DOI:
10.1039/C7RA05945K
(Paper)
RSC Adv., 2017,
7, 35917-35927
Formulation of water-in-oil-in-water (W/O/W) emulsions containing trans-resveratrol
Received
27th May 2017
, Accepted 30th June 2017
First published on 19th July 2017
Abstract
Resveratrol is a high-value bioactive polyphenolic compound with vast applications in functional foods; as such, effective and scalable delivery strategies for this compound are worthy of study. In this research, W/O/W emulsions were successfully prepared using polyglycerol polyricinoleate (PGPR) as a lipophilic emulsifier and Tween 80 as a hydrophilic emulsifier with the goal of developing biocompatible carriers to improve the bioavailability of resveratrol. The effects of the type of emulsifier, the concentration of emulsifier, the ratio of the oil phase to the internal water phase, and homogeneous pressure on the physical properties of the W/O/W emulsions (such as microstructure, droplet size, distribution, zeta potential, viscosity and encapsulation efficiency) were investigated. The optimum processing conditions for preparing W/O/W emulsions are as follows: the ratio of the oil phase to the internal water phase is 80
:
20, the concentrations of lipophilic and hydrophilic emulsifiers are 10 wt% and 5 wt%, and the homogeneous pressures in the first and second steps are 30 MPa and 10 MPa. The optimal preparation process of the W/O/W emulsions was used to encapsulate resveratrol. The results showed that at up to 0.040 wt% in the internal water phase (ethanol), resveratrol could be successfully encapsulated in W/O/W emulsions with an encapsulation efficiency of 99.97 ± 0.001%. Moreover, resveratrol was successfully encapsulated in the internal water phase and oil phase together; thus, it was not necessary to increase the amount of carrier materials. This study provides a novel encapsulation formulation to increase the delivery efficacy of resveratrol.
1 Introduction
Resveratrol (3,5,4′-trans-trihydroxystilbene), a natural polyphenol mainly found in a wide range of plants (grapes, red wine, peanuts, etc.), is one of the most highly studied polyphenolic compounds. Several studies have found that resveratrol has various biological activities, including anti-inflammatory, cardioprotective, antioxidant and anticancer activities.1–3 However, because its solubility is limited and it is degraded in light, the applications of trans-resveratrol are limited.4,5
Currently, numerous methods are being studied to overcome the above problems; of these, encapsulation is the most promising. The main strategy of encapsulation is to entrap a core material within a wall material to assist in the delivery of an active agent to living cells.6–8 This technology is also beneficial to extend the shelf life of the product, reduce evaporation and degradation, prevent intermolecular interaction, improve sensory characteristics, control the release of bioactive compounds and, finally, enhance the bioavailability of the compounds.7,9,10 At present, there are numerous physical, chemical, and biological methods to realize product encapsulation, including spray drying, coacervation, inclusion, extrusion, liposomes, co-crystallization, emulsion, fluid bed coating, and nano-encapsulation.4,6,11,12 Risch13 used the extrusion technique to encapsulate flavor substances. This process starts by forming a low moisture (5% to 10%) carbohydrate melt (110 °C to 130 °C). The melt is composed of a low dextrose equivalent (DE) maltodextrin, a simple sugar and possibly a modified food starch. An emulsifier is added to the melt, and then the flavoring material is added with vigorous agitation. Moreover, they found that the product of this process contains 8–20% flavor load and is exceptionally stable to deterioration by oxidation. Qiaomei et al.,14 on the other hand, successfully prepared oil-in-water (O/W) sub-micrometer emulsions stabilized by ι-carrageenan and β-lactoglobulin to encapsulate epigallocatechin-3-gallate (EGCG). They found that 50 μg mL−1 free EGCG was able to inhibit cell proliferation. However, the EGCG sub-micrometer emulsion showed stronger anticancer effects at concentrations of 25 and 50 μg mL−1. This result suggested that EGCG sub-micrometer emulsion may enhance the bioactivities of EGCG. Shi et al.15 encapsulated resveratrol in yeast cells for the first time. They compared the DPPH radical-scavenging capacities of yeast-encapsulated resveratrol and non-encapsulated resveratrol. They found that the DPPH radical-scavenging activity of resveratrol increased after encapsulation. Moreover, the yeast-encapsulated resveratrol exhibited good stability, and its bioavailability was enhanced as a result of the increased solubility of resveratrol and its controlled release. Among the methods for encapsulation, emulsion technology has attracted much attention because it involves simple processing, low energy cost, and easy application; moreover, it is widely used in food, medical, pharmaceutical, and cosmetological products and separation processes.16
Recently, a series of more complex and structured emulsions have been developed. One of these is double emulsions, also called multiple emulsions, which consist of two types: water-in-oil-in-water (W1/O/W2) emulsions and oil-in-water-in-oil (O1/W/O2) emulsions,17 mainly depending on the loaded active agents. Hemar et al.5 used an aqueous solution of MilliQ water containing 0.1 M NaCl and 20 wt% ethanol as the internal water phase to prepare resveratrol W/O/W emulsions to increase the solubility of resveratrol. Aditya et al.18 prepared W/O/W emulsions to encapsulate catechin and curcumin. Catechin was encapsulated in an internal water phase that consisted of gelatin, ascorbic acid, NaCl and Milli-Q water. Moreover, curcumin was encapsulated in the oil phase, which consisted of olive oil and the lipophilic emulsifier PGPR. Compared with normal emulsions, double emulsions have two oil-water interfaces, which can provide better protection to bioactive compounds and increase their resistance to degradation by the external phase.19,20 For poorly soluble materials such as curcumin, W1/O/W2 emulsions are more suitable, as the internal water phase can optionally change the solvent conditions to meet the requirement of high solubility while the internal oil phase can provide a natural barrier to outside influences, such as light, oxygen and ions, with excellent texture properties. In addition, W1/O/W2 emulsions can be used to replace fats while retaining similar physicochemical and sensory properties to full-fat products, such as appearance, flavor, mouthfeel and texture.16,21 Liu et al.22 successfully produced a W/O/W emulsion as a carrier for butterfat that when used in the manufacture of cheese provided similar physical properties to full-fat cheese with 30% less fat. Although W1/O/W2 emulsions have been used in the encapsulation of these active agents, their applications for resveratrol are rare. Despite the health applications of resveratrol, research is limited on encapsulations of resveratrol that combine both an oil phase and an internal water phase.
The objective of this research was to establish a stable W/O/W emulsion system to encapsulate trans-resveratrol using high pressure homogenization (HPM). The encapsulation efficiency (EE) of the W/O/W emulsions was analyzed and the emulsions were characterized, including their microstructures, zeta potentials, viscosities, droplet sizes and distributions.
2 Materials and methods
2.1 Materials
trans-Resveratrol, absolute ethanol, Tween 80, chitosan, mannose, methanol and HPLC-grade acetonitrile were purchased from Sigma-Aldrich (USA). Lecithin and pectin was supplied by Aladdin (USA). Miglyol 812 and glyceryl monostearate (GMS) were obtained from Sasol GmbH (Germany). Whey protein isolate (WPI) with a purity of 92% was purchased from Davisco Foods International (USA). Polyglycerol polyricinoleate (PGPR) and Span 80 were obtained from Merck (Germany) and Tokyo Chemical Industry (Japan), respectively. Modified starch was supplied by the Anglo-Dutch company Unilever. Peanut protein isolate (PPI) with a purity of 90% was extracted from peanut protein powder in our lab.23 Peanut protein-mannose mixture (PPM) and peanut protein-mannose Maillard reaction products (MRPs) were prepared according to Yang's method24 with slight modifications. Water purified by a Milli-Q system was used for sample preparation.
