DOI:
10.1039/C7RA03517A
(Paper)
RSC Adv., 2017,
7, 32732-32736
Manipulation of monomer-aggregate transformation of a heptamethine cyanine ligand: near infrared chromogenic recognition of Hg2+†
Received
27th March 2017
, Accepted 20th June 2017
First published on 27th June 2017
Abstract
A novel near infrared optical molecular probe (CyL) derived from heptamethine cyanine dye was developed for the selective detection of Hg2+ over other metal ions in aqueous solutions. The detection conditions for Hg2+ were optimized. A linear range from 1.0 × 10−7 M to 5.0 × 10−6 M and a detection limit of 1.93 × 10−8 M with a correlation coefficient of 0.9992 were achieved under the optimal conditions. In the recognition of Hg2+, CyL alone peaks at 760 nm and exhibits a shoulder at 690 nm. Hg2+ can modulate the aggregation state of CyL through coordination of thiosemicarbazide on CyL and Hg2+, and thus results in an obvious shift in the maximal absorption wavelength of CyL in the NIR region. The ESI mass spectra of CyL–Hg2+ solution have been used to prove the conversion of CyL from monomers to aggregates. Finally, the proposed method has been successfully applied to the determination of Hg2+ in cosmetic samples.
1. Introduction
Chemosensing of heavy metals in aqueous media is a rapidly developing field for their negative impacts on the environment and human health.1 Among these heavy metals, Hg2+ is one of the most toxic ones and a kind of persistent pollutant that is not biodegradable.2,3 Moreover, Hg2+ can easily be absorbed by skin, the respiratory system and do great harm to human health through DNA damage, mitosis impairment, and permanent damage to the central nervous system.4–6 Therefore, the development of a rapid, selective, sensitive and low cost sensor for Hg2+ in aqueous solution has become an urgent need.
In the last few years, various fluorogenic and chromogenic receptors have been developed for the recognition and sensing of Hg2+.7–10 However, most of the them show the absorption and emission wavelength in the ultraviolet-visible (UV-Vis) region (400–650 nm), and often suffer from the interference of signals within short emission wavelengths, and/or background interference, thereby making the accurate analysis of Hg2+ difficult in complex matrices.
To solve these problems, more and more near-infrared dyes are exploited for the determination of Hg2+,11–14 and many of them were applied for Hg2+ imaging in the living cells or in animals and show excellent performance.11,13,14 Heptamethine cyanine dye is one of the important class of near-infrared dyes.15 They have rigid chloro cyclohexenyl rings in the polymethine chain that can increase its photo stability, enhance the fluorescence quantum yield, and provide an ideal site for further modification with amino or phenol substitutions. Moreover, these dyes are among the best known self-associating dyes in aqueous solutions, and this self-association is clearly reflected by changes in the absorption spectra.16 Therefore, applications of heptamethine cyanine dyes as NIR chemosensors for various inorganic and biological related species have been a subject of intensive interest.17–24 However, they, to the best of our knowledge, have seldom been employed as Hg2+ optical probes except for few several reports.12,25 In addition, it has been reported that N and S on hydrazine of thiosemicarbazide could form stable complex with Hg2+.26 Meanwhile, self-aggregation of cyanine dyes can be clearly reflected by changes in the absorption spectra.27
By introducing a thiosemicarbazone unit, an ionophore for heavy metal ions,28 into the cyclohexane bridgehead of heptamethine cyanine dye, herein we presented a new NIR heptamethine cyanine ligand CyL. And then, a new strategy for an efficient chromogenic recognition and determination of Hg2+ in aqueous solutions via modulation of aggregation state of a heptamethine cyanine probe in aqueous medium, leading to a shift in the maximal absorption wavelength in the NIR region has been proposed. Compared with Hg2+-selective optical chemical sensors as reported previously, the as-prepared sensor could recognize and detect Hg2+ in the NIR region, where most of the natural matrix interference could be eliminated, and greatly improved the sensitivity of Hg2+ detection. This present study provided an effective solution for the troublesome problem in the recognition and determination of Hg2+, and has been successfully applied to the determination of Hg2+ in cosmetic samples.
