DOI:
10.1039/C7RA04905F
(Paper)
RSC Adv., 2017,
7, 31457-31465
Adsorption of H2S on graphane decorated with Fe, Co and Cu: a DFT study
Received
2nd May 2017
, Accepted 6th June 2017
First published on 19th June 2017
Abstract
Herein, density functional theory (DFT) calculations were performed to investigate the adsorption of a H2S molecule on the surface of hydrogenated graphene (graphane). In our results, we found that the appearance of an H-vacancy significantly improved the reactivity of graphane due to the unpaired electrons of the vacancy site. However, small adsorption energy and low charge transfer indicated that the interaction between the H2S molecule and the pure H-vacancy-defected graphane occurred via physisorption. By introducing transition-metal dopants (Fe, Co, and Cu), the adsorption process of the H2S molecule changed to chemisorption. Furthermore, the adsorption of H2S induced a decrease in the band gaps, which could be seen as signal for the detection of H2S gas.
1. Introduction
Air pollution is still a global challenge, and daily human and industrial activities are the major source of pollution to the environment, also harming human health. H2S is a common environmental pollutant, which is usually produced during the preparation process of petroleum and natural gas.1 It is a highly toxic gas to human beings at low concentrations and can cause mortalities at high concentrations.2 Hence, the detection of H2S is a great technological challenge.3 A promising candidate for gas sensors should possess some advantages such as miniature size, higher sensitivity, and low production cost.4–6 Recently, graphene, a two-dimensional and one-carbon-thick material, has attracted significant attention due to its excellent physical and chemical properties since its first synthesis was experimentally achieved in 2004.7–12 Graphene exhibits great promise for the application of gas sensing because it possesses a large surface and high reactivity.13–17 However, studies suggest that the weak interaction between the adsorbents and graphene and the zero band-gap limit its application in gas detection. To solve this problem, many researchers have focused on improving its reactivity. Several common techniques such as the fictionalization using defects18–20 and impurities21–23 have been explored.
Different elements are attached to the surface of graphene to alter its electronic properties and chemical reactivity. Thus, a promising material prepared by exposing graphene to hydrogen plasma is graphane, which was first synthesized in 2009 by Elias et al.24 In graphane, the hydrogen atoms are attached on both sides of graphene, and then, sp2 hybridization in graphene changes to sp3 hybridization.25 Graphane exhibits unique electronic and magnetic properties and has been explored in recent years in the aspects of science and technology, such as transistor and nanoelectronic designs, hydrogen storage, and bio-sensing.26–31 It is a wide band gap semiconductor and overcomes the restrictions of the non-existence of a band-gap in graphene for some application areas such as gas sensing. Furthermore, its excellent mechanical strength and large surface area predicate its promising application for gas sensing. Islam et al.32 studied the interaction of graphane towards CH4 molecules, and the results suggested that the H-vacancy and metal-doping significantly enhanced the methane adsorption. Thus, the introduction of an H-vacancy defect and metal-dopants have been successfully attempted to increase the adsorption stability of graphane towards gas molecules.33–36 In our study, we investigated the adsorption properties of H2S molecules on the pure, H-vacancy-defected, and transition-metal (TM)-doped graphane using density functional theory (DFT) calculations. The TM-dopants considered herein were Fe, Co, and Cu. We believe that our study provides useful information for the application based on graphane for gas sensing.
2. Computational methods and model
All the DFT calculations were performed via the Dmol3 code.37 We used the generalized gradient approximation (GGA) for the exchange-correlation functional, as described by Perdew–Burke–Ernzerhof (PBE).38 Basiuk et al. reported that the PBE functional along with Grimme dispersion correction could be suggested as most suitable for theoretical studies of the interaction between molecules and graphene.39 Therefore, all the results were obtained using the Grimme-corrected PBE functional. We selected DFT semicore pseudopotential (DSSP) as a single effective potential to replace the core electrons.40 A double numerical plus polarization (DNP) was employed as the basis set. The DNP basis set corresponded to a double-ζ quality basis set with a p-type polarization function to hydrogen and d-type polarization functions added to heavier atoms, which was comparable with the Gaussian 6-31G (d, p) basis set and exhibited a better accuracy.41
We applied a 5 × 5 × 1 supercell under the periodic boundary condition on the x and y axes to model the infinite graphane sheet. The vacuum space of 20 Å was set in the direction normal to the sheets to avoid the interactions between periodic images. A 5 × 5 × 1 mesh of k-point and the global orbital cut-off of 5.0 Å were set in the spin-unrestricted calculations. All atoms were allowed to relax. Convergence in energy, force, and displacement were set at 4.54 × 10−4 eV, 1.09 eV Å−1, and 0.005 Å, respectively.
