL] = 2.0 M, [CL] : [Cat] : [BnOH] = 100 : 1 : 4. Reaction condition: toluene (5 mL), [CL] = 2.0 M, [CL] : [Cat] : [BnOH] = 100 : 2 : 8. Reaction condition: toluene (5 mL), [CL] = 4.0 M, [CL] : [Cat] : [BnOH] = 200 : 0.5 : 2. Reaction condition: toluene (5 mL), [CL] = 6.0 M, [CL] : [Cat] : [BnOH] = 300 : 0.5 : 2. Reaction condition: toluene (5 mL), [CL] = 8.0 M, [CL] : [Cat] : [BnOH] = 400 : 0.5 : 2. Not available. |
1d |
LN2Bu-Al2Me4 (1) |
60/90 |
80/88 |
5100 |
7200 |
5900 |
1.20 |
0.0261 (22) |
2d |
LN–NH-Al2Me4 (2) |
60/1395 |
46/95 |
5500 |
11 800 |
11 200 |
2.18 |
0.0085 (4) |
3d |
LN–NBu-Al2Me4 (3) |
60/1330 |
9/100 |
5800 |
11 600 |
5800 |
1.70 |
0.0023 (8) |
4e |
LClH-AlMe2 (4) |
60/720 |
22/99 |
5800 |
16 100 |
11 000 |
1.73 |
0.0065 (7) |
5e |
LClBu-AlMe2 (5) |
60/230 |
28/95 |
5500 |
5000 |
3700 |
1.16 |
0.0098 (4) |
6e |
LHBu-AlMe2 (6)4r |
60/1260 |
18/94 |
5500 |
5900 |
6700 |
1.15 |
0.0021 (1) |
7e |
LBu-AlMe2 (7) |
60/360 |
17/90 |
5300 |
6900 |
6900 |
1.16 |
0.0065 (3) |
8e |
LBn-AlMe2 (8) |
60/2560 |
0/96 |
5600 |
7700 |
4200 |
1.16 |
0.0014 (5) |
9f |
LN2Bu-Al2Me4 (1) |
1000 |
96 |
11 100 |
29 700 |
11 500 |
1.39 |
0.0035 (2) |
10g |
LN2Bu-Al2Me4 (1) |
30 |
97 |
2900 |
3100 |
4700 |
1.06 |
0.1413 (72) |
11h |
LN2Bu-Al2Me4 (1) |
20 |
99 |
1500 |
1600 |
2300 |
1.06 |
0.2541 (127) |
12i |
LN2Bu-Al2Me4 (1) |
450 |
90 |
11 500 |
22 300 |
11 500 |
1.31 |
—l |
13j |
LN2Bu-Al2Me4 (1) |
1500 |
95 |
16 400 |
47 400 |
17 100 |
1.30 |
—l |
14k |
LN2Bu-Al2Me4 (1) |
1500 |
96 |
22 000 |
57 400 |
20 300 |
1.32 |
—l |
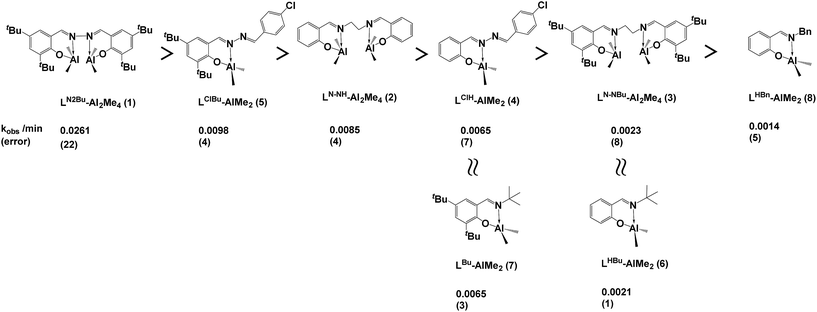 |
| Fig. 5 Comparison of the catalytic activity of di- and mononuclear Al complexes. | |
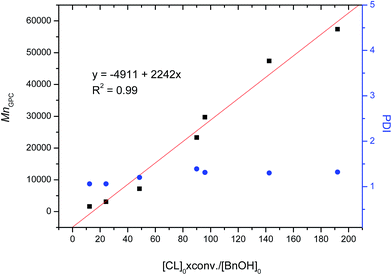 |
| Fig. 6 Linear plot of MnGPC versus [CL]0 × conv./[BnOH]0 with blue dots indicating polydispersity indices (Table 1, entries 1 and 9–14). | |
The 1H NMR spectrum of PCL (Fig. 7, PCL from entry 11, Table 1) shows that peaks at 7.36 ppm (C6H5CH2–, peak a), 5.12 ppm (C6H5CH2–, peak b), and 3.66 ppm (CH2CH2OH, peak c) with an integral ratio of 5
:
2
:
2 between Ha, Hb, and Hc′, indicating that the polymer chain should be capped with one benzyl ester and one hydroxy end and suggesting that the polymerization occurs through the insertion of a benzyl alkoxy group into CL.
