DOI:
10.1039/C7RA05134D
(Paper)
RSC Adv., 2017,
7, 35845-35853
Improved osseointegrating functionality of cell sheets on anatase TiO2 nanoparticle surfaces
Received
7th May 2017
, Accepted 6th July 2017
First published on 19th July 2017
Abstract
Bone marrow mesenchymal stem cell sheets (BMSC sheets) have been reported as a powerful tool for bioengineering applications in accelerating osseointegration. TiO2 nanoparticles are beneficial for osteogenic differentiation of bone marrow mesenchymal stem cells (BMSCs) in vitro. Nonetheless, no studies have reported the effects of anatase TiO2 nanoparticles on the osseointegrating functionality of BMSC sheets in vitro and in vivo. This study is designed to verify the osteogenesis enhancing effects of TiO2 nanoparticle coated implants on BMSC sheets. In this study, we used a novel light-induced cell sheet method to fabricate BMSC–TiO2 nanoparticle–implant complexes. The results showed TiO2 nanoparticles significantly promoted the adhesion and proliferation of cell sheets, and upregulated the expression of osteogenic genes (alkaline phosphatase (ALP), osteocalcin (OCN), Collagen I, bone morphogenetic protein 2 (BMP2), runt-related transcription factor 2 (Runx2) and Osterix) (P < 0.05). In in vivo studies, the results from micro-CT and hard tissue slices showed that the bone-implant contact (BIC) and bone volume per total volume (BV/TV) were significantly higher in the BMSC–TiO2 nanoparticle–implant group compared with the BMSC–implant group (n = 6, P < 0.05). In conclusion, anatase TiO2 nanoparticle surfaces could significantly improve the osseointegrating functionality of BMSC sheets. BMSC–TiO2 nanoparticle–implant complexes are a potential and novel strategy for rapid osseointegration.
1. Introduction
Osseointegration is enhanced by a series of surface modifications, such as grit-blasting/thermo-chemical treatment,1 grit-blasting/acid etching,2 electrodeposits,3 deposition by lasers,4 and microarc oxidation.5 Many studies have investigated the feasibility of applying cell sheets to bone regeneration,6 cardiac regeneration,7 cartilage regeneration,8 tendon healing,9 corneal regeneration,10 esophageal regeneration,11 periodontal regeneration,12 and full thickness skin wound repair.13 Recently, some studies14,15 have reported bone marrow mesenchymal stem cell sheets (BMSC sheets) as a powerful tool for bioengineering applications in accelerating osseointegration.
In addition, previous studies confirmed that micro and nano structures on Ti substrates could enhance the biological responses of BMSCs.16–19 It was reported that specific micro and nano structures with 80 nm grains significantly enhanced osteogenic differentiation of BMSCs compared with 20 nm and 40 nm grains.20 Nevertheless, to the best of our knowledge, no research has been conducted to investigate the effects of TiO2 nanoparticle coated titanium implants on osseointegrating functionality of cell sheets in vitro and in vivo.
We choose BMSCs to investigate because BMSCs are multi-lineage differentiation potential cells that can be induced into osteoblasts, myoblasts, adipocytes, chondrocytes, tenocytes, and neurocytes.21–23 Compared with terminally differentiated cells, BMSCs are immune-tolerated owing to the lack of MHC-II markers and low levels of MHC-I markers.24 Moreover, BMSCs are quite easy to obtain, and culturing method is mature.25 The process of osseointegration around implants includes hemostasis, inflammation, proliferation, and remodeling,26 and BMSCs play a great role in the process of osseointegration. BMSCs migrate to the sites of titanium implants, and differentiate into osteoblasts. During osteogenic differentiation, BMSCs secrete the ECM, and express alkaline phosphatase and osteocalcin, which contribute to the acceleration of osseointegration.26
The aims of this study were to fabricate BMSC–TiO2 nanoparticle–implant complexes by using light-induced cell sheet technology, and to investigate the effects of TiO2 nanoparticle implants on osseointegrating functionality of BMSC sheets in vitro and in vivo.
2. Materials and methods
2.1 Preparation of sandblasted, large-grit, and acid-etched (SLA) titanium disks/implants and TiO2 nanoparticle coated titanium disks/implants
To obtain the SLA titanium disks and implants, disks were prepared from 1 mm thick and 2.5 cm diameter sheets of grade 2 unalloyed Ti, and implants were prepared from 2.2 mm diameter and 6 mm length grade 2 unalloyed Ti (Guangci, Ningbo, Zhejiang, China). The degreased disks and implants were grit-blasted with 0.25–0.50 mm corundum grits and subsequently conducted with double-acid etching. Briefly, titanium disks and implants were immersed in a 0.11 mol L−1 ammonium fluoric acid and 0.09 mol L−1 nitric acid solution (Sigma-Aldrich, St. Louis, MO, USA) at room temperature, and then in a 5.8 mol L−1 hydrochloric acid and 8.96 mol L−1 sulfuric acid solution (Sigma-Aldrich, St. Louis, MO, USA) at 80 °C.