2.2 Preparation of W/O/W emulsions
W/O/W emulsions were prepared using the two-step emulsification method.9 The internal and external water phases consisted of Milli-Q water. The oil phases contained the lipophilic emulsifiers. The preparation process is summarized in Fig. 1.1 Primary water-in-oil (W/O) emulsions were prepared by mixing the internal water phase, lipophilic emulsifier and oil phase, allowing the mixture to stir at 25
000 rpm using an Ultra-Turrax dispersing instrument (T10, IKA, Germany) for 3 min. Finally, the mixture was homogenized (Nano Homogenize Machine, ATS Engineering, US) for 4 passes.2 W/O/W emulsions were produced by adding the previous W/O emulsions (20 wt%) to the external water phase (80 wt%), allowing the mixture to stir at 10
000 rpm for 2 min followed by homogenization for 3 passes. In this step, a hydrophilic emulsifier was used to stabilize the oil-water interface.
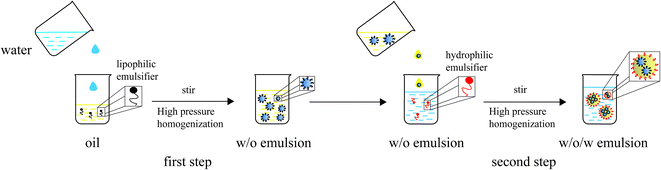 |
| Fig. 1 The preparation process of the W/O/W emulsions. | |
2.2.1 W/O/W emulsions with different lipophilic and hydrophilic emulsifiers. In the single factor experiment with lipophilic emulsifier, W/O/W emulsions were prepared by fixing the hydrophilic emulsifier (Tween 80) at 5 wt% and the ratios of oil phase to internal water phase (O
:
W1) and external water phase to W/O emulsion phase (W2
:
W/O) at 80
:
20 (w/w); the oil phase contained 5 wt% of different lipophilic emulsifiers (PGPR, Span 80, GMS and lecithin). In the single factor experiment with hydrophilic emulsifier, W/O/W emulsions were prepared by fixing the lipophilic emulsifier (PGPR, 5 wt%), leaving the other parameters unchanged, and changing the type of hydrophilic emulsifier (PPI, modified starch, Tween 80, chitosan, PPM, MRPs, pectin, WPI).
2.2.2 W/O/W emulsions with different O
:
W1 ratios. W/O/W emulsions were prepared by fixing the ratio of W2
:
W/O at 80
:
20, the oil phase content of PGPR at 5 wt%, the external water phase content of Tween 80 at 5 wt%, and the first and second step pressures at 30 MPa and 10 MPa, respectively; the ratio of O
:
W1 was varied from 90
:
10 to 50
:
50, w/w.
2.2.3 W/O/W emulsions with different concentrations of emulsifier. W/O/W emulsions were prepared by fixing the ratios of O
:
W1 and W2
:
W/O at 80
:
20 (w/w) and the first and second step pressures at 30 MPa and 10 MPa, respectively. The concentrations of lipophilic (PGPR) and hydrophilic emulsifiers (Tween 80) were varied from 1 wt% to 10 wt%.
2.2.4 W/O/W emulsions with different homogenization pressures. W/O/W emulsions were prepared by fixing the ratios of O
:
W1 and W2
:
W/O at 80
:
20 (w/w), the oil phase content of PGPR at 10 wt%, and the external water phase content of Tween 80 at 5 wt%. The pressure of the first step was varied from 65 MPa to 5 MPa and that of the second step was varied from 0 MPa to 20 MPa.
2.3 Characterization analysis of W/O/W emulsions
2.3.1 Microstructure observations. Freshly prepared W/O/W emulsions were analyzed using an optical microscope (Primo Star, Carl Zeiss, Germany) at room temperature. The photo micrograph images of the emulsions were acquired using a microscope digital camera (Moticam 2306) equipped with vision imaging software (Motic Images Plus 2.0). Samples were placed on microscopic slides and then carefully covered with a cover slip to minimize destruction of the emulsion structures. The microstructures of the W/O/W emulsions were observed using an oil immersion objective (100× magnification), and an appropriate light intensity was selected to reduce sample heating. For each sample, at least three images were taken, and a representative image is shown.Cryo-SEM was conducted to observe the cross-sectional and interfacial structures of the W/O/W emulsions. It was carried out with a scanning electron microscope equipped with liquid nitrogen-cooled sample preparation and transfer units (Quanta PP3010T).
A drop of the sample was placed on the specimen holder and immersed in liquid nitrogen. The sample was then transferred to the sample preparation unit of the cryo-SEM, where it was maintained at 160 °C and a pressure of 10−6 mbar. Once fractured with a blade, the sample was subjected to sublimation at −90 °C for 20 min and then sputter-coated with a layer of Au. Finally, the sample was inserted into the observation chamber equipped with an SEM cold stage module maintained at −140 °C.
2.3.2 Droplet size and distribution. The droplet sizes and distributions of the W/O/W emulsions were determined using dynamic light scattering using a Mastersizer 2000 (Malvern Instruments, Worcestershire, UK) with a measuring range of 20 nm to 2000 μm. Some optical parameters were adjusted as follows: the refractive index of the dispersed phase was 1.466; the refractive index of the continuous phase was 1.333. Absorption was set at 0.01. Average sizes are reported as D3,2.The distribution was expressed in terms of span,4,25 defined as
where
D(
v, 0.1),
D(
v, 0.5) and
D(
v, 0.9) are standard percentile readings from the analysis.
D(
v, 0.1) and
D(
v, 0.9) are the sizes of the droplets lying below 10% and 90%, respectively, of the sample.
D(
v, 0.5) is the median droplet size, which is stated above as the diameter where half of the size lies below this value.
2.3.3 Zeta potential. The zeta potentials of the W/O/W emulsions were determined using a zeta potential analyzer (Zetasizer Nano, UK). The samples were diluted with Milli-Q water prior to analysis; the ratio of sample to water was 0.0309
:
1. In this process, the samples were added slowly to avoid air bubbles. Each sample was analyzed at least in triplicate.
2.3.4 Viscosity. Viscosity measurements were performed with a Discovery DHR-2 instrument (TA Instruments, Surrey, UK) with a cone-plate geometry 40 mm in diameter and a cone angle of 2°. Flow curves were determined at an increasing shear rate from 1 to 100 s−1 in 120 s at room temperature. The instrument was required to be calibrated after each measurement was completed. Two replicas were used for each measurement.