We analyzed the adsorption of H2S on hydrogenated graphene (HG). The adsorption energy was obtained from the expression
Eads = E(H2S/HG) − E(HG) − E(H2S) |
where
E(H
2S/HG) is the total energy of the H
2S molecule adsorbed on hydrogenated graphene,
E(HG) is the total energy of the HG, and
E(H
2S) is the total energy of a free H
2S molecule.
3. Results and discussion
3.1 Structures of HG, VHG, FeVHG, CoVHG, and CuVHG
First, we discussed the geometries of pure graphane (HG) and H-vacancy-defected graphane (VHG), and the optimized structures are presented in Fig. 1. The bond lengths of C–H and C–C in the HG configuration were 1.109 Å and 1.496 Å, respectively, which were consistent with those reported in previous studies.42 Pure graphane was a nonmagnetic semiconductor with a wide gap, which was 3.69 eV as obtained from our calculated results. The gap value was in agreement with the literature, and the little difference was due to the selection of the calculated parameters.25,42,43 After introducing the H-vacancy defect, the Cv atom, which lost an H atom, was labeled in Fig. 1b. The bond lengths of Cv and the adjacent C atoms were 1.469 Å.
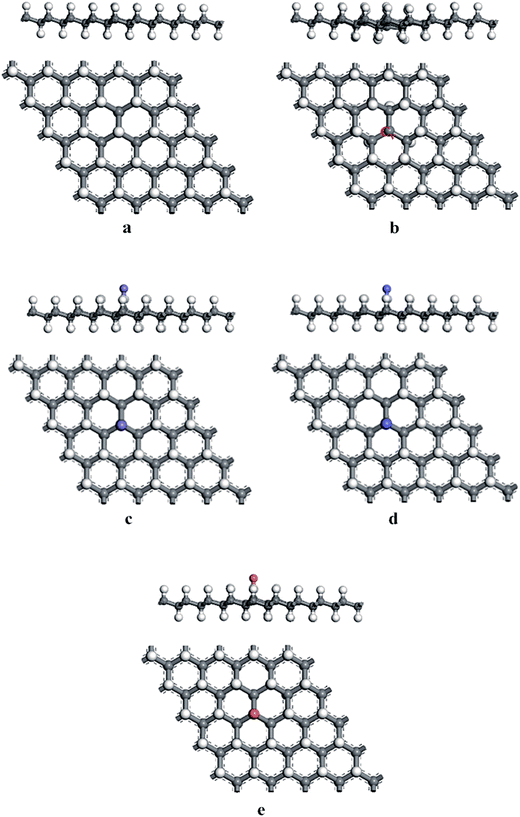 |
| Fig. 1 The optimized structures of (a) HG, (b) VHG, (c) FeVHG, (d) CoVHG, and (e) CuVHG. | |
Second, we considered the configuration of VHG doped with Fe (FeVHG), Co (CoVHG), and Cu (CuVHG). Due to the presence of the H-defect in graphane, the vacancy offered a reactive site to craft adsorbents.35,44 Therefore, we introduced the transition-metal (TM) atoms to the vacancy site. The TM-dopants also preferred to congruously disperse on the graphane surface, which was due to fact that the binding energies were larger than the cohesive energies. The binding energies for the dopants were 3.80 eV, 3.06 eV, and 3.95 eV. Furthermore, the bond lengths of Fe–Cv, Co–Cv, and Cu–Cv were 2.024 Å, 1.977 Å, and 1.967 Å, respectively. The optimized structures were also obtained and are shown in Fig. 1.
To explore the effect of transition-metal dopants on the electronic and magnetic properties of graphane, we performed the density of states (DOS) calculations, as shown in Fig. 2. As shown in Fig. 2a, HG was nonmagnetic and exhibited a wide band gap. However, the H-vacancy released the p electron on the Cv atom and formed a localized state. In our results, VHG exhibited a net magnetic moment of 1 μB. As shown in Fig. 2b, the DOS of the Cv-p orbital split into a spin-up and a spin-down peak around the Fermi level, which revealed that the unpaired electrons of Cv induced a half-metallic behavior. Moreover, the band gap of VHG decreased to 1.70 eV. Therefore, VHG was expected to exhibit higher reactivity to interact with the adsorbents as compared to pure graphane.