TiO2 nanoparticle coated quartz substrates were prepared on the quartz substrates. TiO2 nanoparticle coated titanium disks were prepared on SLA titanium disks. TiO2 nanoparticle coated implants were prepared on SLA titanium implants. All surfaces were prepared through phase separation-induced self-assembly.27 A precursor sol containing titanium tetrabutoxide (TBOT, Sinopharm Chemical Reagent, CP, >98%), acetylacetone (AcAc, Lingfeng Chemical Reagent, AR, >99%), and polyvinylpyrrolidone (PVP, K30, Sinopharm Chemical Reagent, AR, >99%) was spin-coated on the SLA titanium disks and implants at 8000 rpm for 40 s, and subsequently heated at 500 °C. Scanning electron microscopy (SEM) assay (SU-70, Hitachi, Japan) and energy-dispersive X-ray spectroscopy (EDS) assay were performed to characterize the SLA titanium disks/implants and TiO2 nanoparticle coated titanium disks/implants. To collect the phase composition of the TiO2 nanoparticle coated titanium disks, the X-ray diffractometer (XRD, PANalytical, X'Pert PRO) collected the XRD patterns of the samples. Raman spectra assay was conducted on the TiO2 nanoparticle coated titanium disks by OMNIC software for dispersive Raman (Thermo Fisher Scientific, DXR532) with a 10 mW power at 532 nm.
2.2 Culture of BMSCs and fabrication of BMSC sheets
The Institutional Animal Care and Use Committee (Zhejiang University, Hangzhou, China) approved all animal experiments in this study according to “Experimental Animal Management Ordinance” issued by Chinese science and technology commission and “Laboratory Animal Science Guidance” issued by Chinese science and technology ministry. The method of isolation was reported elsewhere.25 Briefly, three-week-old male Sprague-Dawley (SD) rats were anesthetized. BMSCs were aspirated from the bone marrow of tibias and femurs, and cultured in the basal medium (alpha-modified minimum essential medium (alpha-MEM; Gibco, USA) containing 10% fetal bovine serum (FBS; Gibco, USA), 1% penicillin (Gibco, USA), 1% streptomycin (Gibco, USA), and 0.272 g L−1 L-glutamine (Sigma, USA)).
To fabricate BMSC sheets, 3 × 104 cells per cm2 of BMSCs were seeded on the nanoparticle coated quartz substrates which were coated with 1.2 μg mL−1 laminin-521 (Biolamina, Sundbyberg, Sweden) in a 24-well plate. After culturing for 5 days, BMSC sheets were obtained through illumination under 5.5 mW cm−2 power of UV365 nm for 30 min.28 With light treatment, the surface of TiO2 nanoparticles changed from electropositive to electronegative leading to the releasing of cell sheets.29 The obtained cell sheets were used for subsequent experiments.
2.3 Adhesion and proliferation assay
Alamar Blue assay was conducted to evaluate the adhesion and proliferation of BMSC sheets cultured on SLA titanium disks and TiO2 nanoparticle coated titanium disks. To evaluate the adhesive capacity, after culturing for 1 day, disks were picked up into a new 24-well plate. When we conducted proliferation assay, disks were not picked up. After culturing for 1, 3, and 7 days, the culture media was removed and samples were rinsed 3 times with 1× PBS. Subsequently, 100 μL of Alamar Blue solution and 900 μL of fresh cell culture media were added to each well, and the mixture was incubated at 37 °C for 4 h. Then, the incubation solution was measured by a microplate reader at 540/590 nm. Medium supplemented with 10% Alamar Blue dye was used as a negative control.
2.4 Alkaline phosphatase (ALP) activity assay
To evaluate the effects of TiO2 nanoparticles on osteogenic differentiation of BMSC sheets, we performed ALP activity assay. BMSC sheets were seeded in a 6-well plate on SLA titanium disks and TiO2 nanoparticle coated titanium disks. After undergoing osteogenic differentiation for 7 and 14 days, BMSC sheets were lysed, and then total protein concentrations were determined using a bicinchoninic acid (BCA) kit (Pierce Biotechnology, Inc., USA). ALP activity was measured at 405 nm by p-nitrophenyl phosphate (Wako Pure Chemical Industries, Ltd., Japan) and normalized to the amount of total protein. The relative ALP activity was determined as follows: units per mg protein = concentration of p-nitrophenol released by sample (nmol μL−1)/(15 × protein concentration (μg μL−1)).
2.5 Quantitation of osteocalcin (OCN) using enzyme-linked immunosorbent assay (ELISA)
BMSC sheets were seeded in a 6-well plate on SLA titanium disks and TiO2 nanoparticle coated titanium disks. After culturing in osteogenic medium for 14 and 21 days, the culture medium was collected and centrifuged at 3000 rpm, 4 °C for 10 min, and the concentrations of OCN were measured by ELISA kits (R&D Systems Inc., USA) according to the manufacturer's instructions. OCN concentrations were expressed as ng mL−1 supernatant.
2.6 Reverse transcription and quantitative polymerase chain reaction (RT-qPCR)
Total RNA of BMSC sheets was extracted using TRIzol reagent (Invitrogen, USA). Half of one microgram of total RNA was reverse transcribed in a 10 μL reaction volume using the PrimeScript™ RT Reagent Kit (Perfect Real Time, TaKaRa, Dalian, China) according to the manufacturer's protocol. Real-time PCR was performed in the ABI ViiA™ 7 System (Applied Biosystems, Foster City, CA) by using a SYBR Green kit (TaKaRa, Tokyo, Japan). The primers are shown in Table 1. The relative gene expressions were analyzed using the 2−ΔΔCT method. After normalization to GAPDH, the targeted genes were expressed as fold changes relative to controls.