2.3.5 Encapsulation efficiency (EE). The encapsulation efficiency of resveratrol during production was expressed as the amount of resveratrol in the internal water phase, which accounts for the initial ratio of resveratrol in the entire system. Moreover, the resveratrol was determined as described previously using a HPLC method with minor modifications.26 In brief, 4.5 mL of the resveratrol W/O/W emulsion was placed in a centrifugation tube and centrifuged at 20
000 × g for 4 min at 4 °C. The external water phase at the bottom of the centrifugation tube was collected using a syringe and passed through a 0.45 μm syringe filter to collect only water and exclude oil droplets. The collected samples were stored at −80 °C until analysis using the HPLC system (Agilent Technologies, Palo Alto, CA, USA).In the experiment, the column used for the separation was a reversed phase C18 column with 5 μm particle size, 4.6 mm × 150 mm (Agilent, USA). The mobile phase was a mixture of (A) 100% Milli-Q water and (B) 100% acetonitrile. The injection volume was 10 μL with a flow rate of 0.8 mL min−1. A wavelength of 306 nm was used.
2.3.6 Statistical analysis. Analysis of variation (ANOVA) was used to compare the experimental data statistically. The statistical analysis was performed using SPSS software (SPSS Statistics 17). The data were obtained from at least two experiment replications. Moreover, the level of significance was set at p < 0.05.
3 Results and discussion
3.1 Effects of operation parameters on the formation of W/O/W emulsions
3.1.1 Lipophilic emulsifiers. W/O/W emulsions were prepared using different lipophilic emulsifiers at a concentration of 5 wt%. Various characteristics of the W/O/W emulsions were measured.
Distribution. As can be seen from Table 1, there was a significant difference in the distribution of W/O/W emulsions. The GMS W/O/W emulsions had the largest span value of 1.655. Furthermore, the spans of the other W/O/W emulsions ranged from 1.160 to 1.269. This result showed that the GMS W/O/W emulsions have higher polydispersity indices than other W/O/W emulsions. It can be observed in Fig. 2B that the droplet size distribution of the GMS W/O/W emulsions presented unimodal distribution with a wide peak. W/O/W emulsions prepared with PGPR, Span 80, and lecithin presented unimodal distributions with a narrow peak. This finding is consistent with reports by other authors on double emulsions using PGPR as a lipophilic emulsifier.16
Table 1 Effects of lipophilic emulsifiers on droplet size, distribution, viscosity and zeta potentiala
Lipophilic emulsifier |
D3,2 (μm) |
Span |
Viscosity (Pa s) |
Zeta potential (mV) |
Values of the droplet size, span, viscosity and zeta potential. Values in a column followed by different lowercase letters in superscripts were significantly different from each other according to Duncan's double range test (p < 0.05). Data measured at room temperature. |
GMS |
1.739 ± 0.023a |
1.655 ± 0.254a |
0.0056 ± 0.0000b |
−11.800 ± 1.556c |
PGPR |
1.584 ± 0.020b |
1.269 ± 0.153ab |
0.0054 ± 0.0001a |
−33.150 ± 0.778a |
Lecithin |
1.579 ± 0.006b |
1.160 ± 0.091b |
0.0054 ± 0.0001a |
−21.983 ± 0.118b |
Span 80 |
1.544 ± 0.034b |
1.168 ± 0.002b |
0.0053 ± 0.0000a |
−13.633 ± 0.047c |
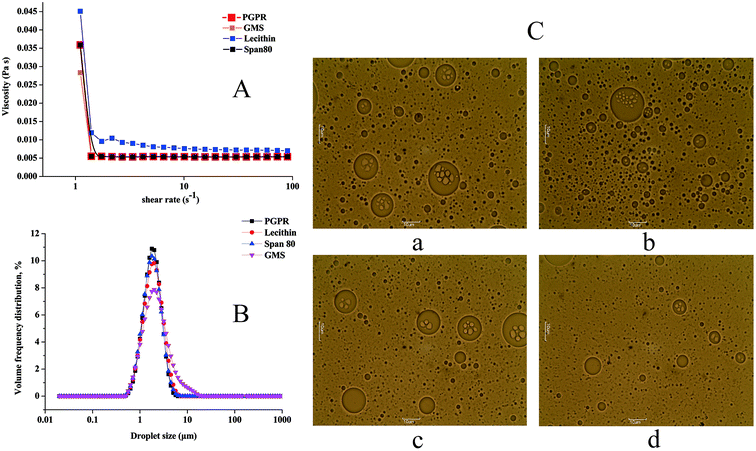 |
| Fig. 2 The properties of W/O/W emulsions prepared with different lipophilic emulsifiers. (A): Viscosity of W/O/W emulsions; (B): distribution of W/O/W emulsions; (C): optical micro-structure of W/O/W emulsions. The scale bars are 10 μm. (a) PGPR W/O/W emulsions; (b) lecithin W/O/W emulsions; (c) Span 80 W/O/W emulsions; (d) GMS W/O/W emulsions. | |
Size. The mean droplet sizes of the different W/O/W emulsions are shown in Table 1. The droplet sizes of the GMS W/O/W emulsions, PGPR W/O/W emulsions, lecithin W/O/W emulsions and Span 80 emulsions are 1.739 μm, 1.584 μm, 1.579 μm, and 1.544 μm, respectively. Tamnak27 reported that the mean size of W/O/W emulsions prepared with PGPR ranged from 3 to 4 μm, which is different from the values observed in this experiment. This may be due to the fact that in their experiment, pectin-pea protein isolate conjugate, which has a higher molecular weight than Tween 80, was used as the hydrophilic emulsifier.
Microscopic images. This characterization shows the compartmented structures of the W/O/W emulsions, which consist of relatively large oil droplets with some smaller water droplets inside. The oil droplets were generally larger in W/O/W emulsion/PGPR (Fig. 2C(a)) and W/O/W emulsion/Span 80 (Fig. 2C(c)) than in W/O/W emulsion/lecithin (Fig. 2C(b)) and W/O/W emulsion/GMS (Fig. 2C(d)). The PGPR W/O/W emulsions contain larger water droplets; as a result, the droplet sizes of the PGPR W/O/W emulsions may be larger than those of the other emulsions.
Viscosity. It is known that small droplet sizes can be obtained by improving the viscosity of the continuous phase in emulsions.28 As can be seen from Table 1, the viscosity of the W/O/W emulsions with PGPR as lipophilic emulsifier is 0.0054 Pa s, while that of the W/O/W emulsions with GMS is 0.0056 Pa s. In addition, the viscosities of the Span 80 W/O/W emulsions and lecithin W/O/W emulsions are 0.0053 Pa s and 0.0054 Pa s, respectively. The viscosities of the emulsions are similar because of the low concentration of W/O emulsions in the external water phase. As can be seen from Fig. 2A, all the emulsions display pseudo-plastic behavior with increasing shear rate. As the shear rate is increased, the viscosities of the different W/O/W emulsions decreased at first and then stabilized at a certain value. As reported by Wang Jing,29 double emulsions are non-Newtonian fluids due to their complex rheological behaviors. They are more dilute under a higher shear than under a lower shear, which is similar to the so-called shear thinning.
