DOI:
10.1039/C7RA06592B
(Paper)
RSC Adv., 2017,
7, 43474-43482
Self-assembly of biosurfactant–inorganic hybrid nanoflowers as efficient catalysts for degradation of cationic dyes†
Received
13th June 2017
, Accepted 4th September 2017
First published on 8th September 2017
Abstract
Three-dimensional (3D) hierarchical nanostructures have generated a large amount of interest because of their unique, unusual properties and potential applications. In this article, copper(II) ions as the inorganic component and various biosurfactants as the organic component were used to successfully form 3D nanoflowers via a facile and effective self-assembly template synthesis strategy. It can be confirmed that the biosurfactant molecules can first form complexes with the copper ions, and these complexes then become nucleation sites for primary crystals of copper phosphate, indicating that the interaction between biosurfactant and copper ions leads to the formation of 3D nanoflowers. Several reaction parameters such as aging time and the concentration of the biosurfactant, which play a critical role in the formation process and morphologies of the nanoflowers, were investigated. Under the optimum synthetic conditions, a spherical flowerlike structure with hundreds of nanopetals was obtained. Moreover, the biosurfactant–Cu3(PO4)2·3H2O nanoflowers also showed high stability and catalytic activity for degradation of cationic dyes. Our results demonstrate that the biosurfactant–inorganic 3D nanoflowers, which combined the advantages of the biosurfactant and inorganic material, have potential applications in industrial biocatalysis, biosensors, and environmental chemistry.
Introduction
Nano-structured materials have attracted increasing attention in recent years and great contributions have been made in the morphology-controlled synthesis of micro- and nano-scale structures, such as nanodendrites, core/shell nanoparticles, nanowires, and nanoflowers.1–5 Among them, “nanoflower” is a fantastic name for some of the nanomaterials whose microscopic images resemble flowers. A variety of materials such as carbon,6–8 metals and other elemental materials,9–11 alloys,12,13 and metaloxides/hydroxides14 have been widely reported to form flower-like structure.15,16 Due to the large surface-to-volume ratio compared with that of bulk materials, these nanoflowers have many applications in catalysis, magnetism, nanodevices, sensing and biosensing and medicine.17–24 But nanoflowers are traditionally synthesized under harsh conditions such as high temperature, pressure and the use of toxic organic solvents. Thus, it is still a challenge to explore a low cost and facile synthetic method for the preparation of hierarchical nanoflowers.
Gratifyingly, self-assembly can spontaneously combine different components together to form different kinds of hybrid materials,25 while the template synthesis is an effective approach to synthetize nanomaterials with various morphologies.26 Thus, a self-assembled template synthesis provides a facile and effective low temperature synthetic strategy for the fabrication of well-defined nanoflowers. Especially, the bio-inspired materials with micro- and nano-scale have been proposed as a big breakthrough on the design of functional materials due to the huge advantage of the bio-molecules in directing and assembling the nanoflowers.27–30 Various bio-molecules, such as enzyme,31–36 protein,37 amino acids38 have been applied to synthesis nanoflower. For example, Zare's group reported the novel synthesis of hybrid nanoflowers comprising of Cu3(PO4)2 and proteins for the first time and they also synthetized immobilized enzymes with greatly enhanced activities.39 Subsequently, using the same synthetic route, Wang et al. prepared CaHPO4-α-amylase nanoflowers and they also further elaborated the mechanism for enhanced catalytic activity of immobilized enzyme.40 Although biodegradable amphiphilic molecules have been widely used in the fabrication of nanomaterials41,42 and self-assembly process assisted by amphiphilic molecules can provide a powerful low temperature tool for the synthesis of hierarchical structures,43 the usage of bio-surfactants in the fabrication of nanoflower has not been reported so frequently.
In this paper, a facile and effective self-assembled template synthesis strategy for shape controlled synthesis of three-dimensional (3D) flower-like Cu3(PO4)2·3H2O microstructures were provided using copper(II) ions as the inorganic component and various biosurfactants as the organic component. The hybrid nanoflowers were fully characterized by transmission electron microscopy (TEM), field emission scanning electron microscopy (FE-SEM), Fourier transform infrared (FT-IR) spectroscopy and X-ray diffraction (XRD). Different growth stages and concentration of biosurfactants on the effect of the morphologies of hybrid nanoflowers were intensively identified and studied. The synthesized biosurfactant–Cu3(PO4)2·3H2O hybrid nanoflowers exhibited high stability and catalytic activity for degradation of cationic dyes. Moreover, the catalyst for MB can be recycled and reused at least six cycles with only a slight dropping of catalytic efficiency, suggesting their promising applications in the treatment of waste water.
Experimental
Reagents and materials
Sodium cholate (SC), sodium deoxycholate (SDC), sodium dihydrogen phosphate (NaH2PO4·2H2O) and disodium phosphate dodecahydrate (Na2HPO4·12H2O) were obtained from Sinopharm Chemical Reagent, Co., Ltd (Shanghai, China). Copper sulfate pentahydrate (CuSO4·5H2O) was purchased from Fuchen Chemical Reagent Factory (Tianjin, China). Polypeptide GG-4 were the products of GL Biochem, Co., Ltd (Shanghai, China) and Meilunbio, Co., Ltd (Dalian, China), respectively. Rhodamine B (RhB), rhodamine 6G (R6G) and methylene blue (MB) were purchased from Kermel Chemical Reagent, Co., Ltd (Tianjin, China). The structures of SC, SDC, GG-4 and RhB, R6G, MB were shown in Fig. S1.† All other chemicals were of analytical grade or better. Ultrapure water with a resistivity of 18.25 MΩ cm was obtained using a UPH-IV ultrapure water purifier (China).