Table 1 Nucleotide sequences for RT-qPCR primers
Genes |
Sequences of primer (5′–3′) |
ALP |
Forward: TGGTACTCGGACAATGAGATGC |
Reverse: GCTCTTCCAAATGCTGATGAGGT |
Runx2 |
Forward: CAGTATGAGAGTAGGTGTCCCGC |
Reverse: AAGAGGGGTAAGACTGGTCATAGG |
Collagen I |
Forward: CAGATTGAGAACATCCGCAGC |
Reverse: CGGAACCTTCGCTTCCATACTC |
BMP2 |
Forward: ACAAACGAGAAAAGCGTCAAGC |
Reverse: CCCACATCACTGAAGTCCACATA |
Osterix |
Forward: CTGGGAAAAGGAGGCACAAAGA |
Reverse: GGGGAAAGGGTGGGTAGTCATT |
OCN |
Forward: AGGGCAGTAAGGTGGTGAATAGA |
Reverse: GAAGCCAATGTGGTCCGCTA |
GAPDH |
Forward: GGCACAGTCAAGGCTGAGAATG |
Reverse: ATGGTGGTGAAGACGCCAGTA |
2.7 Protein isolation and western blot analysis
Total proteins were extracted using RIPA buffer with phenylmethanesulfonyl fluoride (PMSF, Beyotime, China). Proteins were loaded and separated on Tris–glycine SDS-polyacrylamide gel (Invitrogen, USA), and subsequently transferred onto a polyvinylidene fluoride membrane (Millipore, USA) for immunoblotting. The primary antibodies (rat BMP2, Runx2, Collagen type I (Col-I) (Abcam, USA), and β-actin (Wuhan goodbio technology CO., LTD)) were used to incubate with membranes overnight at 4 °C. Then the peroxidase-conjugated secondary antibody (Wuhan goodbio technology CO., LTD) was used to incubate with the membranes for 1 h at room temperature. The blots were visualized using a chemiluminescent ECL reagent (Millipore, USA). Each target protein was normalized to β-actin.
2.8 Construction of BMSC–implant complexes
After BMSC sheets formed, the sheets detached from the substrates with the illumination of UV 365 nm. The detached BMSC sheets floated in 1 mL 1× PBS in a 24-well plate. We took the substrate away and put a SLA or TiO2 nanoparticle-coated implant under the floating BMSC sheet. Then the implant was picked up gradually with sterilized tweezers, and BMSC sheets attached with the implant. The obtained BMSC–implant complexes were cultured in an incubator for half of an hour with 50 μL basal medium in a 6 cm culture dish. After the cell sheet attached well with the implant, the BMSC–implant complexes were then cultured in basal medium for 7 days.
2.9 Transplantation of BMSC–implant complexes
A total of 24 male SD rats were used for in vivo evaluation. 10% sterile chloral hydrate solution was intra-abdominally injected into the rats at a dose of 3.5 mL kg−1. The implant cavity was prepared by 2.2 mm diameter drills. Two kinds of implants (BMSC–implant complexes, and BMSC–TiO2 nanoparticle–implant complexes, n = 6 per group) were inserted into tibias about 7 mm below the knee joints. Each rat received 1 implant in each tibia.
2.10 Micro-CT scan and hard tissue slices
To prepare specimens, the rat tibias were removed and fixed in a 4% paraformaldehyde solution for 48 h at room temperature. The microtomographical reconstruction was performed with micro-CT (Scanco Medical, Switzerland, μCT-100). The parameter settings were 70 kV, 200 μA at a resolution of 14.8 μm and exposure time of 300 ms. The newly formed bone was restricted to a circle with radius of 0.5 mm surrounding the implants. At a 3-dimensional (3D) level, osseointegration was determined using bone-implant contact (BIC) and bone volume per total volume (BV/TV) by Evaluation v6.5-3 (Scanco Medical, Switzerland).
Following the micro-CT testing, specimens were dehydrated with ethanol solutions and embedded with methyl methacrylate (MMA) to solidify. The specimens were placed in a horizontal position and cut into 4 μm thick sections using Leica SP1600 (Leica, Germany) and processed with toluidine blue and Masson's trichrome staining. The image analysis software Image-Pro Plus (version 6.0; Media Cybernetics; Rockville, MD; USA) was used to evaluate the BIC and BV/TV of implants.
2.11 Statistical analysis
Data were obtained from at least 3 independent experiments and expressed as mean ± standard deviation. Analysis was conducted using the SPSS 17.0 software package by one-way ANOVA with Bonferroni's post-hoc test (multiple comparisons) and unpaired Student's t-test (comparison between two groups). Differences were considered statistically significant at P < 0.05.
3. Results
3.1 Morphology of TiO2 nanoparticle coated films, SLA titanium disks, TiO2 nanoparticle coated titanium disks, SLA titanium implants, and TiO2 nanoparticle coated implants
Fig. 1 illustrated the morphology of SLA surfaces and TiO2 nanoparticle surfaces. The films showed homogeneous distribution of the TiO2 nanoparticles. The diameter of nanoparticles was approximately 80–150 nm. After sandblasting and acid-etching treatment, the surface became rough and irregular, and small holes with micro pits (1–2 μm in diameter) were observed under high magnification. In the holes and micro pits, the TiO2 nanoparticles were clearly observed.