Zeta potential. In this study, the zeta potentials of all the prepared emulsions were negative, indicating that the emulsion droplets were negatively charged. Table 1 shows that the zeta potential values in all cases ranged from −11.800 mV to −33.150 mV. Zeta potentials greater than 30 mV or less than −30 mV can stabilize these double emulsions.27 The results revealed that the emulsions stabilized with PGPR had higher stability than the other emulsions.In general, lecithin and GMS have poor water solubility; it is necessary to provide external energy (such as heating and ultrasound) to dissolve these emulsifiers in the preparation of W/O/W emulsions. Stratification was observed when the lecithin and GMS W/O/W emulsions were maintained at room temperature for a period of time. There was no significant difference in the droplet sizes of the PGPR W/O/W emulsions and Span 80 W/O/W emulsions. The former was 1.584 μm and the latter was 1.544 μm. However, the microstructures of the PGPR W/O/W emulsions showed larger water droplets inside (Fig. 2C(a)). In addition, there was a significant difference between the zeta potential values of the PGPR W/O/W emulsions and the Span 80 W/O/W emulsions. The former had the largest absolute value (−33.150 mV).
The above results show that PGPR is the best lipophilic emulsifier. However, our results are more in line with similar W/O/W emulsions reported in other studies. Silvestre de los Reyes and Charcosset30 compared the emulsifying effects of different emulsifiers using PGPR and MO-750 as lipophilic emulsifiers to prepare water-in-oil and ethanol-in-oil emulsions. Water droplet sedimentation occurred (to a small degree) in the W/O emulsions after 1 month of storage at room temperature. They found that using PGPR provided good stabilizing properties to the emulsions.
3.1.2 Hydrophilic emulsifiers. The type of hydrophilic emulsifier also affects the properties of W/O/W emulsions. To study this, W/O/W emulsions were prepared using different hydrophilic emulsifiers, including modified starch, PPI, Tween 80, pectin, chitosan, WPI, PPM and MRPs. Similarly, the microstructure, zeta potential, viscosity, droplet size and distribution were measured.
Distribution. Usually, emulsions will become unstable when the droplet size is larger and the size distribution is wider owing to higher rates of creaming and Ostwald ripening.31 Table 2 reveals that there was a significant difference in the distributions of the W/O/W emulsions. The chitosan W/O/W emulsions had the largest span value (3.647). The span values of the MRPs, PPT, pectin, PPM, Tween 80, modified starch and WPI W/O/W emulsions were 2.020, 2.105, 1.474, 2.791, 1.269, 1.325 and 1.953, respectively. As can be seen from Fig. 3A, when Tween 80 and modified starch were used as hydrophilic emulsifiers, they showed monomodal and symmetric droplet distributions with narrow peaks. W/O/W emulsions stabilized by pectin, PPI, chitosan, WPI, PPM and MRPs were very polydisperse; some of the emulsions prepared from pectin, PPM, PPI and WPI had two peaks. These results reveal that the chitosan, MRPs, PPT, pectin, and PPM W/O/W emulsions are unstable because these emulsions have larger droplet sizes and wider distributions than the Tween 80, modified starch and WPI W/O/W emulsions.
Table 2 Effects of hydrophilic emulsifiers on droplet size, distribution, viscosity and zeta potentiala
Hydrophilic emulsifier |
D3,2 (μm) |
Span |
Viscosity (Pa s) |
Zeta potential (mV) |
Values of the droplet size, span, viscosity and zeta potential. Values in a column followed by different lowercase letters in superscripts were significantly different from each other according to Duncan's double range test (p < 0.05). Data measured at room temperature. |
Chitosan |
10.649 ± 0.776a |
3.647 ± 0.225a |
0.0121 ± 0.0033c |
8.207 ± 1.400f |
MRPs |
6.830 ± 0.701b |
2.020 ± 0.108c |
0.0842 ± 0.0309b |
−37.983 ± 1.06b |
PPI |
6.038 ± 0.275bc |
2.105 ± 0.085c |
0.0246 ± 0.0183bc |
−45.150 ± 0.966a |
Pectin |
5.652 ± 0.093c |
1.474 ± 0.233d |
1.1786 ± 0.0647a |
−22.567 ± 1.839d |
PPM |
4.173 ± 0.273d |
2.791 ± 0.247b |
0.0074 ± 0.0003c |
−44.267 ± 2.169a |
Tween 80 |
1.584 ± 0.020e |
1.269 ± 0.153d |
0.0054 ± 0.0001c |
−33.150 ± 0.778c |
Modified starch |
1.804 ± 0.026e |
1.325 ± 0.012d |
0.0074 ± 0.0001c |
−12.783 ± 0.259e |
WPI |
1.605 ± 0.026e |
1.953 ± 0.247c |
0.0056 ± 0.0000c |
−40.817 ± 0.542b |
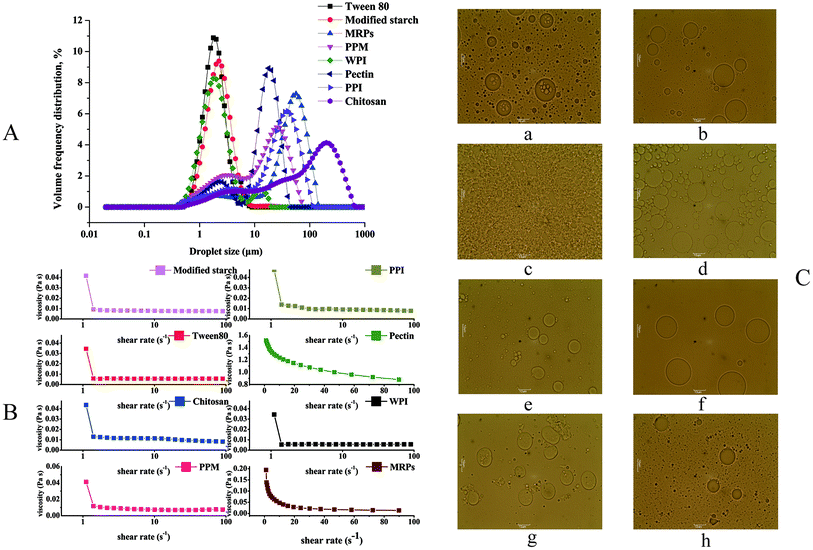 |
| Fig. 3 The properties of W/O/W emulsions prepared with different hydrophilic emulsifiers. (A): Distribution of W/O/W emulsions; (B): viscosity of W/O/W emulsions; (C): optical micro-structure of W/O/W emulsions. The scale bars are 10 μm. (a) Tween 80 W/O/W emulsions; (b) WPI W/O/W emulsions; (c) PPI W/O/W emulsions; (d) PPM W/O/W emulsions; (e) MRPs W/O/W emulsions; (f) pectin W/O/W emulsions; (g) chitosan W/O/W emulsions; (h) modified starch W/O/W emulsions. | |
Size. As can be seen from Table 2, there was a significant difference in the droplet sizes of W/O/W emulsions with different hydrophilic emulsifiers. Chitosan W/O/W emulsions have the largest droplet size of 10.649 μm. The droplet sizes of MRPs, PPI, pectin, PPM, Tween 80, modified starch and WPI were 6.830 μm, 6.038 μm, 5.652 μm, 4.173 μm, 1.584 μm, 1.804 μm and 1.605 μm, respectively. Moreover, there were no significant differences in the droplet sizes of the Tween 80, WPI and modified starch W/O/W emulsions. Mun et al.32 reported that the mean size of W/O/W emulsions prepared with different concentrations of PGPR and WPI ranged from 3.3 to 9.9 μm, similar to what is reported in this experiment. Matos et al.4 determined the droplet sizes of W/O/W emulsions prepared with PGPR as a lipophilic emulsifier and Tween 80 as a hydrophilic emulsifier; however, their results differed from those obtained in this experiment. They found that the distributions of W/O/W emulsions have two evident peaks. This may be due to the fact that their concentration of hydrophilic emulsifier was different. In their experiment, the concentration of Tween 80 used (2% w/v) was lower, which may have resulted in droplets with higher surface tension.