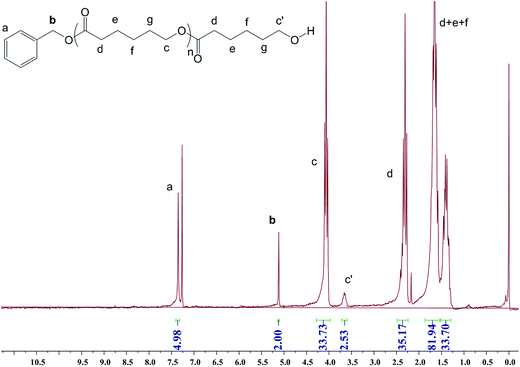 |
| Fig. 7 1H NMR spectrum of PCL in CDCl3 catalyzed by LN2Bu-Al2Me4 (1) with BnOH (entry 11, Table 1). | |
2.3. Kinetic studies of CL polymerization catalyzed by LN2Bu-Al2Me4
Kinetic studies were performed at room temperature with respect to the ratio of [CL]0/[LN2Bu-Al2Me4 (1) + 4BnOH] ([CL] = 2.0 M in 5 mL of toluene) as shown in Table S2 and Fig. S2.† The preliminary results indicated a first-order dependency on [CL] (Fig. S2†). By plotting kobs against [LN2Bu-Al2Me4 (1) + 4BnOH], a kprop values of 7.37 (M−1 min−1) was obtained (Fig. 8). Polymerizing CL by using LN2Bu-Al2Me4 (1) at room temperature demonstrated the following rate law:
d[CL]/dt = 7.37 × [CL][LN2Bu-Al2Me4 (1) + 4BnOH] |
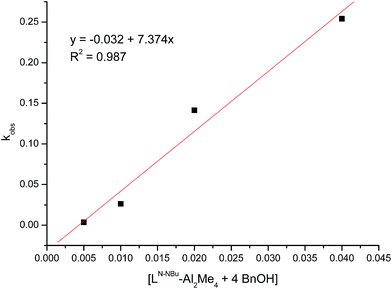 |
| Fig. 8 Linear plot of kobs against [LN2Bu-Al2Me4 (1) + 4BnOH] for CL polymerization with [CL] = 2.0 M in toluene (5 mL). | |
2.4. Polymerization mechanism of LN2Bu-Al2Me4 using DFT calculation
To shed some light on how dinuclear Al catalysts cooperatively polymerizate the CL, DFT calculations were performed for the LN2Bu-Al2Me4 (1) system. To reduce the computational cost, the tBu groups on the hydrazine-bridging Schiff base ligand were replaced by hydrogen atoms and the methoxide was used instead of benzyl alkoxide.
The structure of the active catalyst from the reaction of LN2Bu-Al2Me4 (1) with 4 equivalent of BnOH could not be isolated in the experiments. However, the 1H NMR spectrum (Fig. S17†) of the mixture of benzyl alcohol and LN2Bu-Al2Me4 (1) revealed that methyl groups associated with Al center disappear, and new methylene groups of benzyl alkoxide grow up. Based on the acceptable assumption that alcohol is able to replace methyl groups3b–e,j–n,4q,r and referring to the crystal structure of dinuclear Ti catalyst previously reported,7 our DFT calculations successfully located the optimized geometry of the dinuclear Al catalyst (Fig. 9). In this C2 symmetric structure, two Al metals are bridged by two alkoxide groups with an Al–Al distance of 2.83 Å. The coordination geometry of the Al metals is characterized by a distorted square pyramid in which four oxygen atoms form the base and a nitrogen atom is located at the apex. The vacant site opposite to the nitrogen can be used to accommodate the CL monomer.
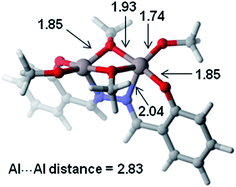 |
| Fig. 9 DFT-optimized structure of an active dinuclear Al catalyst (distances in angstroms). | |
The DFT-calculated reaction pathway and the related free energy changes for the ROP of CL by the dinuclear Al catalyst are illustrated in Fig. 10. The first step is the association of CL and the catalyst. The calculations predict that CL can coordinate with the Al center through the carbonyl oxygen (Ocarbonyl) to form a stable complex a, which is 2.6 kcal mol−1 lower than the separated state. We also considered the Al–CL complex bound through the ester oxygen (Oester) of CL; nonetheless, the binding free energy of the Oester-bound complex is 1.1 kcal mol−1, indicating that it is not a stable complex. That CL prefers coordination to the Al center via Ocarbonyl rather than Oester has been reported previously.4s
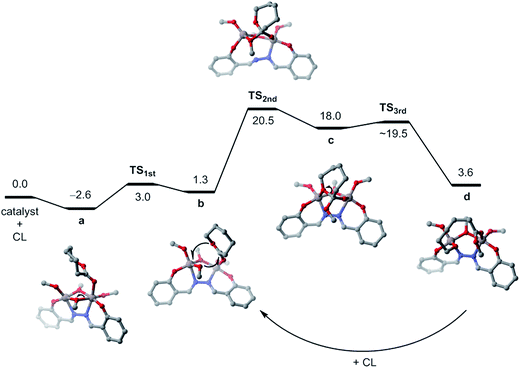 |
| Fig. 10 Possible reaction path for the ring-opening polymerization of ε-caprolactone catalyzed by a dinuclear Al catalyst; the relative free energies are shown in kcal mol−1 and the geometries of TS1st and TS3rd respectively resemble those of b and c and are therefore not shown. | |
Upon association with the CL monomer, one of the bridging alkoxides leaves the CL-coordinated Al center (a → b) and then inserts into the activated carbonyl carbon (b → c). Notice that during the alkoxide insertion, the ester oxygen of CL concurrently coordinates to the other Al center but the ring structure of CL remains closed. The CL in c immediately undergoes ring opening to achieve a more thermodynamically more stable state d. It is assumed that the free CL monomers could subsequently attack the Ocarbonyl-coordinated Al center (right Al site) of d and dissociate the carbonyl group of the open-ring CL to generate a new propagating alkoxide; that is, return to state b to accomplish the catalytic cycle. According to the present DFT calculations, the rate of propagation is determined by the step of alkoxide insertion b → c with an activation free energy of 19.2 kcal mol−1. This theoretical value is in good agreement with the experimental measurement of the propagating rate constant kprop = 7.37 M−1 min−1, which equates to an activation free energy of 18.7 kcal mol−1 using the Eyring equation.