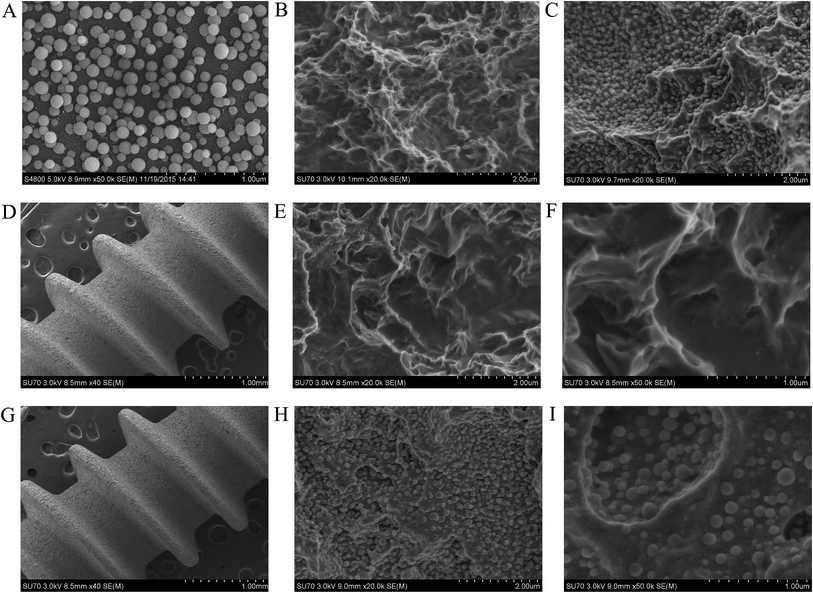 |
| Fig. 1 SEM images of different substrates. (A) TiO2 nanoparticles on quartz substrates, (B) SLA titanium disks, (C) TiO2 nanoparticle coated SLA titanium disks, (D–F) SLA titanium implants, (G–I) TiO2 nanoparticle coated SLA titanium implants. Scale bar: (A, F and I) 1 μm; (B, C, E and H) 2 μm; (D and G) 1 mm. | |
3.2 EDS analysis and crystalline phases of SLA titanium disks and TiO2 nanoparticle coated titanium disks
Traces of oxygen were detected on the SLA surface under EDS analysis (Fig. 2A–G). The mapping of oxygen was consistent with the distribution of TiO2 nanoparticles on the SLA titanium disks. At 500 °C, the peaks at 2θ = 25.35° corresponded to the (101) plane of anatase (Fig. 2H). The anatase phase of TiO2 was also identified through Raman spectra. As shown in Fig. 2I, TiO2 nanoparticle disks showed strong Raman shifts at 144 cm−1, which were ascribed to the Eg mode of the anatase phase (the shift at 144 cm−1 was the strongest for the anatase phase). However, the typical peak of rutile located at 143 cm−1 overlapped with the 144 cm−1 peak of anatase. Another weak Raman shift was at 637 cm−1. Such Raman results were in good consistence with the XRD patterns.
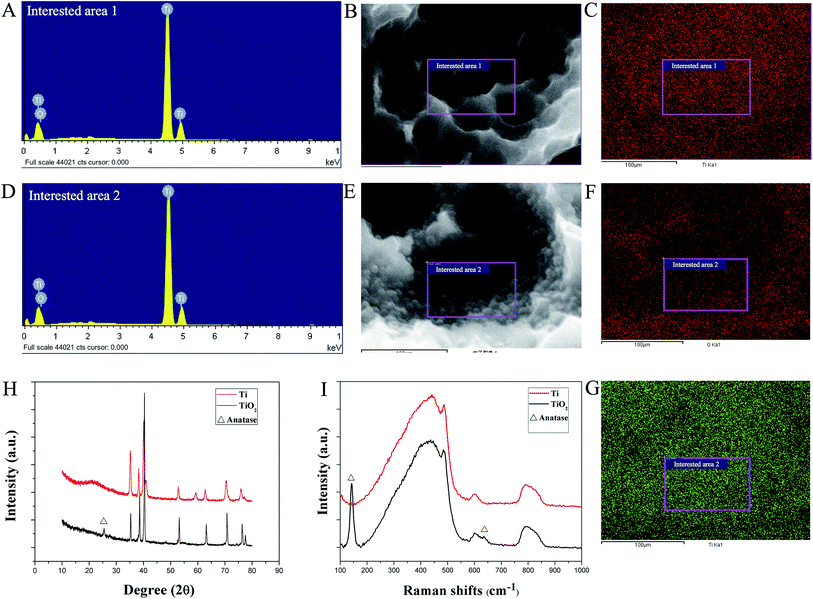 |
| Fig. 2 Characteristics of SLA titanium disks and TiO2 nanoparticle coated SLA titanium disks. (A–F) EDS analysis of SLA titanium disks, and TiO2 nanoparticle coated SLA titanium disks. (C) Mapping of Ti element on SLA titanium disks. (F) Mapping of oxygen element on TiO2 nanoparticle coated SLA titanium disks. (G) Mapping of Ti element on TiO2 nanoparticle coated SLA titanium disks. (H) XRD patterns and (I) Raman spectra of SLA titanium disks and TiO2 nanoparticle coated SLA titanium disks. Δ: peaks of anatase. Scale bar: (B, C and E–G) 100 μm. | |
3.3 Effects of TiO2 nanoparticles on adhesion, proliferation, and osteogenic differentiation of BMSC sheets
The results of adhesion assay showed that TiO2 nanoparticles significantly increased the adhesive capacity of BMSC sheets (Fig. 3A; P < 0.05). The proliferation of BMSC sheets on TiO2 nanoparticle coated titanium disks was elevated on days 5 and 7 (Fig. 3B; P < 0.05). The ALP expression was also significantly increased on days 3 and 7 in the TiO2 nanoparticle group compared with the SLA group (Fig. 3C; P < 0.05), while the expression of OCN protein increased on days 14 and 21 (Fig. 3D; P < 0.05). Fig. 6A–C showed that the mRNA levels of ALP, BMP2, Collagen I, Runx2, and Osterix significantly increased on days 3, 7, and 14 in the TiO2 nanoparticle group, while the mRNA levels of OCN significantly increased on day 14 (P < 0.05). Furthermore, the western blot results revealed that the proteins (Collagen I, BMP2, Runx2, and Osterix) were significantly up-regulated in the TiO2 nanoparticle group on days 3, 7, and 14 (Fig. 4D). The stimulating effects of TiO2 on osteogenic genes indicated that TiO2 nanoparticle coated titanium disks could significantly promote osteogenic differentiation of BMSC sheets in vitro.