Microscopic images. Microscopic images of the different emulsions are shown in Fig. 3C. The modified starch (Fig. 3C(h)), pectin (Fig. 3C(f)), Tween 80 (Fig. 3C(a)), WPI (Fig. 3C(b)) and chitosan (Fig. 3C(g)) emulsions have the characteristics of W/O/W emulsions. The PPM (Fig. 3C(d)), PPI (Fig. 3C(c)) and MRPs (Fig. 3C(e)) emulsions have no significant features of W/O/W emulsions.
Viscosity. The viscosities of these emulsions were also measured. Table 2 reveals that there were significant differences in the viscosities of the W/O/W emulsions prepared with different hydrophilic emulsifiers (p < 0.05). The viscosity of the W/O/W emulsions with pectin as the lipophilic emulsifier was 1.1786 Pa s, while that of the W/O/W emulsions with MRPs was 0.0842 Pa s. Moreover, the viscosities of the W/O/W emulsions with PPI, chitosan, modified starch, PPM, Tween 80 and WPI were 0.0246 Pa s, 0.0121 Pa s, 0.0074 Pa s, 0.0074 Pa s, 0.0054 Pa s and 0.0056 Pa s, respectively. As can be seen from Fig. 3B, the viscosity of the pectin W/O/W emulsions decreases with increasing shear rate. In addition, the viscosities of the other W/O/W emulsions did not undergo any significant changes with increasing shear rate. These emulsions can therefore be classified as Newtonian fluids.
Zeta potential. The zeta potential measurements of all W/O/W emulsions were negative, with the exception of the chitosan emulsions. As can be seen from Table 2, there was a significant difference in the zeta potentials of the different emulsions (p < 0.05). As previously described, a zeta potential over 30 mV or less than −30 mV can stabilize double emulsions. The zeta potentials of the PPI, PPM, WPI, MRPs and Tween 80 W/O/W emulsions were all less than −30 mV. Thus, these W/O/W emulsions can be classified as stable systems.In general, stratification was observed when the PPM, PPI, MRPs and chitosan W/O/W emulsions were maintained at room temperature for a period of time. This is because PPI, PPM and chitosan are difficult to dissolve in water. In addition, from the microscopic images of the emulsions, we can barely discern the structures of the W/O/W emulsions. This may be attributed to the fact that these emulsifiers only dispersed in the water phase and did not dissolve in the water phase. Therefore, W/O/W emulsions prepared with these emulsifiers will settle after a period of time. The viscosity of the pectin W/O/W emulsions is higher; however, these emulsions have a wider droplet size and distribution. In addition, from the microstructures (Fig. 3C(f)), we can see that the pectin W/O/W emulsions contain only a small drop of water. This may be due to the fact that pectin has thickening properties, and hence it absorbs a portion of the internal water phase. Therefore, PPM, PPI, MRPs and pectin are not suitable for use as hydrophilic emulsifiers.
There were no significant differences in the droplet sizes and viscosities of the Tween 80, WPI and modified starch W/O/W emulsions. However, the WPI W/O/W emulsions had two peaks. This shows that the WPI W/O/W emulsions are unstable. As previously described, a zeta potential greater than 30 mV or less than −30 mV can stabilize these double emulsions. Thus, the Tween 80 W/O/W emulsions are expected to have good stability.
3.1.3 O
:
W1 ratio. In this study, we used different O
:
W1 ratios to determine its effects on the droplet sizes of W/O/W emulsions. As can be seen from Table 3, it was found that there were significant differences in the droplet sizes of W/O/W emulsions prepared with different O
:
W1 ratios. This table shows that increasing the O
:
W1 ratio increases the droplet size significantly (p < 0.05).
Table 3 Effects of different O
:
W1 ratios on droplet size, distribution, viscosity and zeta potentiala
Oil/water |
D3,2 (μm) |
Span |
Viscosity |
Zeta potential |
Values of the droplet size, span, viscosity and zeta potential. Values in a column followed by different lowercase letters in superscripts were significantly different from each other according to Duncan's double range test (p < 0.05). Data measured at room temperature. |
90 : 10 |
1.341 ± 0.156bc |
1.088 ± 0.067ab |
0.0055 ± 0.0001a |
−25.017 ± 0.823a |
80 : 20 |
1.584 ± 0.020a |
1.269 ± 0.153a |
0.0054 ± 0.0001a |
−33.150 ± 0.778b |
70 : 30 |
1.459 ± 0.040ab |
1.081 ± 0.002ab |
0.0054 ± 0.0001a |
−26.633 ± 0.849ab |
60 : 40 |
1.219 ± 0.077cd |
0.942 ± 0.015b |
0.0054 ± 0.0002a |
−28.566 ± 2.357ab |
50 : 50 |
1.090 ± 0.043d |
1.101 ± 0.006ab |
0.0052 ± 0.0001a |
−28.417 ± 2.003ab |
As observed from the microstructures of the emulsions (Fig. 4A), the oil phase contains smaller water droplets at an O
:
W1 ratio of 90
:
10 (Fig. 4A(a)). As the internal water phase increases, the oil droplets imbibe larger water droplets, which results in an increase in the droplet sizes of W/O/W emulsions. A possible reason for this observation may be that when the volume of the internal water phase increases (e.g. as the O
:
W1 ratio is varied from 90
:
10 to 80
:
20), larger W/O emulsions are formed. Furthermore, as the internal water phase increases, forming the W/O/W emulsions becomes challenging because there is less emulsifier by proportion, which causes higher surface tension of the droplets. Thus, the W/O emulsions will be unstable. When adding the W/O emulsions to external water phase, it is possible that the internal and external water phases will come together. With homogenization, a large amount of O/W emulsion is formed rather than the desired W/O/W emulsions; this causes a reduction in the droplet sizes of the W/O/W emulsions. From this study, an O
:
W1 ratio of 80
:
20 is a suitable operation parameter for the preparation of W/O/W emulsions.