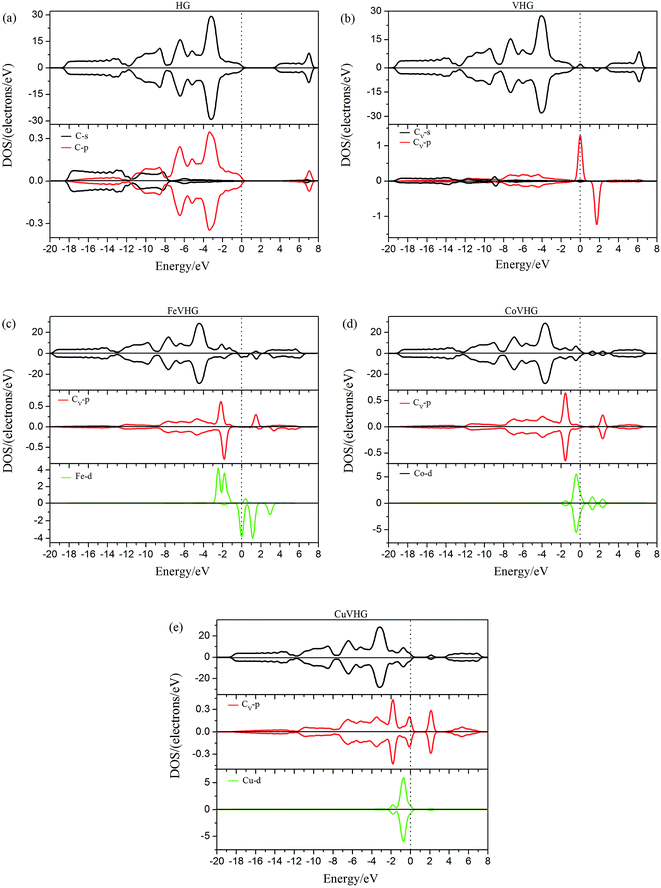 |
| Fig. 2 The PDOS of (a) HG, (b) VHG, (c) FeVHG, (d) CoVHG, and (e) CuVHG. | |
For the cases of VHG decorated with the TM atoms, there was strong hybridization between the Cv-2p and TM-3d orbitals (Fig. 2c–e). Transition-metal atoms are well known for their magnetic properties. In the case of FeVHG, the Fe-3d orbital exhibited obvious net magnetic moments. For the cases of CoVHG and CuVHG, the magnetic properties disappeared due to the formation of chemical bonds between the dopants and the Cv atoms. In general, the introduction of TM-dopants contributed to the DOS around the Fermi level, and the resulting compounds exhibited higher sensitivity to absorb molecules.
3.2 Adsorption of H2S on HG and VHG
Herein, we explored the adsorption of H2S on HG and VHG. According to the geometric symmetry of configuration, there were three initial adsorption structures: the H atom of the H2S molecule close to the graphane surface with the H–S bond parallel to graphane, the H atom of the H2S molecule close to the graphane surface with the H–S bond perpendicular to graphane, and the S atom of the H2S molecule close to the graphane surface. After full relaxation, the calculated results indicated that the S atom of the H2S molecule close to the graphane was the most stable adsorption structure. We performed the most stable optimized configurations of H2S/HG and H2S/VHG, as shown in Fig. 3. Moreover, the corresponding parameters describing the adsorption configurations are summarized in Table 1.
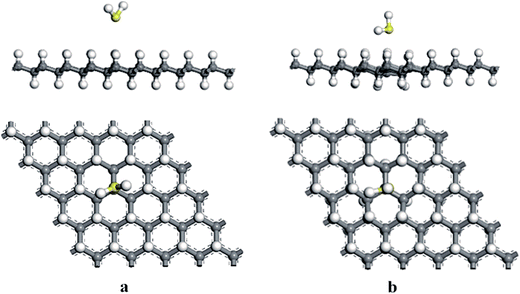 |
| Fig. 3 The optimized structures of (a) H2S/HG and (b) H2S/VHG. | |
Table 1 Summary of the results for the H2S molecule adsorbed on pure graphane (HG) and H-vacancy-defected graphane (VHG). The properties listed are as follows: the distance between the H and S atom of the H2S molecule (dH–S), the angle of the H2S molecule (α), the distance between the S atom of H2S and graphane surface (dS–G), the adsorption energy (Eads), the band gap of the adsorption system (Eg), and the charge transfer of the H2S molecule (Q)
System |
dH–S (Å) |
α (°) |
dS–G (Å) |
Eads (eV) |
Eg (eV) |
Q (e) |
H2S/HG |
1.355⋯1.354 |
91.44 |
4.120 |
−0.02 |
3.58 |
0.002 |
H2S/VHG |
1.355⋯1.356 |
91.45 |
3.299 |
−0.05 |
1.66 |
0.007 |
As shown in Table 1, the Eads of H2S/VHG was −0.05 eV, which was larger than that of H2S/HG showing a value of −0.02 eV. The enhancement of adsorption stability suggested that the presence of the H-vacancy improved the sensitivity of graphane to absorb the H2S molecule. The distances between the S atom of the H2S molecule and the graphane surface (HG and VHG) were 3.299 Å and 4.120 Å, respectively. Moreover, the band gaps of the adsorption complexes were obtained. The Eg of HG and VHG was 3.69 eV and 1.70 eV, which changed to 3.58 eV and 1.66 eV, respectively. The weak interaction with the H2S molecule did not induce an obvious change in the band gaps of the graphane substrate. Moreover, we calculated the charge transfer of the H2S molecule to graphane. The Q of H2S/VHG was 0.007 e. The small adsorption energy, large bond distance, and low charge transfer showed that the adsorption of H2S was due to physisorption.