3. Conclusions
A comparison of CL polymerization between mononuclear Al complexes bearing Schiff base ligands, and dinuclear Al complexes bearing hydrazine-bridging Schiff base and salen ligands was investigated. Mononuclear Al complexes with steric bulky groups increased the catalytic activity, whereas the opposite phenomenon was observed in dinuclear Al complexes bearing salen ligands. Steric repulsion may reduce the cooperatively activation mechanism in a dinuclear Al system. LN2Bu-Al2Me4 (1) bearing a hydrazine-bridging Schiff base ligand had the highest catalytic activity (approximately 3- to 11-fold), higher than that of dinuclear Al complexes bearing salen ligands and mononuclear Al complexes bearing Schiff base ligands. A mechanism study using DFT calculations revealed that the cooperation that coordinated the CL with one Al center was initiated by the benzyl alkoxide of another Al center.
4. Experimental section
Standard Schlenk techniques and a N2-filled glovebox were used all over the isolation and treatment of all the compounds. Solvents, ε-caprolactone, and deuterated solvents were purified prior to use. Salicylaldehyde, 3,5-di-tert-butyl-2-hydroxybenzaldehyde, 4-chlorobenzaldehyde, hydrazine monohydrate, ethane-1,2-diamine, benzyl amine, and benzyl alcohol were purchased from Aldrich. 1H and 13C NMR spectra were recorded on a Varian Gemini2000-200 (200 MHz for 1H and 50 MHz for 13C) spectrometer with chemical shifts given in ppm from the internal TMS or center line of CDCl3. Microanalyses were performed using a Heraeus CHN-O-RAPID instrument. The gel permeation chromatography (GPC) measurements were performed on a Waters 1515 Isotratic HPLC pump system equipped with a differential Waters 2414 refractive index detector using THF (HPLC grade) as the eluent. The chromatographic column was a Water Styragel Column (HR4E), and the calibration curve was made by polystyrene standards to calculate Mn(GPC). Ligands of LN2Bu-H,7 LN–NH-H,9a LN–NBu-H,9b LClH-H,7a LHBu-H,9c LBu-H,9d LBn-H,11 and Al complexes of LHBu-AlMe2 (6) (ref. 3d and 4r) and LBu-AlMe2 (7) (ref. 10) were prepared following literature procedures. All the symmetrical ligands were reacted with two equivalent of AlMe3 in toluene to obtain a moderate yield of Al compounds (Scheme 1(A) and (B)). The synthesis of LHBu-AlMe2 (6), LBu-AlMe2 (7), LHBn-AlMe2 (8), LClH-AlMe2 (4), and LClBu-AlMe2 (5) was similar to the aforementioned method except that one equivalent of AlMe3 was used. DFT geometry optimizations were carried out at M06/6-31G* level combined with the D3 version of Grimme's dispersion correction. Calculations were performed by Gaussian 09 program.
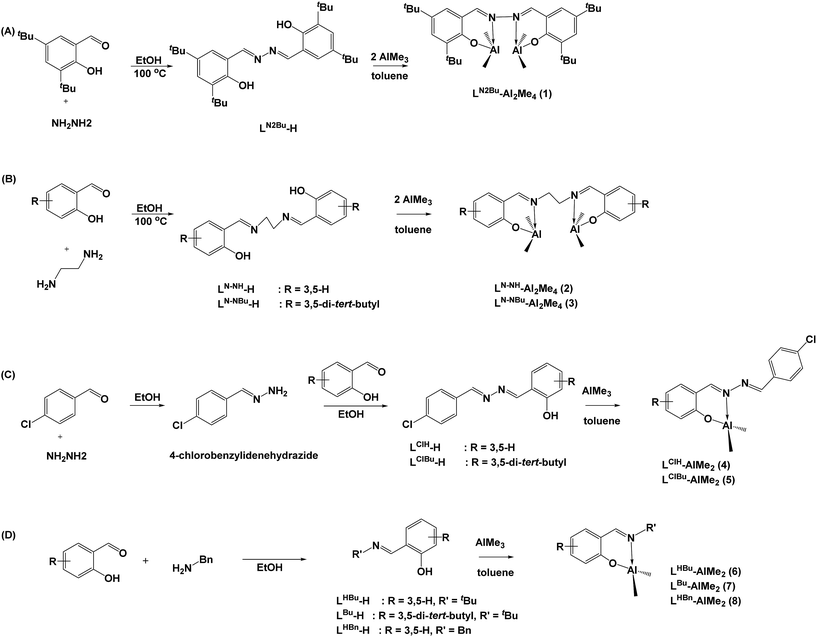 |
| Scheme 1 Synthesis of hydrazine-bridging Schiff base ligands, salen ligands, and associated Al complexes. | |
4.1. Synthesis of LClBu-H
4-Chlorobenzaldehyde (7.03 g, 50 mmol) was added drop-wise into an ethanol solution (100 mL) of the hydrazine monohydrate (5.0 g, 0.10 mol) at 0 °C for 6 h. Volatile materials were removed under vacuum to give a white powder. 3.685 g of the white powder was reacted with 3,5-di-tert-butyl-2-hydroxybenzaldehyde (5.85 g, 25 mmol) in ethanol (100 mL) and refluxed for one day. The product precipitated as a yellow solid which was filtered. Yield: 6.05 g (65%). 1H NMR (CDCl3, 200 MHz): δ 8.77, 8.61 (2H, s, CH
N), 7.80, 7.43 (4H, d, J = 8 Hz, ArH), 7.46, 7.17 (2H, s, ArH), 7.05–6.92 (2H, m, ArH). 13C NMR (CDCl3, 50 MHz): δ 166.68, 165.24 (C
N), 160.23, 157.03, 141.10, 136.80, 132.26, 132.33, 129.69, 128.12, 126.97, 116.79 (Ar), 35.10, 34.15 (C(CH3)3), 31.45, 29.44 (C(CH3)3). Mp = 155 °C.