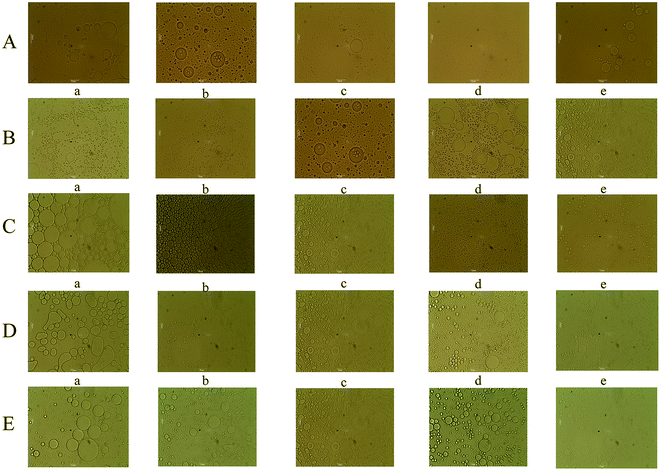 |
| Fig. 4 Optical micro-structures of W/O/W emulsions. The scale bars are 10 μm. (A): O : W1 ratios varied from 90 : 10 to 50 : 50. (a) 90 : 10; (b) 80 : 20; (c) 70 : 30; (d) 60 : 40; (e) 50 : 50. (B): Concentration of Tween 80 fixed at 5 wt%, concentration of PGPR varied from 1 wt% to 10 wt%. (a) PGPR 1 wt%; (b) PGPR 2 wt%; (c) PGPR 5 wt%; (d) PGPR 8 wt%; (e) PGPR 10 wt%. (C): Amount of PGPR 80 fixed at 10 wt%, concentration of Tween 80 varied from 1 wt% to 10 wt%. (a) Tween 80 1 wt%; (b) Tween 80 2 wt%; (c) Tween 80 5 wt%; (d) Tween 80 8 wt%; (e) Tween 80 10 wt%. D: Second step pressure varied from 0 MPa to 20 MPa with the first pressure fixed at 30 MPa. (a) 0 MPa; (b) 5 MPa; (c) 10 MPa; (d) 15 MPa; (e) 20 MPa. E: First step pressure varied from 65 MPa to 5 MPa with the second step pressure fixed at 10 MPa. (a) 5 MPa; (b) 15 MPa; (c) 30 MPa; (d) 50 MPa; (e) 65 MPa. | |
3.1.4 The concentrations of lipophilic and hydrophilic emulsifiers. Lipophilic and hydrophilic emulsifiers play very important roles in the formation of W/O/W emulsions. Table 4 shows that increasing the concentration of lipophilic emulsifier increases the droplet size significantly (p < 0.05). This can be attributed to the fact that when the concentration of the lipophilic emulsifier increases, the surface tension of the droplets decreases, which results in the formation of more W/O emulsions; moreover, W/O/W emulsions will be formed when W/O emulsions are mixed with an external water phase because the internal water phase cannot be combined with the external water phase. Mun et al.32 compared the microstructures of emulsions prepared with different concentrations of PGPR (4, 6, and 8 wt%). They observed that some aggregated water droplets could be found in the W/O emulsions with the addition of 4 wt% PGPR. As the concentration of PGPR was increased, the aggregated water droplets in the microstructure disappeared. This indicates that the W/O emulsion microstructure stability increased with increasing PGPR. Muschiolik et al.33 reported that the droplet size of W/O emulsions increased with decreasing PGPR content; however, the PGPR content showed no effect on the oil droplet size of the W/O/W emulsions. This may be due to the fact that increasing the PGPR content resulted in the formation of more and larger W/O emulsions. Furthermore, according to Fig. 4B, with increasing PGPR, more W/O/W emulsions were formed. These results indicate that 10 wt% PGPR is more suitable for stabilizing the W/O emulsion droplets which contain 20 wt% internal water phase.
Table 4 Effects of different concentrations of lipophilic and hydrophilic emulsifiers on droplet sizea
Concentration (wt%) |
D3,2 (μm) |
Tween 80 1 |
Tween 80 2 |
Tween 80 5 |
Tween 80 8 |
Tween 80 10 |
Values of the droplet size. Values in a column followed by different lowercase letters (a–c) in superscripts were significantly different from each other according to Duncan's double range test (p < 0.05). Also, values in a row followed by different lowercase letters (m–o) in superscripts were significantly different from each other according to Duncan's double range test (p < 0.05). Data measured at room temperature. |
PGPR 1 |
1.573 ± 0.099bm |
1.472 ± 0.020bm |
1.472 ± 0.000bm |
1.416 ± 0.055bm |
1.212 ± 0.063cn |
PGPR 2 |
1.567 ± 0.030bm |
1.562 ± 0.016abmn |
1.554 ± 0.001amn |
1.459 ± 0.074abn |
1.310 ± 0.031bo |
PGPR 5 |
1.621 ± 0.004abm |
1.606 ± 0.030am |
1.584 ± 0.020amn |
1.542 ± 0.018abno |
1.508 ± 0.014ao |
PGPR 8 |
1.698 ± 0.038abm |
1.650 ± 0.079amn |
1.598 ± 0.022amno |
1.565 ± 0.016ano |
1.532 ± 0.018ao |
PGPR 10 |
1.721 ± 0.045am |
1.660 ± 0.044amn |
1.598 ± 0.001ano |
1.569 ± 0.052ano |
1.547 ± 0.031ao |
As can be seen from Table 4, the size of the W/O/W emulsions is quite significantly affected (p < 0.05) by increasing the hydrophilic emulsifier concentration. The droplet size showed a decreasing trend when the concentration of hydrophilic emulsifier (Tween 80) was increased from 1 wt% to 10 wt%. Mun et al.32 prepared W/O/W emulsions with different concentrations of hydrophilic emulsifier (WPI); they found that the droplet size decreased when the concentration of WPI was increased. The same observations were made in this study. From the microstructures of the emulsions (shown in Fig. 4C), it is difficult to observe the effects on the W/O/W emulsions when the concentration of hydrophilic emulsifier is increased. This may be due to the fact that when the concentration of lipophilic emulsifier is fixed and the concentration of hydrophilic emulsifier is increased, more micelles are formed, which will lead to rupture of the oil film and facilitate the release of internal water droplets.30 These results showed that 5 wt% was the best concentration of hydrophilic emulsifier.
3.1.5 HPM pressures during the first and second steps. In general, the mean droplet sizes of emulsions decrease with increasing emulsifying pressure due to the higher energy density. As can be seen from Table 5, the droplet sizes decreased from 12.188 μm to 0.927 μm, 10.895 μm to 0.823 μm, 13.862 μm to 0.958 μm, 12.694 μm to 0.873 μm, and 13.669 μm to 0.927 μm when the pressure during the first step was decreased from 65 MPa to 5 MPa and the pressure during the second step was decreased from 0 MPa to 20 MPa.
Table 5 Effects of HPM pressure during the first and second steps on droplet sizea
Pressure (MPa) |
D3,2 (μm) |
65 |
50 |
30 |
15 |
5 |
Values of the droplet size. Values in a column followed by different lowercase letters (a–e) in superscripts were significantly different from each other according to Duncan's double range test (p < 0.05). Values in a row followed by different lowercase letters (m–o) in superscripts were significantly different from each other according to Duncan's double range test (p < 0.05). Data measured at room temperature. |
0 MPa |
12.188 ± 0.143amn |
10.895 ± 1.041an |
13.862 ± 0.887am |
12.694 ± 0.563amn |
13.669 ± 0.692am |
5 MPa |
2.034 ± 0.090bm |
2.049 ± 0.054bm |
2.057 ± 0.013bm |
2.011 ± 0.026bm |
1.978 ± 0.040bm |
10 MPa |
1.618 ± 0.059cm |
1.577 ± 0.029bm |
1.598 ± 0.001bcm |
1.603 ± 0.036bm |
1.630 ± 0.047bcm |
15 MPa |
1.219 ± 0.002dn |
1.192 ± 0.030bn |
1.169 ± 0.043bcn |
0.792 ± 0.010co |
1.323 ± 0.035bcm |
20 MPa |
0.927 ± 0.044emn |
0.823 ± 0.005bn |
0.958 ± 0.044cm |
0.873 ± 0.077cmn |
0.927 ± 0.030cmn |
The microstructures of W/O/W emulsions produced using different pressures are shown in Fig. 4D. When the second step pressure is 0 MPa (Fig. 4D(a)), the system is unstable and droplets will occur due to aggregation. With increasing second step pressure, increasing numbers of W/O/W emulsions can be observed in Fig. 4D(c). However, as can be seen from Fig. 4D(d) and (e), with increasing second step pressure, fewer W/O/W emulsions can be observed. Therefore, a pressure of 10 MPa is more suitable for the second step.