2. Materials and methods
2.1 Chemicals and reagents
1,2,3,3-Tetramethyl-3H-indolium iodide was purchased from Sigma-Aldrich and dry DMF from ACROS ORGANICS. All the other reagents were obtained from Shanghai Chemicals Group Company. The inorganic salts were of the highest purity in the form of nitrates or chlorides. Dry ethanol was distilled from Mg and twice deionized water was further distilled in the presence of KMnO4. Buffer solution (pH 4.0) was prepared by mixing 0.20 M sodium acetate and 0.20 M acetic acid.
2.2 Instrumentation
Bruker AV400 spectrometer (400 MHz for 1H and 100 MHz for 13C) with tetramethylsilane (TMS) as internal standard was used for profiling NMR spectra in d6-DMSO; JEOL JMS-T100LC mass spectrometer was employed to profile mass spectra; and Varian CARY 300 spectrophotometer was utilized to determine absorption spectra. CEM MARS microwave digestion apparatus was used for the pretreatment of cosmetics.
2.3 Synthesis of the new heptamethine cyanine dye
The synthetic procedure for the new heptamethine cyanine dye (CyL) was illustrated in Scheme 1. The heptamethine cyanine 1 was synthesized according to the method reported previously.29
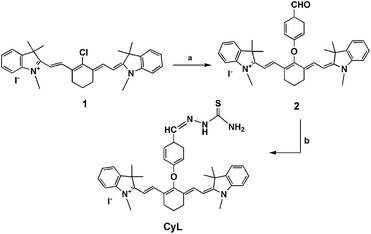 |
| Scheme 1 Synthetic scheme of compound CyL. Conditions: (a) NaH, p-hydroxy-benzaldehyde, dry DMF, room temperature, N2; (b) thiosemicarbazide, dry ethanol, reflux, N2. | |
2.3.1 Synthesis of compound 2. Compound 2 was synthesized as follows: 28.58 mg (0.65 mmol) of sodium hydride (55–65%) and 79.37 mg (0.65 mmol) of p-hydroxybenzaldehyde were dissolved in anhydrous DMF; the reaction mixture was stirred for 30 min under N2 atmosphere at 0 °C, and then allowed to warm to room temperature. This solution was added to the cyanine dye 1 (400.0 mg, 0.65 mmol) in 5.0 mL of dry DMF at room temperature with stirring under N2. The reaction was performed for a total of 12 h, and then diluted with chloroform. After washed with water for several times, the organic layer was dried with Na2SO4. Following removal of chloroform, the residue was purified by flash chromatography with methanol/chloroform (1/30) as eluent to afford 2 (236.30 mg, yield: 46.52%). The newly synthesized compound was characterized using 1H NMR, 13C NMR and mass spectral techniques. The results obtained were as follows: 1H NMR (d6-DMSO, 400 MHz) δ: 1.263 (s, 12H, CH3), 1.965 (quintet, J = 6 Hz, 2H, –CH2–CH2–CH2–), 2.750 (t, J = 6 Hz, 4H, –CH2–CH2–CH2–), 3.618 (s, 6H, CH3), 6.202 (d, J = 14 Hz, 2H, CH
CH), 7.184–7.224 (m, 2H, ArH), 7.375–7.395 (m, 6H, ArH), 7.492 (d, J = 7.6 Hz, 2H, ArH), 7.716 (d, J = 14 Hz, 2H, CH
CH), 8.005 (d, J = 8.4 Hz, 2H, ArH), 9.917 (s, 1H, CHO) (Fig. S1†). 13C NMR (d6-DMSO, 100 MHz): δ 20.569, 23.636, 26.897, 31.252, 48.372, 100.828, 111.146, 115.150, 120.734, 122.193, 124.802, 128.358, 130.950, 132.467, 139.768, 140.772, 142.643, 160.787, 163.569, 172.029, 191.355 (Fig. S2†). ESI mass spectrometry, m/z: 569.3 (M − I)+, 570.3 (M − I + 1)+ (Fig. S3†).