4.2. Synthesis of LN2Bu-Al2Me4
A mixture of LN2Bu-H (2.32 g, 5 mmol) and AlMe3 (6 mL, 2.0 M in toluene, 12 mmol) in toluene (25 mL), was stirred at 0 °C for one day. Volatile materials were removed under vacuum to give red mud and then it was washed with hexane (50 mL). The product precipitated as a deep yellow powder which was filtered. Yield: 2.45 g (80%). 1H NMR (CDCl3, 200 MHz): δ 8.57 (2H, s, CH
N), 7.62 (2H, s, Ar-H), 6.95 (2H, s, Ar-H), 1.42, 1.31 (36H, s, C(CH3)3), −0.60 (12H, s, Al(CH3)2). 13C NMR (CDCl3, 50 MHz): δ 167.52 (C
N), 162.30, 140.85, 140.45, 134.26, 128.81, 116.18 (Ar), 35.30, 34.11 (C(CH3)3), 31.18, 29.28 (C(CH3)3), −8.79 (Al(CH3)2). Anal. calcd (found) for C34H54N2O2Al2: C, 70.80 (71.14); H, 9.44 (9.74); N, 4.86 (4.76)%. Mp = 224 °C.
4.3. Synthesis of LN–NH-Al2Me4
Using a method similar to that for LN2Bu-Al2Me4 expect LN–NH-H was used in place of LN2Bu-H. Yield: 0.99 g (52%). 1H NMR (CDCl3, 200 MHz): δ 8.01 (2H, s, CH
N), 7.42 (2H, t, J = 8.0 Hz, Ar-H), 7.04 (2H, d, J = 8.0 Hz, Ar-H), 6.89 (2H, d, J = 8.0 Hz, Ar-H), 6.71 (2H, t, J = 8.0 Hz, Ar-H), 3.93 (4H, s, NCH2CH2N), −0.67 (12H, s, Al(CH3)2). 13C NMR (CDCl3, 50 MHz): δ 173.25 (C
N), 168.47, 164.08, 137.55, 134.84, 121.59, 117.82 (Ar), 56.21 (NCH2CH2N), −9.31 (Al(CH3)2). Anal. calcd (found) for C20H26N2O2Al2: C, 63.15 (63.75); H, 6.89 (6.71); N, 7.36 (7.55)%. Mp = 153 °C.
4.4. Synthesis of LN–NBu-Al2Me4
Using a method similar to that for LN2Bu-Al2Me4 expect LN–NBu-H was used in place of LN2Bu-H. Yield: 0.37 g (12%). 1H NMR (CDCl3, 200 MHz): δ 8.01 (2H, s, CH
N), 7.50, 6.86 (2H, s, Ar-H), 3.87 (4H, s, NCH2CH2N), 1.39, 1.22 (36H, s, C(CH3)3), −0.69 (12H, s, Al(CH3)2). 13C NMR (CDCl3, 50 MHz): δ 174.23 (C
N), 161.73, 140.32, 139.20, 132.54, 128.83, 117.86 (Ar), 56.61 (NCH2CH2N), 35.22, 33.93 (C(CH3)3), 31.24, 29.27 (C(CH3)3), −9.18 (Al(CH3)2). Anal. calcd (found) for C36H58N2O2Al2: C, 71.49 (71.82); H, 9.67 (9.56); N, 4.63 (4.99)%. Mp = 180 °C.
4.5. Synthesis of LClH-AlMe2
A mixture of LCl–H-H (1.29 g, 5 mmol) and AlMe3 (3 mL, 2.0 M in toluene, 6 mmol) in toluene (25 mL), was stirred at 0 °C for one day. Volatile materials were removed under vacuum to give red viscous mud and then it was washed with hexane (50 mL). The product precipitated as a yellow powder which was filtered. Yield: 0.13 g (8%). 1H NMR (CDCl3, 200 MHz): δ 8.72, 8.68 (2H, s, CH
N), 7.74, 7.49 (2H, d, J = 8.0 Hz, Ar-H), 7.29, 7.28 (2H, m, Ar-H), 6.94, 6.84 (2H, m, Ar-H), −0.62 (6H, s, Al(CH3)2). 13C NMR (CDCl3, 50 MHz): δ 169.45, 164.49 (C
N), 158.36, 138.49, 137.91, 135.14, 131.09, 129.79, 129.38, 121.61, 118.20, 117.19 (Ar), −9.98 (Al(CH3)2). Anal. calcd (found) for C16H16N2O2ClAl2: C, 61.06 (60.55); H, 5.12 (5.44); N, 8.90 (8.45)%. Mp = 120 °C.