3.3 Adsorption of H2S on FeVHG, CoVHG, and CuVHG
Herein, we investigated the adsorption of H2S on FeVHG, CoVHG, and CuVHG. As stated in Section 3.2, we set the initial adsorption structure with the S atom close to the dopants. After optimization, the adsorption configurations were obtained, as shown in Fig. 4. Furthermore, the corresponding parameters are summarized in Table 2. The adsorption complex of H2S/CoVHG exhibited maximum stability, of which the adsorption energy was −1.50 eV. Compared with the adsorption of H2S on HG and VHG, the Eads was enlarged by the introducing of dopants (Fe, Co, and Cu). After interaction with H2S, the bond lengths of dopant-Cv were elongated such as the dC–Fe changed from 2.224 Å to 2.247 Å. According to the Mulliken analysis results, the Q of H2S/CoVHG was the highest with the value of 0.302 e. In the adsorption process, the electrons transferred from the H2S molecule to the graphane substrate. The large adsorption energy and high charge transfer indicated that the introduction of dopants improved the sensitivity to the H2S molecule and the adsorptions on VHG doped with dopants (Fe, Co, and Cu) were via chemisorption.
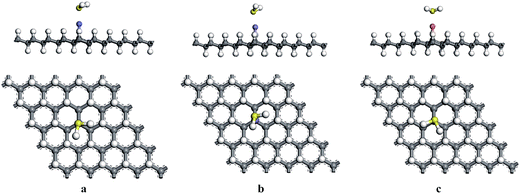 |
| Fig. 4 The optimized structures of (a) H2S/FeVHG, (b) H2S/CoVHG, and (c) H2S/CuVHG. | |
Table 2 Summary of the results for the H2S molecule adsorbed on metal atom doped H-vacancy defected graphane. The properties listed are as follows: the distance between the H and S atom of the H2S molecule (dH–S), the angle of the H2S molecule (α), the distance between the S atom of H2S and metal-dopant (dS-dopant), the distance between the Cv atom and metal-dopant (dC-dopant), the adsorption energy (Eads), the band gap of the adsorption system (Eg), and the charge transfer of the H2S molecule (Q)
System |
dH–S (Å) |
α (°) |
dS-dopant (Å) |
dC-dopant (Å) |
Eads (eV) |
Eg (eV) |
Q (e) |
H2S/FeVHG |
1.370⋯1.371 |
90.45 |
2.247 |
2.047 |
−0.93 |
0.75 |
0.058 |
H2S/CoVHG |
1.369⋯1.371 |
90.36 |
2.221 |
1.995 |
−1.50 |
1.10 |
0.170 |
H2S/CuVHG |
1.363⋯1.363 |
91.71 |
2.220 |
1.966 |
−0.88 |
2.12 |
0.302 |
In our calculated results, the band gaps of FeVHG, CoVHG, and CuVHG were 0.97 eV, 1.26 eV, and 2.33 eV, respectively. The adsorption of the H2S molecule induced a decrease of band gaps. In the case of H2S/FeVHG, the Eg was smaller by about 0.2 eV than that of FeVHG. As is well-known, the change in the Eg of the structures induces the electric conductivity change of graphane according to the following equation:45
where
σ is the electric conductivity of the configurations,
k is the Boltzmann's constant, and
T is the thermodynamic temperature. Therefore, the change of electric conductance of the graphane substrate by the adsorption of the H
2S molecule can be seen as a signal to detect H
2S gas.