2.3.2 Synthesis of CyL. Compound CyL was synthesized as follows: compound 2 (200.00 mg, 0.28 mmol) and thiosemicarbazide (127.58 mg, 1.40 mmol) were dissolved in dry ethanol, and the reaction mixture was refluxed for 4 hours under N2 atmosphere. After removal of ethanol, the residue was purified through flash chromatography with methanol/chloroform (1/30) as eluent to afford CyL (112.76 mg, yield: 50%). Compound CyL was characterized using 1H NMR, 13C NMR and mass spectral techniques. The results obtained are as follows: 1H NMR (d6-DMSO, 400 MHz) δ: 1.280 (s, 12H, CH3), 1.949 (quintet, J = 5.6 Hz, 2H, –CH2–CH2–CH2–), 2.737 (t, J = 5.6 Hz, 4H, –CH2–CH2–CH2–), 3.611 (s, 6H, CH3), 6.183 (d, J = 14.4 Hz, 2H, CH
CH), 7.169–7.219 (m, 4H, ArH), 7.369 (s, 2H, ArH), 7.377 (s, 2H, NH2), 7.494 (d, J = 7.6 Hz, 2H, ArH), 7.767 (d, J = 14.4 Hz, 2H, CH
CH), 7.862 (d, J = 8.8 Hz, 2H, ArH), 7.969 (d, J = 12.4 Hz, 2H, ArH), 8.187 (s, 1H, CH), 11.391 (s, 1H, NH) (Fig. S4†). 13C NMR (d6-DMSO, 100 MHz): δ 21.215, 24.175, 27.501, 31.747, 48.899, 101.161, 111.614, 115.112, 121.574, 122.747, 125.272, 128.893, 128.980, 130.065, 140.655, 141.296, 141.906, 143.206, 161.005, 162.071, 172.508, 178.263 (Fig. S5†). ESI mass spectrometry, m/z: 642.3 (M − I)+, 643.3 (M − I + 1)+ (Fig. S6†).
2.4 General procedure
Stock solutions containing 1.0 × 10−3 M of CyL and 0.8 × 10−3 M of Hg2+ were prepared in MeOH and redistilled water, respectively. To 10.0 mL of 0.20 M acetate buffer (pH 4.0) solution containing different amounts of Hg2+, small aliquots of CyL stock solution (100 μL) was added. After being mixed thoroughly and allowed to equilibrate for 10 minutes, the NIR absorption spectra were recorded and the ratio R(A666/A760) was tested.
3. Results and discussion
3.1 Spectral characteristics of CyL
CyL is a new derivative of cationic cyanine dyes. Absorption spectral traces of CyL upon coordination with Hg2+ in an optimized acetate buffer (0.20 M) solution of pH 4.0 (MeOH/H2O = 1/99, v/v) were monitored. Fig. 1 showed the absorption spectral changes of CyL (1.0 × 10−5 M) in the presence of Hg2+. CyL solution alone exhibits a maximum at 760 nm and a shoulder at 690 nm. With the increase in Hg2+ concentration, the absorbance at 760 nm decreased, while a new absorption band at a shorter wavelength of 666 nm appeared. The system color also changed from emerald green to bluish green accordingly. These pronounced hypsochromic shift can be ascribed to the H-aggregation of the cyanine dye15 resulting from the coordination of CyL and Hg2+.