4.6. Synthesis of LClBu-AlMe2
Using a method similar to that for LClH-AlMe2 expect LClBu-H was used in place of LClH-H. Yield: 1.59 g (74%). 1H NMR (CDCl3, 200 MHz): δ 8.69 (2H, s, CH
N), 7.72, 7.47 (4H, d, J = 8.0 Hz, Ar-H), 7.59, 7.10 (2H, s, Ar-H), 1.42, 1.31 (18H, s, C(CH3)3), −0.63 (6H, s, Al(CH3)2). 13C NMR (CDCl3, 50 MHz): δ 170.57, 162.13 (C
N), 157.24, 140.34, 139.74, 138.12, 133.10, 131.38, 129.62, 128.99, 116.76 (Ar), 35.25, 34.07 (C(CH3)3), 31.27, 29.30 (C(CH3)3), −8.83 (Al(CH3)2). Anal. calcd (found) for C24H32N2OClAl: C, 67.51 (67.75); H, 7.55 (7.47); N, 6.56 (6.66)%. Mp = 150 °C.
4.7. Synthesis of LBn-AlMe2
Using a method similar to that for LClH-AlMe2 expect LBn-H was used in place of LClH-H. Yield: 1.09 g (82%). 1H NMR (CDCl3, 200 MHz): δ 7.88 (1H, s, CH
N), 7.35 (5H, br, Bn-H) 7.17–6.95, 6.66–6.57 (4H, m, Ar-H), 5.15, 4.80 (2H, d, J = 14 Hz, CH2Ph), −0.85 (6H, s, Al(CH3)2). 13C NMR (CDCl3, 50 MHz): δ 167.36 (C
N), 163.89, 137.56, 134.28, 133.08, 129.26, 128.67, 128.15, 119.80, 116.65, 114.61 (Ar), 58.65 (CH2Ph), −7.74 (Al(CH3)2). Anal. calcd (found) for C16H18NOAl: C, 71.89 (71.48); H, 6.79 (7.01); N, 5.24 (5.33)%. Mp = 125 °C.
4.8. General procedures for the polymerization of PCL
A typical polymerization procedure was exemplified by the synthesis of entry 1 (Table 1) using complex LN2Bu-Al2Me4 as a catalyst and BnOH as an initiator. The polymerization conversion was analyzed by 1H NMR spectroscopic studies. Toluene (5.0 mL) was added to a mixture of complex LN2Bu-Al2Me4 (0.058 g, 0.1 mmol) and CL (1.14 g, 10 mmol) at room temperature. After the solution was stirred for 90 min, the reaction was then quenched by adding to a drop of ethanol, and the polymer was precipitated pouring into n-hexane (80.0 mL) to give white solids. The white solid was dissolved in CH2Cl2 (5.0 mL) and then n-hexane (70.0 mL) was added to give white crystalline solid. Yield: 1.09 g (95%).
4.9. Computational methods
The B3LYP functional12 was adopted in the present DFT calculations. To remedy the inaccurate treatment of the B3LYP method in dispersion force, the D3 version of dispersion correction developed by Grimme's group was added.13 Geometry optimizations were carried out in the gas phase at B3LYP-D3/6-31+G(d) level. The nature of the obtained stationary points was confirmed by vibrational frequency analyses at the same level of theory. To obtain more accurate energies, single point energy calculations employing a larger basis set 6-311+G(d,p) were performed and the solvent effects of toluene treated by the SMD continuum solvation model14 were included. Pure d basis functions (i.e., 5 d functions) were used in the present calculations. Thermal corrections to Gibbs free energy were done at the standard conditions of 298.15 K and 1 atm. Connections between transition states and their corresponding intermediates were justified by geometry optimizations with initial structures derived from displacing the geometries of transition states a little bit along the imaginary vibrational vector. All calculations were accomplished by the Gaussian 09 program.15
We would like to mention that our endeavors to locate the transition state TS3rd for the ring-opening step (c → d) failed. Instead, we performed the calculation of potential energy surface scan from c to d with increasing the Ccarbonyl–Oester bond length and approximately identified the maximum point along the scan path as the TS3rd. Vibrational frequency analysis of this approximate TS3rd indeed shows an imaginary vibration corresponding to the Ccarbonyl–Oester bond stretching.
Acknowledgements
This study is supported by Kaohsiung Medical University “Aim for the top 500 universities grant” under Grant No. KMU-DT105009, NSYSU-KMU JOINT RESEARCH PROJECT, (#NSYSUKMU 105-I004), and the Ministry of Science and Technology (Grant MOST 105-2113-M-037-007). We thank Center for Research Resources and Development at Kaohsiung Medical University for the instrumentation and equipment support.
References
-
(a) W. Mattanavee, O. Suwantong, S. Puthong, T. Bunaprasert, V. P. Hoven and P. Supaphol, ACS Appl. Mater. Interfaces, 2009, 1, 1076–1085 CrossRef CAS PubMed;
(b) N. E. Zander, J. A. Orlicki, A. M. Rawlett and T. P. Beebe Jr, ACS Appl. Mater. Interfaces, 2012, 4, 2074–2081 CrossRef CAS PubMed;
(c) S. Tarafder and S. Bose, ACS Appl. Mater. Interfaces, 2014, 6, 9955–9965 CrossRef CAS PubMed;
(d) O. Castaño, N. Sachot, E. Xuriguera, E. Engel, J. A. Planell, J.-H. Park, G.-Z. Jin, T.-H. Kim, J.-H. Kim and H.-W. Kim, ACS Appl. Mater. Interfaces, 2014, 6, 7512–7522 CrossRef PubMed;
(e) E. A. Rainbolt, K. E. Washington, M. C. Biewer and M. C. Stefan, Polym. Chem., 2015, 6, 2369–2381 RSC;
(f) L. C. Palmer, C. J. Newcomb, S. R. Kaltz, E. D. Spoerke and S. I. Stupp, Chem. Rev., 2008, 108, 4754–4783 CrossRef CAS PubMed;
(g) D. J. A. Cameron and M. P. Shaver, Chem. Soc. Rev., 2011, 40, 1761–1776 RSC;
(h) J. Nicolas, S. Mura, D. Brambilla, N. Mackiewicz and P. Couvreur, Chem. Soc. Rev., 2013, 42, 1147–1235 RSC;
(i) C. G. Palivan, R. Goers, A. Najer, X. Zhang, A. Car and W. Meier, Chem. Soc. Rev., 2016, 45, 377–411 RSC.