3.4 Electronic and magnetic properties
To deeply understand the effect of H-vacancy and dopants on the adsorption of H2S, we performed the spin polarized density of states (PDOS) of adsorption complexes, as shown in Fig. 5–9. As shown in Fig. 5 and 6, the DOS of H2S/HG and H2S/VHG were similar to those of HG and VHG (Fig. 2a and b). From the PDOS of the H2S molecule, we can see that there was very little interaction between the HG (VHG) sheet and the H2S molecule.
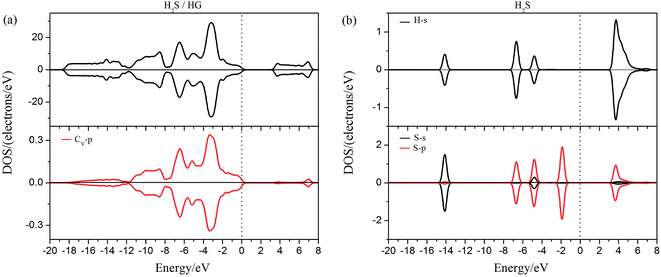 |
| Fig. 5 The PDOS of the (a) H2S/HG complex and (b) H2S molecule. | |
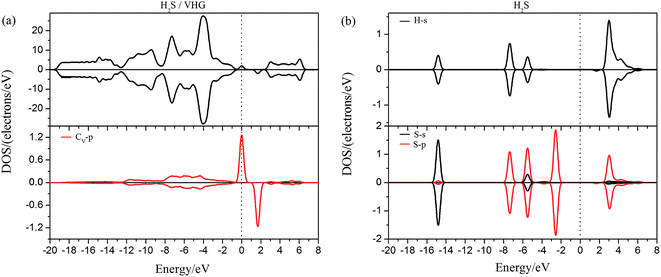 |
| Fig. 6 The PDOS of the (a) H2S/VHG complex and (b) H2S molecule. | |
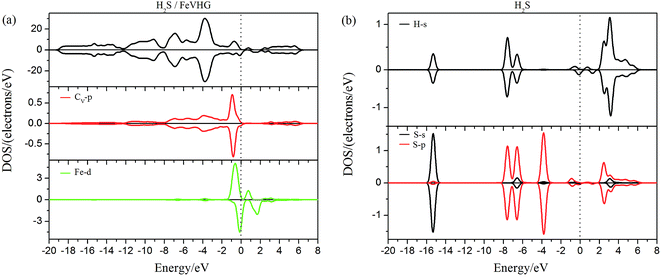 |
| Fig. 7 The PDOS of the (a) H2S/FeVHG complex and (b) H2S molecule. | |
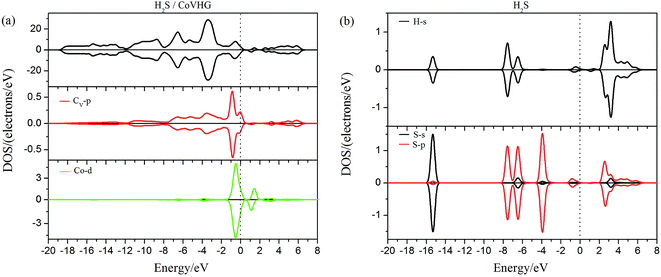 |
| Fig. 8 The PDOS of the (a) H2S/CoVHG complex and (b) H2S molecule. | |
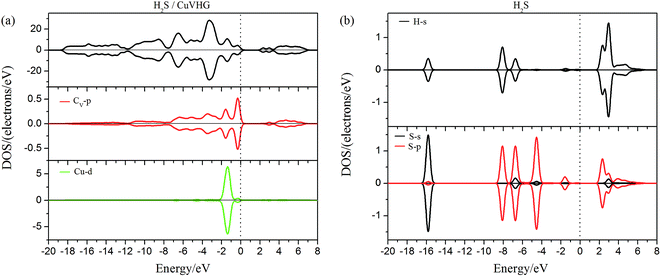 |
| Fig. 9 The PDOS of (a) H2S/CuVHG complex and (b) H2S molecule. | |
In the case of H2S/FeVHG, instead of the two spin-up peaks of the 3d orbital in FeVHG, there was a sharp peak through the Fermi level. Furthermore, we found that a strong hybridization occurred between the Fe-3d and Cv-2p orbitals around the Fermi level, which indicated that a chemical bond formed between the Fe and Cv atoms. For the configuration of H2S/CoVHG, the spin-up and spin-down of the Co-3d orbitals mismatched and induced a magnetic moment, which resulted from the adsorption of H2S. There were overlaps between the DOS of Co-3d and S-2p orbitals, which varied from −1.8 eV to −1.5 eV. The strong hybridization explained the strong affinity of CoVHG towards the H2S gas molecule. As abovementioned, the contribution of the 3d orbital of dopants to the DOS around the Fermi level improved the reactivity of graphane and the strong interaction between the dopants and molecules was responsible for the increase in the adsorption energy.