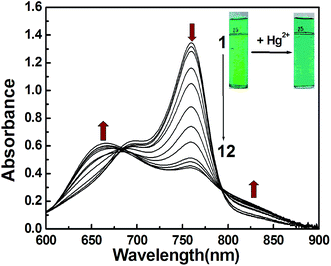 |
| Fig. 1 Vis-NIR spectra of CyL in MeOH–H2O (1/99, v/v) aqueous acetate buffer solution of pH 4.0 in the absence (curve 1) and presence of increasing concentration of Hg2+ (curves 2–12). [CyL]: 1.0 × 10−5 M; [Hg2+] (from curves 2 to 12, 10−6 M): 0.3, 0.5, 0.7, 0.9, 1, 3, 5, 7, 9, 10, 20. Insert: chromogenic response of CyL to 1.0 × 10−5 M of Hg2+ in MeOH–H2O (1/99, v/v) aqueous acetate buffer solution of pH 4.0. | |
3.2 Optimization of experimental conditions
3.2.1 Effect of buffer system and pH. To obtain the optimal conditions, the buffer system and the influence of pH on the sensitivity of this method were investigated. The results showed the best absorbance changes (R/R0, where R0 and R are A666/A760 in the absence and presence of Hg2+, respectively) were obtained at pH 3.0–6.0 (Fig. 2), and the changes of buffer system had minimal effect on detection sensitivity. Thus, in this study, pH 4.0 of HAc–NaAc buffer was selected to control the acidity of the system.
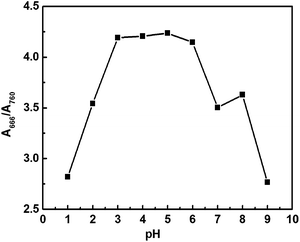 |
| Fig. 2 Effects of pH on the changes of absorbance. [Hg2+] = 8.0 × 10−6 M; [CyL] = 1.0 × 10−5 M; volume of each buffer is 1 mL; other conditions are the same as those described in the general procedure except the buffer system. | |
3.2.2 Effect of CyL concentration. The effect of CyL concentration on Hg2+ measurement was investigated. When concentration of Hg2+ was kept at 1.0 × 10−5 M, R(A666/A760) increased with the increasing amount of CyL. When the concentration was 1.0 × 10−5 M, R reached the maximum. Thus, 1.0 × 10−5 M was chosen as the optimum CyL concentration in the experiment.
3.2.3 Effect of incubation time. Under the optimized conditions as depicted above, the effect of incubation time on R(A666/A760) was tested. As shown in Fig. 3, the system reached equilibrium 10 min following Hg2+ addition, and remained constant for at least 50 min. Thus, it was suggested that the measurement should start 10 min after addition of Hg2+ finish within 50 min.
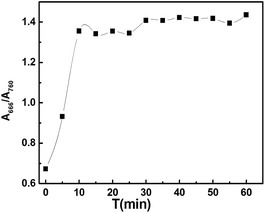 |
| Fig. 3 Effects of time on the absorption response of CyL to Hg2+ in MeOH–H2O (1/99, v/v) aqueous acetate buffer solution of pH 4.0; [CyL] = 1.0 × 10−5 M; [Hg2+] = 5.0 × 10−6 M; other conditions were the same as those described in the general procedure. | |
3.3 Selectivity and interference of foreign ions
To examine the specificity of Hg (CyL)2 complex formation under the presence of environmentally relevant metal ions, a variety of heavy, transition, and main group metal ions were tested in their capability of binding CyL (Fig. 4). It was revealed from Fig. 4 that CyL at 1.0 × 10−5 M exhibited little decrease in absorbance at 760 nm upon addition of 1.0 equiv. of the tested ions including Li+, Na+, K+, Ca2+, Mg2+, Ba2+, Co2+, Cr3+, Cu2+, Cd2+, Fe3+, Ni2+, Mn2+, Zn2+, and Pb2+. Only the addition of Hg2+ resulted in a remarkable reduction of the absorbance at 760 nm and a new absorption at shorter wavelength of 666 nm. This implied the existence of a higher coordination selectivity of CyL toward Hg2+. Competitive experiments further showed that the decrease in R(A666/A760) induced by Hg2+ was not disturbed by the presence of coexisting metal ions (Fig. S7†), indicating the highly specific coordination of CyL to Hg2+. Taken together, CyL could be practically served as a NIR-sensing probe for Hg2+ in aqueous solutions.