-
(a) C. A. Wheaton, P. G. Hayes and B. J. Ireland, Dalton Trans., 2009, 4832–4846 RSC;
(b) S. M. Guillaume, E. Kirillov, Y. Sarazin and J.-F. Carpentier, Chem.–Eur. J., 2015, 21, 7988–8003 CrossRef CAS PubMed;
(c) J.-F. Carpentier, Organometallics, 2015, 34, 4175–4189 CrossRef CAS;
(d) S. Bellemin and S. Dagorne, Chem. Rev., 2014, 114, 8747–8774 CrossRef PubMed;
(e) E. Piedra-Arroni, A. Amgoune and D. Bourissou, Dalton Trans., 2013, 42, 9024–9029 RSC;
(f) N. Ajellal, J.-F. Carpentier, C. Guillaume, S. M. Guillaume, M. Helou, V. Poirier, Y. Sarazin and A. Trifonov, Dalton Trans., 2010, 39, 8363–8376 RSC;
(g) J. N. Hoskins and S. M. Grayson, Polym. Chem., 2011, 2, 289–299 RSC;
(h) C. M. Thomas, Chem. Soc. Rev., 2010, 39, 165–173 RSC;
(i) A. Arbaoui and C. Redshaw, Polym. Chem., 2010, 1, 801–826 RSC.
-
(a) J. Lewiński, P. Horeglad, M. Dranka and I. Justyniak, Inorg. Chem., 2004, 43, 5789–5791 CrossRef PubMed;
(b) N. Nomura, T. Aoyama, R. Ishii and T. Kondo, Macromolecules, 2005, 38, 5363–5366 CrossRef CAS;
(c) N. Iwasa, J. Liu and K. Nomura, Catal. Commun., 2008, 9, 1148–1152 CrossRef CAS;
(d) J. Liu, N. Iwasa and K. Nomura, Dalton Trans., 2008, 3978–3988 RSC;
(e) N. Iwasa, M. Fujiki and K. Nomura, J. Mol. Catal. A: Chem., 2008, 292, 67–75 CrossRef CAS;
(f) N. Iwasa, S. Katao, J. Liu, M. Fujiki, Y. Furukawa and K. Nomura, Organometallics, 2009, 28, 2179–2187 CrossRef CAS;
(g) J. Wu, X. Pan, N. Tang and C.-C. Lin, Eur. Polym. J., 2007, 43, 5040–5046 CrossRef CAS;
(h) Z.-X. Du, J.-T. Xu, Y. Yang and Z.-Q. Fan, J. Appl. Polym. Sci., 2007, 105, 771–776 CrossRef CAS;
(i) C. Zhang and Z.-X. Wang, J. Organomet. Chem., 2008, 693, 3151–3158 CrossRef CAS;
(j) K. V. Zaitsev, Y. A. Piskun, Y. F. Oprunenko, S. S. Karlov, G. S. Zaitseva, I. V. Vasilenko, A. V. Churakov and S. V. Kostjuk, J. Polym. Sci., Part A: Polym. Chem., 2014, 52, 1237–1250 CrossRef CAS;
(k) A. Gao, Y. Mu, J. Zhang and W. Yao, Eur. J. Inorg. Chem., 2009, 3613–3621 CrossRef CAS;
(l) D. Pappalardo, L. Annunziata and C. Pellecchia, Macromolecules, 2009, 42, 6056–6062 CrossRef CAS;
(m) S. Tabthong, T. Nanok, P. Kongsaeree, S. Prabpaib and P. Hormnirun, Dalton Trans., 2014, 43, 1348–1359 RSC;
(n) M. Shen, W. Zhang, K. Nomura and W.-H. Sun, Dalton Trans., 2009, 9000–9009 RSC;
(o) W. Zhang, Y. Wang, W.-H. Sun, L. Wang and C. Redshaw, Dalton Trans., 2012, 41, 11587–11596 RSC;
(p) K. Matsubara, C. Terata, H. Sekine, K. Yamatani, T. Harada, K. Eda, M. Dan, Y. Koga and M. Yasuniwa, J. Polym. Sci., Part A: Polym. Chem., 2012, 50, 957–966 CrossRef CAS;
(q) I. V. D. Meulen, E. Gubbels, S. Huijser, R. Sablong, C. E. Koning, A. Heise and R. Duchateau, Macromolecules, 2011, 44, 4301–4305 CrossRef;
(r) A. Meduri, T. Fuoco, M. Lamberti, C. Pellecchia and D. Pappalardo, Macromolecules, 2014, 47, 534–543 CrossRef CAS.