4. Conclusions
In summary, we explored the adsorption of the H2S molecule on the surface of pure graphane, and H-vacancy-defected and transition-metal-doped graphane. First, we investigated the effect of the H-vacancy and dopants on the geometric structure and electronic properties of graphane. Our results revealed that the states of Cv and dopants were available near the Fermi level and improved the reactivity of the graphane substrate. Furthermore, the Cv atom exhibited a half-metallic behavior. Second, we calculated the adsorption energy, bond length, band gap, and charge transfer for the adsorption systems. The results suggested that the H-vacancy and dopants enhanced the adsorption stability of H2S on the graphane surface. Furthermore, the PDOS results indicated that there was a strong hybridization between the Cv-p orbital and the dopants. Based on these results, it can be concluded that TM-doped graphane is capable of becoming a new sensor for H2S molecules. We believe that our results can provide useful information towards the applications based on graphane.
Acknowledgements
This work was supported by the National Natural Science Foundation of China (NSFC, Grant No. 11604080, 11404096, U1404609, 11304080, 11547153) and the Innovation Team of Henan University of Science and Technology (No. 2015XTD001).
References
- H. Kimura, Physiological Role of Hydrogen Sulfide and Polysulfide in the Central Nervous System, Neurochem. Int., 2013, 63, 492 CrossRef CAS PubMed.
- L. Spadaro, A. Palella, F. Frusteri and F. Arena, Valorization of Crude Bio-Oil to Sustainable Energy Vector for Applications in Cars Powering and on-Board Reformers Via Catalytic Hydrogenation, Int. J. Hydrogen Energy, 2015, 40, 14507–14518 CrossRef CAS.
- P. P. Hankare, S. D. Jadhav, U. B. Sankpal, R. P. Patil, R. Sasikala and I. S. Mulla, Gas Sensing Properties of Magnesium Ferrite Prepared by Co-Precipitation Method, J. Alloys Compd., 2009, 488, 270–272 CrossRef CAS.
- S. Capone, A. Forleo, L. Francioso, R. Rella, P. Siciliano, J. Spadavecchia, D. S. Presicce and A. M. Taurino, Solid State Gas Sensors: State of the Art and Future Activities, ChemInform, 2004, 35, 1335–1348 CrossRef.
- N. Barsan, D. Koziej and U. Weimar, Metal Oxide-Based Gas Sensor Research: How To?, Sens. Actuators, B, 2007, 121, 18–35 CrossRef CAS.
- A. Kolmakov and M. Moskovits, Chemical Sensing and Catalysis by One-Dimensional Metal-Oxide Nanostructures, Annu. Rev. Mater. Res., 2004, 35, 151–180 CrossRef.
- K. S. Novoselov, A. K. Geim, S. V. Morozov, D. Jiang, Y. Zhang, S. V. Dubonos, I. V. Grigorieva and A. A. Firsov, Electric Field Effect in Atomically Thin Carbon Films, Science, 2004, 306, 666 CrossRef CAS PubMed.
- S. Stankovich, D. A. Dikin, G. H. B. Dommett, K. M. Kohlhaas, E. J. Zimney, E. A. Stach, R. D. Piner, S. T. Nguyen and R. S. Ruoff, Graphene-Based Composite Materials, Nature, 2006, 442, 282–286 CrossRef CAS PubMed.
- J. Gu, C. Liang, X. Zhao, B. Gan, H. Qiu, Y. Guo, X. Yang, Q. Zhang and D. Y. Wang, Highly Thermally Conductive Flame-Retardant Epoxy Nanocomposites with Reduced Ignitability and Excellent Electrical Conductivities, Compos. Sci. Technol., 2017, 139, 83–89 CrossRef CAS.
- Q. Zhou, Z. Fu, Y. Tang, H. Zhang and C. Wang, First-Principle Study of the Transition-Metal Adatoms on B-Doped Vacancy-Defected Graphene, Phys. E, 2014, 60, 133–138 CrossRef CAS.