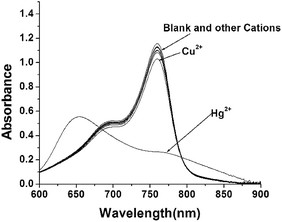 |
| Fig. 4 Absorption response of CyL to various metal ions (K+, Li+, Na+, Zn2+, Ni2+, Co2+, Fe3+, Al3+, Pb2+, Cr3+, Mn2+, Ca2+, Mg2+, Cu2+, Hg2+) in pH 4.0 acetate buffer. [CyL] = 1.0 × 10−5 M, [Mn+] = 1.0 × 10−5 M. | |
3.4 Calibration graphs and analysis of samples
Under the optimized conditions, the titration curves for the measurement of Hg2+ were constructed. Response assays showed that R(A666/A760) increased with the increment of Hg2+ concentration, varied from 0.302 in the absence of Hg2+ to 1.637 after 5.0 × 10−6 M Hg2+ treatment. That was, R increased 4.42 times due to the addition of Hg2+. The curve equation as shown in Fig. 5 was R = 0.284 + 2.648CHg2+, with a correlation coefficient of 0.9992 (N = 14, S.D. = 0.0017) when a CyL concentration of 1.0 × 10−5 M was used. The linear range of quantitative detection for Hg2+ was determined to be 1.0 × 10−7 to 5.0 × 10−6 M with a detection limit of 1.93 × 10−8 M in the used aqueous solution system.
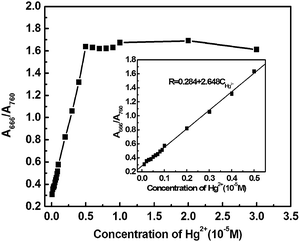 |
| Fig. 5 The absorption titration curves and calibration graphs for spectrophotometric determination of Hg2+ in MeOH–H2O (1/99, v/v) aqueous acetate buffer solution of pH 4.0; [CyL] = 1.0 × 10−5 M; [Hg2+]: (1.0 × 10−7 M to 3.0 × 10−5 M); other conditions were the same as those described in the general procedure. | |
In order to check the potential applicability of this method, we have applied our method to the quantitative determination of Hg2+ in cosmetics. In our experiment, three cosmetics samples were collected and treated according to the literature,30 and then diluted 10-fold for experimental uses. The specific sample pretreatment processes were listed in the footnote of Table 1, and the determination results by this method and by the cold atomic absorption spectrometry (AAS) method were shown in Table 1. It could be seen from Table 1 that the determination results are very close to those obtained by the cold AAS method. These results together with results of the selectivity and competition experiments confirmed that method proposed in the present work was reliable and practical for the determination of Hg2+ in actual samples.