One possible reason is that increasing the second step pressure will lead to damage of the W/O emulsions. This promotes the fusion of the internal water phase and the external water phase; as a result, the majority of the entire system consists of O/W emulsions, with only a small fraction of W/O/W emulsions. Jeonghee et al.34 reported that the mean droplet sizes of the W/O/W emulsions decreased with increasing homogenization pressure and number of passes.
Table 5 shows the details of the different W/O/W emulsions when the second step pressure was fixed and the first step pressure was varied from 65 MPa to 5 MPa. This result reveals that the droplet size of the W/O/W emulsions has no obvious trend with changing first step pressure from 65 MPa to 5 MPa. As can be seen from Fig. 4E, the W/O/W emulsions contain more water droplets when the first step pressure is 30 MPa (Fig. 4E(c)) than when it is 5 MPa (Fig. 4E(a)) or 15 MPa (Fig. 4E(b)). This may be because when the first step pressure is 5 MPa or 15 MPa, it is too difficult to mix the oil phase and internal water phase because the pressure in the first step is too low. Also, it is difficult to form stable W/O emulsions. Thus, it is difficult to observe W/O/W emulsions under an optical microscope. When the first step pressure was increased, we also could not observe the W/O/W emulsions (Fig. 4E(d) and (e)). This may be due to the fact that the surface area of the dispersed phase increases sharply, which means the emulsifier cannot be adsorbed on the surfaces of all the droplets when the first step pressure is high. Thus, the droplets form unstable W/O emulsions. The second step is homogeneous; thus, the internal water phase and the external water phase will come together and the system will eventually form O/W emulsions. Therefore, 30 MPa is a more suitable pressure for the first step.
3.2 Encapsulation efficiency (EE) of W/O/W emulsions
EE was observed for resveratrol (Fig. 5). In the case of co-loaded resveratrol in the internal water phase and oil phase, no significant difference in the EE of resveratrol was observed in comparison with the W/O/W emulsions where resveratrol was only present in the internal water phase when the internal water phase was ethanol. However, when the internal water phase was Milli-Q water, the EE of resveratrol was 94.97 ± 0.298%, which is lower than when the internal water phase was ethanol (99.97 ± 0.001%). This may be because resveratrol is much more soluble in ethanol than in Milli-Q water. Thus, the resveratrol present in the external water phase is negligible.
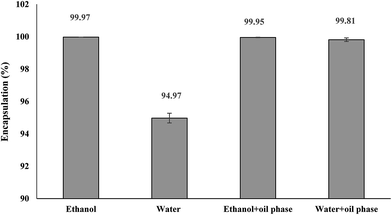 |
| Fig. 5 Encapsulation efficiencies of W/O/W emulsions stabilized with the lipophilic stabilizer PGPR and the hydrophilic stabilizer Tween 80. Ethanol: the internal water phase is absolute ethanol, and resveratrol is only present in absolute ethanol; water: the internal water phase is Milli-Q water, and resveratrol is only present in the internal water phase; ethanol + oil phase: the internal water phase is absolute ethanol, and resveratrol is present in the absolute ethanol and oil phases; water + oil phase: the internal water phase is Milli-Q water, and resveratrol is present in the Milli-Q water and oil phases. | |
Another important observation is total loading efficiency, which can be described as the total amount of resveratrol that remained entrapped in a known amount of the emulsion sample.35 The amount of resveratrol in the W/O/W emulsions prepared with Milli-Q water as the internal water phase was 0.000052 wt%. However, the amount of resveratrol in the W/O/W emulsions prepared with absolute ethanol as the internal water phase was 0.040 wt%, which was significantly higher than that when Milli-Q water was used as the internal water phase. Furthermore, when resveratrol is encapsulated in the oil phase and internal water phase (Milli-Q water) together, the proportion of resveratrol to the whole system is 0.016 wt%. If the internal water phase is ethanol, the proportion of resveratrol to the whole system is 0.056 wt%. This result shows that more resveratrol can be delivered by encapsulation without increasing the carrier materials.
Mun et al.32 determined the EEs of different W/O/W emulsions prepared with different pressures. They found that all emulsions showed good EE (over >90%), which is lower than this experiment. Aditya et al.35 prepared W/O/W emulsions to encapsulate catechin. They found that the EE of catechin was 97 ± 0.3%. This EE is lower than that reported in this study.
4 Conclusions
In summary, a simple and effective method has been developed to prepare resveratrol W/O/W emulsions. This study demonstrates the successful preparation of resveratrol W/O/W emulsions using PGPR as a lipophilic emulsifier and Tween 80 as a hydrophilic emulsifier. By analyzing the microstructures, droplet sizes, zeta potentials and viscosities of the W/O/W emulsions, we obtained the optimum process conditions for preparing W/O/W emulsions (O
:
W1 is 80
:
20, the concentration of lipophilic and hydrophilic emulsifiers are 10 wt% and 5 wt%, and the homogeneous pressures in the first and second steps are 30 MPa and 10 MPa). The EE of resveratrol W/O/W emulsions prepared by the optimum process was 99.97 ± 0.001% (internal water phase is ethanol). Furthermore, in this study, resveratrol was encapsulated in an internal water phase and oil phase together without the need to increase the carrier materials.
Compared with delivery systems such as liposomes (EE = 97.36 ± 2.00%)36 and lipid nanoparticles (EE = 70%),37 our double emulsion system has a higher EE (99.97 ± 0.001%). A possible reason is that W/O/W emulsions have two oil-water interface films, which provide better protection to the resveratrol core. In addition to the EE, the W/O/W emulsion systems have other advantages that strengthen their potential use in food and health products. First, W/O/W emulsion systems can be used to encapsulate bioactive compounds such as flavor or nutrient compounds and deliver them at a controlled rate during eating and digestion. Second, they can be used to prepare reduced-fat products. However, although the W/O/W emulsion in this study has a high EE, the absorption and transport mechanism is not well understood. Follow-up studies should therefore focus on elucidating the absorption and transport mechanisms of the W/O/W emulsion under in vivo and in vitro conditions. This knowledge is important to understand how W/O/W-encapsulated compounds will interact with other components in food matrices, thereby extending their application in food systems beyond meat products,38 cheese products,39 emulsion-based desserts (e.g. ice cream), and functional food beverage systems.40
Author contributions
Jun Wang collected test data, interpreted results, and drafted the manuscript. Qiang Wang and Ai-min Shi designed the study and helped to draft the manuscript. Dominic Agyei also helped to draft the manuscript.