- R. R. Q. Freitas, R. Rivelino, F. d. B. Mota and C. M. C. de Castilho, Dft Studies of the Interactions of a Graphene Layer with Small Water Aggregates, J. Phys. Chem. A, 2011, 115, 12348–12356 CrossRef CAS PubMed.
- J. Sabio, C. Seoánez, S. Fratini, F. Guinea, A. H. C. Neto and F. Sols, Electrostatic Interactions between Graphene Layers and Their Environment, Phys. Rev. B: Condens. Matter Mater. Phys., 2008, 77, 195409 CrossRef.
- B. S. Li, P. W. Yong and K. D. Hai, First-Principle Study of Structural, Electronic, Vibrational and Magnetic Properties of Hcn Adsorbed Graphene Doped with Cr, Mn and Fe, Appl. Surf. Sci., 2015, 329, 330–336 CrossRef.
- A. S. Rad, Al-Doped Graphene as a New Nanostructure Adsorbent for Some Halomethane Compounds: Dft Calculations, Surf. Sci., 2016, 645, 6–12 CrossRef CAS.
- X. Zhang, L. Yu, Y. Gui and W. Hu, First-Principles Study of Sf 6 Decomposed Gas Adsorbed on Au-Decorated Graphene, Appl. Surf. Sci., 2016, 367, 259–269 CrossRef CAS.
- Y. Tang, W. Chen, C. Li, L. Pan, X. Dai and D. Ma, Adsorption Behavior of Co Anchored on Graphene Sheets toward No, So 2, Nh 3, Co and Hcn Molecules, Appl. Surf. Sci., 2015, 342, 191–199 CrossRef CAS.
- Y. Tang, Z. Liu, Z. Shen, W. Chen, D. Ma and X. Dai, Adsorption Sensitivity of Metal Atom Decorated Bilayer Graphene toward Toxic Gas Molecules (CO, NO, SO2 and HCN), Sens. Actuators, B, 2016, 238, 182–195 CrossRef.
- F. Ding, Theoretical Study of the Stability of Defects in Single-Walled Carbon Nanotubes as a Function of Their Distance from the Nanotube End, Phys. Rev. B: Condens. Matter Mater. Phys., 2005, 72, 245409 CrossRef.
- R. M. Ribeiro, N. M. R. Peres, J. Coutinho and P. R. Briddon, Inducing Energy Gaps in Monolayer and Bilayer Graphene: Local Density Approximation Calculations, Phys. Rev. B: Condens. Matter Mater. Phys., 2008, 78, 075442 CrossRef.
- S. C. Pradhan and J. K. Phadikar, Small Scale Effect on Vibration of Embedded Multilayered Graphene Sheets Based on Nonlocal Continuum Models, Phys. Lett. A, 2009, 373, 1062–1069 CrossRef CAS.
- S. Gholami, A. S. Rad, A. Heydarinasab and M. Ardjmand, Adsorption of Adenine on the Surface of Nickel-Decorated Graphene; a DFT Study, J. Alloys Compd., 2016, 686, 662–668 CrossRef CAS.
- M. Kunaseth, T. Mudchimo, S. Namuangruk, N. Kungwan, V. Promarak and S. Jungsuttiwong, A Dft Study of Arsine Adsorption on Palladium Doped Graphene: Effects of Palladium Cluster Size, Appl. Surf. Sci., 2016, 367, 552–558 CrossRef CAS.
- W. Zan, W. Geng, H. Liu and X. Yao, Influence of Interface Structures on the Properties of Molybdenum Disulfide/Graphene Composites: A Density Functional Theory Study, J. Alloys Compd., 2015, 649, 961–967 CrossRef CAS.
- D. C. Elias, R. R. Nair, T. M. G. Mohiuddin, S. V. Morozov, P. Blake, M. P. Halsall, A. C. Ferrari, D. W. Boukhvalov, M. I. Katsnelson and A. K. Geim, Control of Graphene's Properties by Reversible Hydrogenation: Evidence for Graphane, Science, 2009, 323, 610–613 CrossRef CAS PubMed.
- O. Leenaerts, H. Peelaers, A. D. Hernández-Nieves, B. Partoens and F. M. Peeters, First-Principles Investigation of Graphene Fluoride and Graphane, Phys. Rev. B: Condens. Matter Mater. Phys., 2010, 82, 195436 CrossRef.
- H. Şahin, C. Ataca and S. Ciraci, Magnetization of Graphane by Dehydrogenation, Appl. Phys. Lett., 2009, 95, 222510 CrossRef.