Table 1 Analytical results of cosmetic samples
Samples |
Proposed method founda (10−6 M) |
Hg2+, added (10−6 M) |
Hg2+, found (10−6 M) |
Recovery (%) |
Cold AAS method founda (10−6 M) |
The cosmetics samples were prepared by resolving 1.0 g of each sample into 2.0 mL distilled water and stirring into syrupy liquid, then, they were added 3.0 mL of nitric acid and kept for 12 hours at room temperature. Next, each sample was added 2.0 mL of hydrogen peroxide and treated with microwave digestion. Finally, the resulting solution was diluted into 10 mL, the determination results were obtained by further diluting above solution for 10-fold. |
1 |
0.70 ± 0.1 |
1.00 |
1.72 ± 0.1 |
102 |
0.67 ± 0.1 |
2 |
0.90 ± 0.1 |
1.00 |
1.88 ± 0.1 |
98.5 |
0.86 ± 0.1 |
3 |
Not found |
1.00 |
0.97 ± 0.1 |
97.0 |
Not found |
3.5 Sensing mechanism
The Job's plots of absorbance at 760 nm (Fig. S8†) suggested that a 2
:
1 complex formed between CyL and Hg2+. On the basis of the high affinity of Hg2+ toward thiosemicarbazide,14 the decrease of absorbance at 760 nm could be ascribed to the formation of Hg(CyL)2 complex by the coordination of Hg2+ to N and S on thiosemicarbazide of CyL. This assumption was further supported by the ESI mass spectra of CyL–Hg2+ solution (Fig. S9†), which shows a strong peak at m/z 741.7 and a weak peak at m/z 642.3, assigned to (2CyL − 2I + Hg − 2H)2+ for Hg(CyL)2 complex and (CyL − I)+ for CyL monomer, respectively. Based on these results, sensing mechanism of CyL to Hg2+ was proposed in Scheme 2.
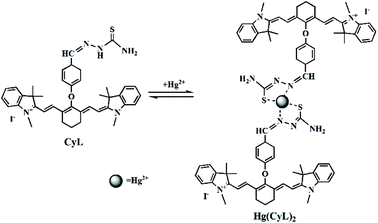 |
| Scheme 2 Proposed mechanism of CyL binding with Hg2+. | |
4. Conclusion
In summary, a new NIR heptamethine cyanine ligand has been proposed for selective binding of Hg2+ based on the monomer-aggregate transformation of the NIR ligand. In addition to simplicity and effectivity, this sensor still showed a limit of detection of 1.93 × 10−8 M in the aqueous solution. The high sensitivity was made possible through the unique sensing mechanism, whereby even small amount of Hg2+ recognized could result in the aggregation of CyL, leading to the changes of absorption spectrum. The tested method proposed CyL as a novel sensor for the detection of Hg2+ by absorption spectrometry in aqueous solution, and could be used as a good alternative to the other sensors for Hg2+.
Acknowledgements
The authors are grateful for the fund support by the Foundation and Cutting-edge Research Projects of Henan Province (162300410019) and the Doctoral Scientific Research Foundation of Xinxiang Medical University (505062).
References
- X. Fu, T. Lou, Z. Chen, M. Lin, W. Feng and L. Chen, ACS Appl. Mater. Interfaces, 2012, 4, 1080 CAS.
- E. M. Nolan and S. J. Lippard, Chem. Rev., 2008, 108, 3443 CrossRef CAS PubMed.
- T. Balaji, S. A. El Safty, H. Matsunaga, T. Hanaoka and F. Mizukami, Angew. Chem., Int. Ed., 2006, 45, 7202 CrossRef CAS PubMed.
- J. Mutter, J. Naumann, R. Schneider, H. Walach and B. Haley, Neuroendocrinol. Lett., 2005, 26, 439 Search PubMed.
- W. F. Fitzgerald, C. H. Lamgorg and C. R. Hammerschmidt, Chem. Rev., 2007, 107, 641 CrossRef CAS PubMed.
- E. M. Nolan and S. J. Lippard, Chem. Rev., 2008, 108, 3443 CrossRef CAS PubMed.
- C. Kumaria, D. Saina, A. Kumara, H. P. Nayeka, S. Debnath, P. Saha and S. Dey, Sens. Actuators, B, 2017, 243, 1181 CrossRef.
- Y. Zhao, L. L. Gui and Z. B. Chen, Sens. Actuators, B, 2017, 241, 262 CrossRef CAS.
- S. R. Tang, P. Tong, M. L. Wang, J. H. Chen, G. W. Li and L. Zhang, Chem. Commun., 2015, 51, 15043 RSC.
- N. Kaur, P. Kaur, G. Bhatia, K. Singh and J. Singh, RSC Adv., 2016, 6, 82810 RSC.