Acknowledgements
This study was supported by the National Key Research and Development Plan (2016YFD0400205) and Science and Technology Innovation Project of the Chinese Academy of Agricultural Sciences (CAAS-ASTIP-201X-IAPPST).
References
- W. Yu, Y. C. Fu and W. Wang, J. Cell. Biochem., 2012, 113, 752–759 CrossRef CAS PubMed.
- L. G. Carter, J. A. D'Orazio and K. J. Pearson, Endocr.-Relat. Cancer, 2014, 21, R209–R225 CrossRef CAS PubMed.
- D. Delmas, V. Aires, E. Limagne, P. Dutartre, F. Mazue, F. Ghiringhelli and N. Latruffe, Ann. N. Y. Acad. Sci., 2011, 1215, 48–59 CrossRef CAS PubMed.
- M. Matos, G. Gutiérrez, J. Coca and C. Pazos, Colloids Surf., A, 2014, 442, 69–79 CrossRef CAS.
- Y. Hemar, L. J. Cheng, C. M. Oliver, L. Sanguansri and M. Augustin, Food Biophys., 2010, 5, 120–127 CrossRef.
- V. Nedovic, A. Kalusevic, V. Manojlovic, S. Levic and B. Bugarski, Procedia Food Sci., 2011, 1, 1806–1815 CrossRef CAS.
- G. B. Celli, A. Ghanem and M. S.-L. Brooks, Food Bioprocess Technol., 2015, 8, 1825–1837 CrossRef CAS.
- N. Prichapan and U. Klinkesorn, Songklanakarin J. Sci. Technol., 2014, 36, 651–661 Search PubMed.
- J. O'Regan and D. M. Mulvihill, Food Res. Int., 2010, 43, 224–231 CrossRef.
- A. Luca, B. Cilek, V. Hasirci, S. Sahin and G. Sumnu, Food Bioprocess Technol., 2013, 7, 204–211 CrossRef.
- Z. Fang and B. Bhandari, Trends Food Sci. Technol., 2010, 21, 510–523 CrossRef CAS.
- A. Munin and F. Edwards-Levy, Pharmaceutics, 2011, 3, 793–829 CrossRef CAS PubMed.
- S. J. Risch, Encapsulation of Flavors by Extrusion, Flavors Encapsulation, 1988, vol. 370, pp. 103–109 Search PubMed.
- Q. Ru, H. Yu and Q. Huang, J. Agric. Food Chem., 2010, 58, 10373–10381 CrossRef CAS PubMed.
- G. Shi, L. Rao, H. Yu, H. Xiang, H. Yang and R. Ji, Int. J. Pharm., 2008, 349, 83–93 CrossRef CAS PubMed.
- R. Bou, S. Cofrades and F. Jiménez-Colmenero, Innovative Food Sci. Emerging Technol., 2014, 23, 1–9 CrossRef CAS.
- H. Lamba, K. Sathish and L. Sabikhi, Food Bioprocess Technol., 2015, 8, 709–728 CrossRef CAS.
- N. P. Aditya, S. Aditya, H.-J. Yang, H. W. Kim, S. O. Park, J. Lee and S. Ko, J. Funct. Foods, 2015, 15, 35–43 CrossRef CAS.
- D. J. McClements, E. A. Decker and J. Weiss, J. Food Sci., 2007, 72, R109–R124 CrossRef CAS PubMed.
- M. Kanouni, H. L. Rosano and N. Naouli, Adv. Colloid Interface Sci., 2002, 99, 229–254 CrossRef CAS PubMed.
- D. J. McClements, E. A. Decker, Y. Park and J. Weiss, Crit. Rev. Food Sci. Nutr., 2009, 49, 577–606 CrossRef CAS PubMed.
- D. B. Clayton, Stability of W1/O/W2 Double Emulsion Made With Milk Fat and a Simplified Make Procedure and Its Use in Reduced-Fat Cheese, Master's thesis, Utah State University, 2014.
- W.-J. Lin, H.-Z. Liu, A.-M. Shi, L. Liu, B. Adhikari and Q. Wang, Int. J. Food Sci. Technol., 2015, 50, 1538–1544 CrossRef CAS.
- Y. Yang, S. W. Cui, J. Gong, Q. Guo, Q. Wang and Y. Hua, Food Hydrocolloids, 2015, 48, 155–164 CrossRef CAS.
- T. Angkuratipakorn, A. Sriprai, S. Tantrawong, W. Chaiyasit and J. Singkhonrat, Colloids Surf., A, 2017, 522, 310–319 CrossRef CAS.
- M. Yu, H. Liu, Y. Yang, A. Shi, L. Liu, H. Hui and Q. Wang, Int. J. Food Sci. Technol., 2016, 51, 938–945 CrossRef CAS.
- S. Tamnak, H. Mirhosseini, C. P. Tan, B. Tabatabaee Amid, M. Kazemi and S. Hedayatnia, Food Hydrocolloids, 2016, 61, 599–608 CrossRef CAS.
- C. A. Carrillo, T. E. Nypelo and O. J. Rojas, J. Colloid Interface Sci., 2015, 445, 166–173 CrossRef CAS PubMed.
- J. Wang, H. Jing and Y. Wang, Chem. Eng. Sci., 2015, 135, 381–392 CrossRef CAS.
- J. Silvestre de los Reyes and C. Charcosset, Fuel, 2010, 89, 3482–3488 CrossRef CAS.
- T. Ito, Y. Tsuji, K. Aramaki and N. Tonooka, J. Oleo Sci., 2012, 61, 413–420 CrossRef CAS PubMed.
- S. Mun, Y. Choi, S. J. Rho, C. G. Kang, C. H. Park and Y. R. Kim, J. Food Sci., 2010, 75, E116–E125 CrossRef CAS PubMed.
- J. Weiss, I. Scherze and G. Muschiolik, Food Hydrocolloids, 2005, 19(3), 605–615 CrossRef CAS.
- G. T. V. Jeonghee Surh, A. Saehun Mun and D. J. McClements, J. Agric. Food Chem., 2007, 55, 175–184 CrossRef PubMed.
- N. P. Aditya, S. Aditya, H. Yang, H. W. Kim, S. O. Park and S. Ko, Food Chem., 2015, 173, 7–13 CrossRef CAS PubMed.
- B. D. Isailović, I. T. Kostić, A. Zvonar, V. B. Đorđević, M. Gašperlin, V. A. Nedović and B. M. Bugarski, Innovative Food Sci. Emerging Technol., 2013, 19, 181–189 CrossRef.
- S. Reis, A. R. Neves, M. Lúcio, S. Martins and J. L. C. Lima, Int. J. Nanomed., 2013, 8, 117 Search PubMed.
- M. Serdaroğlu, B. Öztürk and M. Urgu, Meat Sci., 2016, 117, 187–195 CrossRef PubMed.
- D. B. Clayton, Dissertations & Theses – Gradworks, 2014.
- J. Li, I.-C. Hwang, X. Chen and H. J. Park, Food Hydrocolloids, 2016, 60, 138–147 CrossRef CAS.
Footnote |
† These authors contributed equally to this work. |
|
This journal is © The Royal Society of Chemistry 2017 |
Click here to see how this site uses Cookies. View our privacy policy here.