- T. Hussain, B. Pathak, M. Ramzan, T. A. Maark and R. Ahuja, Calcium Doped Graphane as a Hydrogen Storage Material, Appl. Phys. Lett., 2012, 100, 302–304 CrossRef.
- S. Haldar, D. G. Kanhere and B. Sanyal, Magnetic Impurities in Graphane with Dehydrogenated Channels, Phys. Rev. B: Condens. Matter Mater. Phys., 2012, 85, 155426 CrossRef.
- T. Hussain, A. D. Sarkar and R. Ahuja, Strain Induced Lithium Functionalized Graphane as a High Capacity Hydrogen Storage Material, Appl. Phys. Lett., 2012, 101, 103907 CrossRef.
- C. Zhou, S. Chen, J. Lou, J. Wang, Q. Yang, C. Liu, D. Huang and T. Zhu, Graphene's Cousin: The Present and Future of Graphane, Nanoscale Res. Lett., 2014, 9, 26 CrossRef PubMed.
- R. E. Mapasha, M. P. Molepo and N. Chetty, Ab Initio Studies of Isolated Hydrogen Vacancies in Graphane, Phys. E, 2016, 79, 52–58 CrossRef CAS.
- M. S. Islam, T. Hussain, G. S. Rao, P. Panigrahi and R. Ahuja, Augmenting the Sensing Aptitude of Hydrogenated Graphene by Crafting with Defects and Dopants, Sens. Actuators, B, 2016, 228, 317–321 CrossRef CAS.
- J. Berashevich and T. Chakraborty, Influence of Adsorbates on the Electronic and Magnetic Properties of Graphane with H-Vacancy Defects, Phys. Rev. B: Condens. Matter Mater. Phys., 2010, 82, 134415 CrossRef.
- C. K. Yang, Graphane with Defect or Transition-Metal Impurity, Carbon, 2010, 48, 3901–3905 CrossRef CAS.
- T. Hussain, P. Panigrahi and R. Ahuja, Enriching Physisorption of H2S and NH3 Gases on a Graphane Sheet by Doping with Li Adatoms, Phys. Chem. Chem. Phys., 2014, 16, 8100–8105 RSC.
- H. Tanveer, P. Puspamitra and A. Rajeev, Sensing Propensity of a Defected
Graphane Sheet Towards CO, H2O and NO2, Nanotechnology, 2014, 25, 325501 CrossRef PubMed.
- B. Delley, From Molecules to Solids with the Dmol3 Approach, J. Chem. Phys., 2000, 113, 7756–7764 CrossRef CAS.
- J. P. Perdew, K. Burke and M. Ernzerhof, Generalized Gradient Approximation Made Simple, Phys. Rev. Lett., 1996, 77, 3865–3868 CrossRef CAS PubMed.
- V. A. Basiuk and L. V. Henaoholguín, Dispersion-Corrected Density Functional Theory Calculations of Meso-Tetraphenylporphine-C60 Complex by Using Dmol3 Module, J. Comput. Theor. Nanosci., 2014, 11, 1609–1615 CrossRef CAS.
- D. J. Chadi, Special Points for Brillouin-Zone Integrations, Phys. Rev. B: Solid State, 1976, 13, 5188–5192 CrossRef.
- B. Delley, Hardness Conserving Semilocal Pseudopotentials, Phys. Rev. B: Condens. Matter Mater. Phys., 2002, 66, 155125 CrossRef.
- J. O. Sofo, A. S. Chaudhari and G. D. Barber, Graphane: A Two-Dimensional Hydrocarbon, Phys. Rev. B: Condens. Matter Mater. Phys., 2007, 75, 153401 CrossRef.
- S. Lebègue, M. Klintenberg, O. Eriksson and M. I. Katsnelson, Accurate Electronic Band Gap of Pure and Functionalized Graphane from Gw Calculations, Phys. Rev. B: Condens. Matter Mater. Phys., 2009, 79, 245117 CrossRef.
- Y. H. Zhang, Y. B. Chen, K. G. Zhou, C. H. Liu, J. Zeng, H. L. Zhang and Y. Peng, Improving Gas Sensing Properties of Graphene by Introducing Dopants and Defects: A First-Principles Study, Nanotechnology, 2009, 20, 185504 CrossRef PubMed.
- S. S. Li, Semiconductor Physical Electronics, Springer, USA, 2nd edn, 2006 Search PubMed.
|
This journal is © The Royal Society of Chemistry 2017 |
Click here to see how this site uses Cookies. View our privacy policy here.