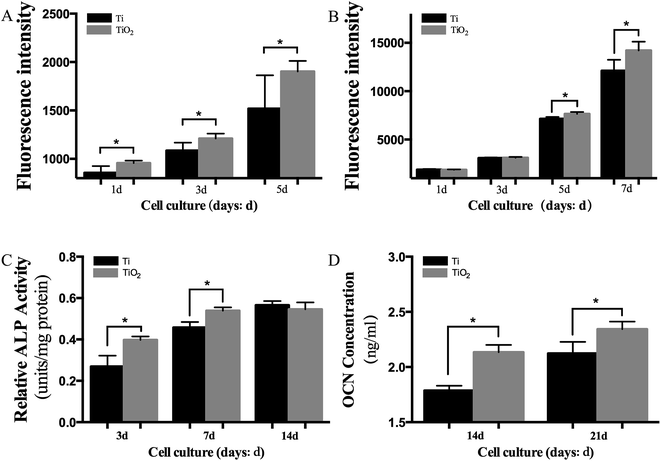 |
| Fig. 3 (A) Adhesion assay on TiO2 nanoparticle coated titanium disks at days 1, 3, and 5. (B) Proliferation of BMSC sheets on TiO2 nanoparticle coated titanium disks measured by Alamar Blue at days 1, 3, 5, and 7. Effects of TiO2 nanoparticle coated titanium disks on (C) relative ALP activity at days 3, 7, and 14, and (D) OCN concentration at days 14, and 21. *P < 0.05. | |
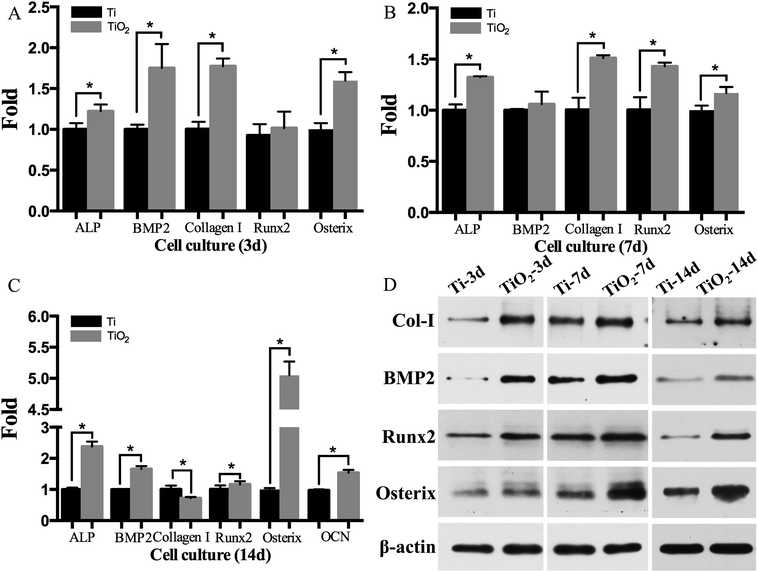 |
| Fig. 4 Relative mRNA expression levels of ALP, BMP2, Collagen I, Runx2, Osterix, and OCN on SLA titanium disks and TiO2 nanoparticle coated SLA titanium disks at (A) day 3, (B) day 7, and (C) day 14 compared with the SLA titanium disk group. (D) Western blot analysis of Col-I, BMP2, Runx2, and Osterix after 3, 7, and 14 days of osteogenic induction. *P < 0.05. | |
3.4 Performance of BMSC–TiO2 nanoparticle–implant complexes
The osseointegration was evaluated by the micro-CT and hard tissue slices after 1 month and 2 months of healing (Fig. 5 and 6). In the BMSC–TiO2 nanoparticle–implant group, more bones contacted with implants than in the BMSC–implant group after 1 month of healing (Fig. 5A, B and 6A). Moreover, more surrounding bones in the BMSC–TiO2 nanoparticle–implant group were discovered compared with the BMSC–implant group after 2 months of healing (Fig. 5C, D and 6B). In the Fig. 5E, F and 6C, D, the BIC and BV/TV values of the BMSC–TiO2 nanoparticle–implant group were higher than BMSC–implant group (P < 0.05).