-
(a) L. M. Alcazar-Roman, B. J. O'Keefe, M. A. Hillmyer and W. B. Tolman, Dalton Trans., 2003, 3082–3087 RSC;
(b) Z. Tang and V. C. Gibson, Eur. Polym. J., 2007, 43, 150–155 CrossRef CAS;
(c) K. Ding, M. O. Miranda, B. Moscato-Goodpaster, N. Ajellal, L. E. Breyfogle, E. D. Hermes, C. P. Schaller, S. E. Roe, C. J. Cramer, M. A. Hillmyer and W. B. Tolman, Macromolecules, 2012, 45, 5387–5396 CrossRef CAS;
(d) H. Du, A. H. Velders, P. J. Dijkstra, J. Sun, Z. Zhong, X. Chen and J. Feijen, Chem.–Eur. J., 2009, 15, 9836–9845 CrossRef CAS PubMed;
(e) R.-C. Yu, C.-H. Hung, J.-H. Huang, H.-Y. Lee and J.-T. Chen, Inorg. Chem., 2002, 41, 6450–6455 CrossRef CAS PubMed;
(f) H.-C. Tseng, M. Y. Chiang, W.-Y. Lu, Y.-J. Chen, C.-J. Lian, Y.-H. Chen, H.-Y. Tsai, Y.-C. Lai and H.-Y. Chen, Dalton Trans., 2015, 44, 11763–11773 RSC;
(g) D. J. Darensbourg, P. Ganguly and D. Billodeaux, Macromolecules, 2005, 38, 5406–5410 CrossRef CAS;
(h) A. Bhaw-Luximon, D. Jhurry and N. Spassky, Polym. Bull., 2000, 44, 31–38 CrossRef CAS;
(i) P. Hormnirun, E. L. Marshall, V. C. Gibson, A. J. P. White and D. J. Williams, J. Am. Chem. Soc., 2004, 126, 2688–2689 CrossRef CAS PubMed;
(j) X. Pang, H. Du, X. Chen, X. Wang and X. Jing, Chem.–Eur. J., 2008, 14, 3126–3136 CrossRef CAS PubMed;
(k) X. Pang, H. Du, X. Chen, X. Zhuang, D. Cui and X. Jing, J. Polym. Sci., Part A: Polym. Chem., 2005, 43, 6605–6612 CrossRef CAS;
(l) X. Pang, X. Chen, H. Du, X. Wang and X. Jing, J. Organomet. Chem., 2007, 692, 5605–5613 CrossRef CAS;
(m) W.-H. Sun, M. Shen, W. Zhang, W. Huang, S. Liu and C. Redshaw, Dalton Trans., 2011, 40, 2645–2653 RSC;
(n) C. Bakewell, R. H. Platel, S. K. Cary, S. M. Hubbard, J. M. Roaf, A. C. Levine, A. J. P. White, N. J. Long, M. Haaf and C. K. Williams, Organometallics, 2012, 31, 4729–4736 CrossRef CAS;
(o) S. Gong and H. Ma, Dalton Trans., 2008, 3345–3357 RSC;
(p) W.-A. Ma, L. Wang and Z.-X. Wang, Dalton Trans., 2011, 40, 4669–4677 RSC;
(q) H.-C. Tseng, M. Y. Chiang, W.-Y. Lu, Y.-J. Chen, C.-J. Lian, Y.-H. Chen, H.-Y. Tsai, Y.-C. Lai and H.-Y. Chen, Dalton Trans., 2015, 44, 11763–11773 RSC;
(r) M.-C. Chang, W.-Y. Lu, H.-Y. Chang, Y.-C. Lai, M. Y. Chiang, H.-Y. Chen and H. Y. Chen, Inorg. Chem., 2015, 54, 11292–11298 CrossRef CAS PubMed;
(s) M. O. Miranda, Y. DePorre, H. Vazquez-Lima, M. A. Johnson, D. J. Marell, C. J. Cramer and W. B. Tolman, Inorg. Chem., 2013, 52, 13692–13701 CrossRef CAS PubMed.
-
(a) W. Li, W. Wu, Y. Wang, Y. Yao, Y. Zhang and Q. Shen, Dalton Trans., 2011, 40, 11378–11381 RSC;
(b) Y. Wang and H. Ma, Chem. Commun., 2012, 48, 6729–6731 RSC;
(c) X. Pang, R. Duan, X. Li, Z. Sun, H. Zhang, X. Wang and X. Chen, Polym. Chem., 2014, 5, 6857–6864 RSC;
(d) T. R. Forder and M. D. Jones, New J. Chem., 2015, 39, 1974–1978 RSC;
(e) D. J. Darensbourg and O. Karroonnirun, Organometallics, 2010, 29, 5627–5634 CrossRef CAS;
(f) D. J. Darensbourg, O. Karroonnirun and S. J. Wilson, Inorg. Chem., 2011, 50, 6775–6787 CrossRef CAS PubMed;
(g) J. Yang, Y. Yu, Q. Li, Y. Li and A. Cao, J. Polym. Sci., Part A: Polym. Chem., 2005, 43, 373–384 CrossRef CAS;
(h) C. Kan, J. Ge and H. Ma, Dalton Trans., 2016, 45, 6682–6695 RSC;
(i) Z. Sun, R. Duan, J. Yang, H. Zhang, S. Li, X. Pang, W. Chen and X. Chen, RSC Adv., 2016, 6, 17531–17538 RSC;
(j) N. Maudoux, T. Roisonel, V. Dorcet, J.-F. Carpentier and Y. Sarazin, Chem.–Eur. J., 2014, 20, 6131–6147 CrossRef CAS PubMed;
(k) H.-L. Chen, S. Dutta, P.-Y. Huang and C.-C. Lin, Organometallics, 2012, 31, 2016–2025 CrossRef CAS;
(l) C. Kan and H. Ma, RSC Adv., 2016, 6, 47402–47409 RSC;
(m) Z. Qu, R. Duan, X. Pang, B. Gao, X. Li, Z. Tang, X. Wang and X. Chen, J. Polym. Sci., Part A: Polym. Chem., 2014, 52, 1344–1352 CrossRef CAS;
(n) X. Pang, R. Duan, X. Li and X. Chen, Polym. Chem., 2014, 5, 3894–3900 RSC;
(o) L. Li, B. Liu, C. Wu, S. Li, B. Liu and D. Cui, Organometallics, 2014, 33, 6474–6480 CrossRef CAS;
(p) W. Yao, Y. Mu, A. Gao, W. Gao and L. Ye, Dalton Trans., 2008, 3199–3206 RSC;
(q) L. E. N. Allan, J. A. Bélanger, L. M. Callaghan, D. J. A. Cameron, A. Decken and M. P. Shaver, J. Organomet. Chem., 2012, 706–707, 106–112 CrossRef CAS;
(r) S. Doherty, R. J. Errington, N. Housley and W. Clegg, Organometallics, 2004, 23, 2382–2388 CrossRef CAS;
(s) W. Ziemkowska, S. Kucharski, A. Kolodziej and R. Anulewicz-Ostrowska, J. Organomet. Chem., 2004, 689, 2930–2939 CrossRef CAS;
(t) W. Ziemkowska, J. Kochanowski and M. K. Cyrański, J. Organomet. Chem., 2010, 695, 1205–1209 CrossRef CAS.