- J. L. Wang, W. L. Li and L. P. Long, Sens. Actuators, B, 2017, 245, 462 CrossRef CAS.
- H. Zheng, X. J. Zhang, X. Cai, Q. N. Bian, M. Yan, G. H. Wu, X. W. Lai and Y. B. Jiang, Org. Lett., 2012, 14, 1986 CrossRef CAS PubMed.
- M. N. Wang, X. J. An and J. Gao, J. Lumin., 2013, 144, 91 CrossRef CAS.
- C. C. Zhao, K. B. Li, N. Xie, M. Zhao and S. Q. Peng, J. Photochem. Photobiol., A, 2014, 290, 72 CrossRef CAS.
- L. Strekowski, M. Lipowska and G. Patonay, J. Org. Chem., 1992, 57, 578 Search PubMed.
- A. Mishra, R. K. Behera, P. K. Behera, B. K. Mishra and G. B. Behera, Chem. Rev., 2000, 100, 1973 CrossRef CAS PubMed.
- G. H. Cheng, J. L. Fan, W. Sun, J. F. Cao, C. Hu and X. J. Peng, Chem. Commun., 2014, 50, 1018 RSC.
- B. H. Li, Y. L. Zhang, F. S. Li, W. Wang, J. Liu, M. Liu, Y. Cui, X. B. Li and B. L. Li, Sens. Actuators, B, 2016, 233, 479 CrossRef CAS.
- K. Yin, F. B. Yu, W. W. Zhang and L. X. Chen, Biosens. Bioelectron., 2015, 74, 156 CrossRef CAS PubMed.
- X. X. Hu, Y. T. Su, Y. W. Ma, X. Q. Zhan, H. Zheng and Y. B. Jiang, Chem. Commun., 2015, 51, 15118 RSC.
- X. Y. Han, F. B. Yu, X. Y. Song and L. X. Chen, Chem. Sci., 2016, 7, 5098 RSC.
- Z. R. Lou, P. Li and K. L. Han, Acc. Chem. Res., 2015, 48, 1358 CrossRef CAS PubMed.
- F. B. Yu, P. Li, G. Y. Li, G. J. Zhao, T. S. Chu and K. L. Han, J. Am. Chem. Soc., 2011, 133, 11030 CrossRef CAS PubMed.
- F. B. Yu, P. Li, B. S. Wang and K. L. Han, J. Am. Chem. Soc., 2013, 135, 7674 CrossRef CAS PubMed.
- C. Kur, Y. Shindo, K. Oka, S. Nishiyama, K. Suzuki and D. Citterio, RSC Adv., 2017, 7, 24970 RSC.
- Y. Yu, L. R. Lin, K. B. Yang, X. Zhong, R. B. Huang and L. S. Zheng, Talanta, 2006, 69, 103 CrossRef CAS PubMed.
- A. Mishra, R. K. Behera, P. K. Behera, B. K. Mishra and G. B. Behera, Chem. Rev., 2000, 100, 1973 CrossRef CAS PubMed.
- T. S. Lobana, A. Sanchez, J. S. Casas, A. Castineiras, J. Sordo and M. S. Garcia-Tasende, Polyhedron, 1998, 17, 3701 CrossRef CAS.
- R. Chin and N. Salazar, J. Heterocycl. Chem., 1996, 33, 1871 CrossRef.
- F. R. Tian, L. H. Yang, X. Y. Ye and Y. L. Liu, J. Chem. Res., 2011, 22, 91 CAS.
Footnote |
† Electronic supplementary information (ESI) available: 1H, 13C NMR and ESI-MS mass spectra of compound 2 and CyL; competitive absorbance response of CyL with Hg2+; absorbance titrations of CyL with Hg2+; ESI-MS spectra of CyL–Hg2+. See DOI: 10.1039/c7ra03517a |
|
This journal is © The Royal Society of Chemistry 2017 |
Click here to see how this site uses Cookies. View our privacy policy here.