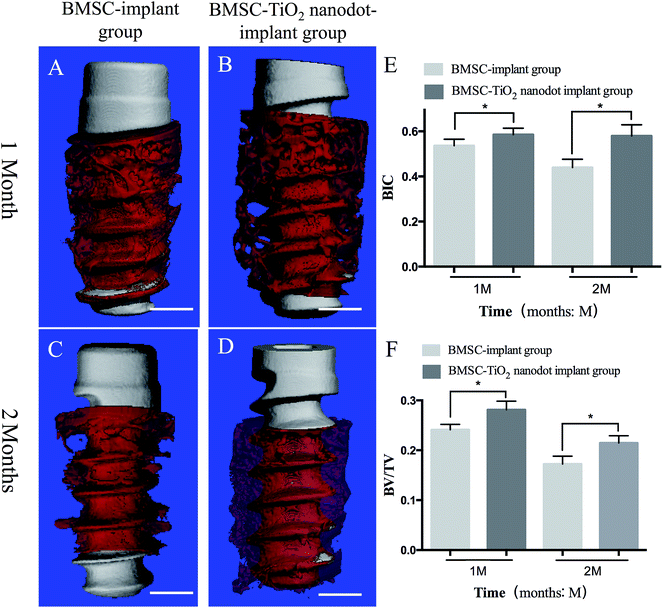 |
| Fig. 5 BMSC–implant complexes and BMSC–TiO2 nanoparticle–implant complexes were measured by micro-CT. The amount of bones surrounding inserted implants was larger in (B and D) the BMSC–TiO2 nanoparticle–implant group than in (A and C) the control group. According to the analysis of micro-CT, BV/TV and BIC of the BMSC–TiO2 nanoparticle–implant group were obviously higher than the BMSC–implant group after 1 month and 2 months of healing. (E) BIC and (F) BV/TV values of the two implant groups. Scale bar: (A–D) 1 mm, *P < 0.05. | |
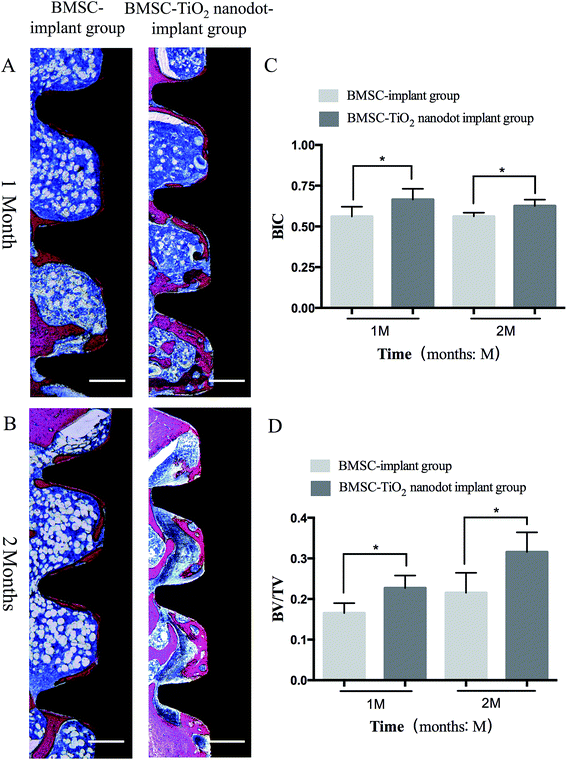 |
| Fig. 6 Hard tissue slices were used to evaluate the osseointegration of BMSC–implants and BMSC–TiO2 nanoparticle–implants ((A) 1 month; (B) 2 months). More contacting bones were observed in the BMSC–TiO2 nanoparticle–implant group than BMSC–implant group after (A) 1 month and (B) 2 months of healing. According to the analysis of hard tissue slices, the (C) BIC and (D) BV/TV of the BMSC–TiO2 nanoparticle–implant group were higher than the BMSC–implant group. Scale bar: 300 μm. *P < 0.05. | |
4. Discussion
In this study, we developed a novel, simple, and effective method to fabricate rat BMSC–TiO2 nanoparticle–implant complexes. According to our data, anatase TiO2 nanoparticle coated surfaces showed enhanced osseointegrating ability, and BMSC–TiO2 nanoparticle–implant complexes provided an alternative novel strategy for potential rapid osseointegration.
In this study, we proved anatase TiO2 nanoparticle coated surfaces effective for promoting osteogenic differentiation of BMSC sheets. There are several reasons for this. Firstly, anatase TiO2 contributed to better osteogenic abilities when compared with the rutile TiO2.30 Secondly, the hierarchical micro/nano-topographies contributed to enhancing osteogenesis. Some studies have documented the effects of micro/nano-topographies on osteogenesis in vitro.16–18 The micro and nanoscale surfaces matched the natural structure of bone, which consisted of hierarchical structures such as nano-structures (including collagen, non-collagenous organic proteins, and mineral crystals) and micro-structures (including haversian systems, osteons, and single trabeculae). Thirdly, micro/nano hierarchical surfaces have great potential for improving spreading and osteogenic differentiation of BMSC sheets. Fourthly, UV-activated TiO2 itself has excellent antibacterial activity. UV-activated TiO2 has been used for killing a wide range of Gram-negative and Gram-positive bacteria, filamentous and unicellular fungi, algae, protozoa, mammalian viruses, and bacteriophage.31,32 However, the toxic kinetics of TiO2 nanoparticles has not yet been investigated. Therefore, since we do not know whether it is safe when used in vivo, the safety of TiO2 nanoparticle coated implants will be measured in our future study.
BMSC sheets have been proven beneficial for cell-based tissue engineering, owing to the ability of multi-lineage potential33 and preserving abundant ECM.34,35 Meanwhile, BMSCs secrete quantities of growth factors effective at promoting osteogenic differentiation, bone matrix synthesis, and mineralization, such as BMP2, ALP, and OCN.36,37 In our study, BMSC–TiO2 nanoparticle–implant groups have elevated expression of BMP2, ALP, and OCN, thus providing a beneficial condition for osseointegration. Moreover, Zhou38 reported that BMSCs could inhibit inflammation through BMSC-derived 15d-PGJ2 activation of the PPAR-γ receptor. Furthermore, Goodwin39 reported that BMSCs could reduce inflammation by influencing antigen-specific CD4 T lymphocyte differentiation. Overall, these factors potentially promote a beneficial biological environment for osseointegration.