-
(a) L. Chen, W. Li, D. Yuan, Y. Zhang, Q. Shen and Y. Yao, Inorg. Chem., 2015, 54, 4699–4708 CrossRef CAS PubMed;
(b) X.-F. Yu and Z.-X. Wang, Dalton Trans., 2013, 42, 3860–3868 RSC;
(c) A. Arbaoui, C. Redshaw and D. L. Hughes, Chem. Commun., 2008, 4717–4719 RSC;
(d) H.-L. Han, Y. Liu, J.-Y. Liu, K. Nomura and Y.-S. Li, Dalton Trans., 2013, 42, 12346–12353 RSC;
(e) M. Normand, T. Roisonel, J.-F. Carpentier and E. Kirillov, Chem. Commun., 2013, 49, 11692–11694 RSC;
(f) H.-C. Huang, B. Wang, Y.-P. Zhang and Y.-S. Li, Polym. Chem., 2016, 7, 5819–5827 RSC;
(g) F. Isnard, M. Lamberti, L. Lettieri, I. D'auria, K. Press, R. Troiano and M. Mazzeo, Dalton Trans., 2016, 45, 16001–16010 RSC.
-
(a) H.-C. Tseng, H.-Y. Chen, Y.-T. Huang, W.-T. Lu, Y.-L. Chang, M. Y. Chiang, Y.-C. Lai and H.-Y. Chen, Inorg. Chem., 2016, 55, 1642–1650 CrossRef CAS PubMed;
(b) L. Wang, Q. Su, Q. Wu, W. Gao and Y. Mu, C. R. Chim., 2012, 15, 463–470 CrossRef CAS.
- M. S. Bharara, K. Heflin, S. Tonks, K. L. Strawbridge and A.
E. V. Gorden, Dalton Trans., 2008, 2966–2973 RSC.
-
(a) I. Correia, J. C. Pessoa, M. T. Duarte, M. F. M. da Piedade, T. Jackush, T. Kiss, M. M. C. A. Castro, C. F. G. C. Geraldes and F. Avecilla, Eur. J. Inorg. Chem., 2005, 732–744 CrossRef CAS;
(b) I. A. Fallis, D. M. Murphy, D. J. Willock, R. J. Tucker, R. D. Farley, R. Jenkins and R. R. Strevens, J. Am. Chem. Soc., 2004, 126, 15660–15661 CrossRef CAS PubMed;
(c) B. D. Clercq and F. Verpoort, J. Mol. Catal. A: Chem., 2002, 180, 67–76 CrossRef;
(d) G. Alesso, M. Sanz, M. E. G. Mosquera and T. Cuenca, Eur. J. Inorg. Chem., 2008, 4638–4649 CrossRef CAS;
(e) A. Duda, Z. Florjanczyk, A. Hofman, S. Slomkowski and S. Penczek, Macromolecules, 1990, 23, 1640–1646 CrossRef CAS.
- M. S. Hill, A. R. Hutchison, T. S. Keizer, S. Parkin, M. A. VanAelstyn and D. A. Atwood, J. Organomet. Chem., 2001, 628, 71–75 CrossRef CAS.
- J. U. Ahmad, M. Nieger, M. R. Sundberg, M. Leskelä and T. Repo, J. Mol. Struct., 2011, 995, 9–19 CrossRef CAS.
-
(a) C. Lee, W. Yang and R. G. Parr, Phys. Rev. B: Condens. Matter Mater. Phys., 1988, 37, 785–789 CrossRef CAS;
(b) A. D. Becke, J. Chem. Phys., 1993, 98, 5648–5652 CrossRef CAS.
- S. Grimme, J. Antony, S. Ehrlich and H. Krieg, J. Chem. Phys., 2010, 132, 154104 CrossRef PubMed.
- A. V. Marenich, C. J. Cramer and D. G. Truhlar, J. Phys. Chem. B, 2009, 113, 6378–6396 CrossRef CAS PubMed.
- M. J. Frisch, G. W. Trucks, H. B. Schlegel, G. E. Scuseria, M. A. Robb, J. R. Cheeseman, G. Scalmani, V. Barone, B. Mennucci and G. A. Petersson, et al., Gaussian 09, revision D.01, Gaussian, Inc., Wallingford, CT, 2009 Search PubMed.
Footnote |
† Electronic supplementary information (ESI) available: Polymer characterization data, and details of the kinetic study. CCDC 1482938. For ESI and crystallographic data in CIF or other electronic format see DOI: 10.1039/c7ra02136d |
|
This journal is © The Royal Society of Chemistry 2017 |
Click here to see how this site uses Cookies. View our privacy policy here.