We chose the male rat model in this study. We found an interesting phenomenon that tibias became longer when rats grew older. At the same time, distance from the metaphysis to implants became larger. The decreasing BIC and BV/TV could be explained by this phenomenon. Clokie40 compared various bones from SD rats of different weights (i.e., 100 g, 200 g, 300 g, 350 g), and found that the tibial plateau of 350 g rats was a good recipient site. However, the study only evaluated osseointegration at 6 weeks. Yu14 used Wistar rats (200–250 g) to evaluate osseointegration at 4 and 8 weeks. They did not find similar phenomenon. This discrepancy may be due to using different strains of rats.
Furthermore, the molecular mechanisms of the effects of anatase TiO2 nanoparticles on osseointegration were not investigated in this study. In order to fully link the surface modifications to observations, we will evaluate the effects of specific genes on osseointegration through inhibition and overexpression in the future study.
In this study, we successfully constructed BMSC–TiO2 nanoparticle–implant complexes using light-induced cell sheet technology. Our BMSC–TiO2 nanoparticle–implant complexes were a potential and novel strategy for accelerating osseointegration.
5. Conclusions
Within the limits of the present investigation we concluded that anatase TiO2 nanoparticle coated titanium surfaces significantly enhanced osteogenic differentiation of BMSC sheets in vitro and enhanced bone formation in vivo. These findings indicated that BMSC–TiO2 nanoparticle–implant complexes possessed potential for improving biocompatibility of titanium implants.
Conflict of interest
There are no conflicts of interest to declare.
Acknowledgements
This work was supported by the National Natural Science Foundation of China (Grant No. 81272157 and 31470945) and the Health Department of Zhejiang Province Fund (Grant No. 2015KYA147 and 2014KYA127). We thank LetPub (www.letpub.com) for its linguistic assistance during the preparation of this manuscript.
References
- F. J. Gil, D. D. S. P. Eduardo Espinar, D. D. S. P. Jose Maria Llamas and P. Sevilla, Clinical Implant Dentistry and Related Research, 2014, 16, 273–281 CrossRef PubMed.
- D. Yoo, C. Marin, G. Freitas, N. Tovar, E. A. Bonfante, H. S. Teixeira, M. N. Janal and P. G. Coelho, Implant Dent., 2015, 24, 256–262 Search PubMed.
- Z. Shifang, S. Jue, H. Fuming, L. Liu and Y. Guoli, J. Biomed. Nanotechnol., 2014, 10, 1313–1319 CrossRef PubMed.
- F. Sima, P. M. Davidson, J. Dentzer, R. Gadiou, E. Pauthe, O. Gallet, I. N. Mihailescu and K. Anselme, ACS Appl. Mater. Interfaces, 2015, 7, 911–920 CAS.
- R. Zhou, D. Wei, S. Cheng, W. Feng, Q. Du, H. Yang, B. Li, Y. Wang, D. Jia and Y. Zhou, ACS Appl. Mater. Interfaces, 2014, 6, 4797–4811 CAS.
- Q. Xie, Z. Wang, Y. Huang, X. Bi, H. Zhou, M. Lin, Z. Yu, Y. Wang, N. Ni, J. Sun, S. Wu, Z. You, C. Guo, H. Sun, Y. Wang, P. Gu and X. Fan, Biomaterials, 2015, 66, 67–82 CrossRef CAS PubMed.
- K. Matsuura, F. Kodama, K. Sugiyama, T. Shimizu, N. Hagiwara and T. Okano, Tissue Eng., Part C, 2015, 21, 330–338 CrossRef CAS PubMed.
- F. Yano, H. Hojo, S. Ohba, T. Saito, M. Honnami, M. Mochizuki, T. Takato, H. Kawaguchi and U. I. Chung, Biomaterials, 2013, 34, 5581–5587 CrossRef CAS PubMed.
- I. Komatsu, J. H. Wang, K. Iwasaki, T. Shimizu and T. Okano, Acta Biomater., 2016, 42, 136–146 CrossRef CAS PubMed.
- T. Kobayashi, K. Kan, K. Nishida, M. Yamato and T. Okano, Biomaterials, 2013, 34, 9010–9017 CrossRef CAS PubMed.
- N. Kanai, M. Yamato and T. Okano, Ann. Transl. Med., 2014, 2, 28 Search PubMed.
- H. Zhang, S. Liu, B. Zhu, Q. Xu, Y. Ding and Y. Jin, Stem Cell Res. Ther., 2016, 7, 168 CrossRef PubMed.
- L. Chen, Q. Xing, Q. Zhai, M. Tahtinen, F. Zhou, L. Chen, Y. Xu, S. Qi and F. Zhao, Theranostics, 2017, 7, 117–131 CrossRef PubMed.
- M. Yu, W. Zhou, Y. Song, F. Yu, D. Li, S. Na, G. Zou, M. Zhai and C. Xie, Bone, 2011, 49, 387–394 CrossRef CAS PubMed.
- W. Zhou, C. Han, Y. Song, X. Yan, D. Li, Z. Chai, Z. Feng, Y. Dong, L. Li, X. Xie, F. Chen and Y. Zhao, Biomaterials, 2010, 31, 3212–3221 CrossRef CAS PubMed.
- N. Ren, S. Zhang, Y. Li, S. Shen, Q. Niu, Y. Zhao and L. Kong, Br. J. Oral and Maxillofac. Surg., 2014, 52, 907–912 CrossRef PubMed.
- L. Zhao, S. Mei, P. K. Chu, Y. Zhang and Z. Wu, Biomaterials, 2010, 31, 5072–5082 CrossRef CAS PubMed.
- L. Zhao, L. Liu, Z. Wu, Y. Zhang and P. K. Chu, Biomaterials, 2012, 33, 2629–2641 CrossRef CAS PubMed.
- G. Li, H. Cao, W. Zhang, X. Ding, G. Yang, Y. Qiao, X. Liu and X. Jiang, ACS Appl. Mater. Interfaces, 2016, 8, 3840–3852 CAS.
- X. Shen, P. Ma, Y. Hu, G. Xu, J. Zhou and K. Cai, Colloids Surf., B, 2015, 127, 221–232 CrossRef CAS PubMed.
- G. Kaur, M. T. Valarmathi, J. D. Potts, E. Jabbari, T. Sabo-Attwood and Q. Wang, Biomaterials, 2010, 31, 1732–1741 CrossRef CAS PubMed.
- P. Sreejit, K. B. Dilip and R. S. Verma, Cell Tissue Res., 2012, 350, 55–68 CrossRef CAS PubMed.
- Y. Jiang, B. N. Jahagirdar, R. L. Reinhardt, R. E. Schwartz, C. D. Keene, X. R. Ortiz-Gonzalez, M. Reyes, T. Lenvik, T. Lund, M. Blackstad, J. Du, S. Aldrich, A. Lisberg, W. C. Low, D. A. Largaespada and C. M. Verfaillie, Nature, 2002, 418, 41–49 CrossRef CAS PubMed.
- K. Thejaswi, M. Amarnath, G. Srinivas, M. K. Jerald, T. A. Raj and S. Singh, Cell Tissue Transplant. Ther., 2012, 4, 1–13 CAS.
- D. P. Lennon and A. I. Caplan, Exp. Hematol., 2006, 34, 1606–1607 CrossRef CAS PubMed.
- R. Tejero, E. Anitua and G. Orive, Prog. Polym. Sci., 2014, 39, 1406–1447 CrossRef CAS.
- K. Zhu, N. R. Neale, A. Miedaner and A. J. Frank, Nano Lett., 2007, 7, 69–74 CrossRef CAS PubMed.
- Z. Jiang, Y. Xi, K. Lai, Y. Wang, H. Wang and G. Yang, BioMed Res. Int., 2017, 2017, 9474573 Search PubMed.
- Y. Hong, M. F. Yu, W. J. Weng, K. Cheng, H. M. Wang and J. Lin, Biomaterials, 2013, 34, 11–18 CrossRef CAS PubMed.
- L. Lv, K. Li, Y. Xie, Y. Cao and X. Zheng, Mater. Sci. Eng., C, 2017, 78, 96–104 CrossRef CAS PubMed.
- H. A. Foster, I. B. Ditta, S. Varghese and A. Steele, Appl. Microbiol. Biotechnol., 2011, 90, 1847–1868 CrossRef CAS PubMed.
- J. C. Yu, W. Ho, J. Lin, H. Yip and P. K. Wong, Environ. Sci. Technol., 2003, 37, 2296–2301 CrossRef CAS PubMed.
- F. Wei, C. Qu, T. Song, G. Ding, Z. Fan, D. Liu, Y. Liu, C. Zhang, S. Shi and S. Wang, J. Cell. Physiol., 2012, 227, 3216–3224 CrossRef CAS PubMed.
- J. Chen, D. Zhang, Q. Li, D. Yang, Z. Fan, D. Ma and L. Ren, Tissue Cell, 2016, 48, 442–451 CrossRef CAS PubMed.
- J. Ren, P. Jin, M. Sabatino, A. Balakumaran, J. Feng, S. A. Kuznetsov, H. G. Klein, P. G. Robey and D. F. Stroncek, Cytotherapy, 2011, 13, 661–674 CrossRef CAS PubMed.
- Y. Liu, L. Ming, H. Luo, W. Liu, Y. Zhang, H. Liu and Y. Jin, Biomaterials, 2013, 34, 9998–10006 CrossRef CAS PubMed.
- J. Yan, C. Zhang, Y. Zhao, C. Cao, K. Wu, L. Zhao and Y. Zhang, Biomaterials, 2014, 35, 7734–7749 CrossRef CAS PubMed.
- J. Zhou, L. Jiang, X. Long, C. Fu, X. Wang, X. Wu, Z. Liu, F. Zhu, J. Shi and S. Li, J. Cell. Mol. Med., 2016, 20, 1706–1717 CrossRef CAS PubMed.
- M. Goodwin, V. Sueblinvong, P. Eisenhauer, N. P. Ziats, L. Leclair, M. E. Poynter, C. Steele, M. Rincon and D. J. Weiss, Stem Cells, 2011, 29, 1137–1148 CrossRef CAS PubMed.
- C. M. Clokie and H. Warshawsky, Compendium-continuing Education for Veterinarians, 1995, vol. 16 Search PubMed.
|
This journal is © The Royal Society of Chemistry 2017 |
Click here to see how this site uses Cookies. View our privacy policy here.