Synthesis of biosurfactant–inorganic nanoflowers
Biosurfactant–Cu3(PO4)2 nanoflowers were synthesized similar to the previous literature.25 Briefly, 20 μL of aqueous CuSO4·5H2O solution (120 mM) was added into 3 mL of phosphate buffered saline (PBS) solution (2 mM, pH 7.4) with different concentrations of biosurfactants. The solution was incubation at room temperature for 72 h and the obtained blue products were washed with water for three times, centrifuged at 7000 rpm for 5 min and then dried at room temperature.
Characterization of biosurfactant–inorganic nanoflowers
Transmission electron microscopy (TEM) observations were carried out on a JEM-100 CXII (JEOL) at an accelerating voltage of 80 kV. For sample preparation, the obtained powder was placed on a ultrathin carbon-coated copper grid and the excess powder was wicked away with filter paper, which was then dried by using a near infrared reflection (NIR) lamp before observations. Field-emission scanning electron microscopy (FE-SEM) observations were carried out on SU8010 (HATACHI), a drop of wet powder was placed on a silica wafer to form a thin film then dried by using NIR lamp. Energy-Dispersive X-ray Spectroscopy (EDS) analysis and telemental mapping were also performed on the same FE-SEM microscope. FT-IR spectra were recorded 4000 to 400 cm−1 on a VERTEX-70/70v spectrometer (Bruker Optics, Germany). The samples for FT-IR spectra were prepared by using KBr pellets mixed with the powder we obtained. XRD patterns of the samples were obtained between 10 and 90 in the 2θ scan mode (2.5 min−1) using a Rigaku D/Max 2200 PC diffractometer with Cu Kα radiation (λ = 0.15418 nm) and a graphite monochromator at room temperature. UV-vis measurements were carried out on a computer manipulated spectrometer (UV-vis 4100, Hitachi, Japan).
Degradation of cationic dyes
Three cationic dyes RhB, R6G and MB were chosen as samples to investigate the catalytic activity of our nanoflowers for degradation of cationic dyes by H2O2. The initial concentration of dye solutions were 12.5 μg mL−1, concentration of dye solutions during degradation were detected by UV-vis measurements. The degradation rates were calculated using the following equation:
where C0 and Ct are initial concentration and concentration at time t.
Results and discussion
Microstructures of biosurfactant–inorganic nanoflowers
Xie's group have reported the biomolecule-assisted synthesis of Bi2S3 flowerlike patterns which need a relatively high reaction temperature (150 °C).44 However, in our experiment, the biosurfactant–inorganic nanoflowers were prepared by a facile and efficient low temperature (at room temperature) process by using CuSO4·5H2O as the precursor, and biosurfactants as the assistant of the formation of 3D flower-like microstructures. To validate our design, SC–Cu3(PO4)2·3H2O nanoflowers were firstly prepared. Blue precipitates were formed after 3 days incubation and TEM images shows that the nanoflower morphologies were formed (Fig. 1A–C). SEM images further confirmed the flower-like morphology (Fig. 1D–F) and it can be observed that the samples consist of large quantities of flower-like nanostructures with diameters in the range of 15–30 μm which possess high surface-to-volume ratios and have good monodispersity. Subsequently, the elemental mapping (Fig. 2) and EDS pattern (Fig. S2†) further confirm that C, Na, Cu, P and O elements are homogeneously distributed inside the nanoflowers which gave a proof that the nanoflowers obtained were complex of SC and Cu3(PO4)2·3H2O. When SC was replaced by other two biosurfactants SDC and GG-4, flower-like microstructures were also been found as shown in Fig. S3 (ESI†). We have calculated the surface area and as 3.20 m2 g−1 and an average pore size of 3.23 nm through BET measurement (Fig. S4†).
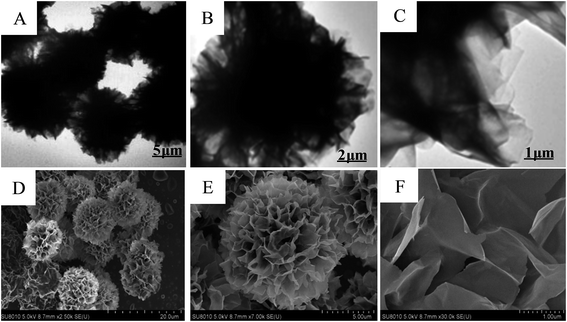 |
| Fig. 1 The detailed characterizations of SC–Cu3(PO4)2·3H2O nanoflowers (0.1 mg mL−1 SC). TEM image of (A) hybrid nanoflowers, (B) a local partial enlarged image of (A), and (C) nanopetals (a local partial enlarged image of (B)). SEM images of (D) hybrid nanoflowers, (E) single nanoflower (a local partial enlarged image of (D)) and (F) nanopetals (a local partial enlarged image of (E)). | |
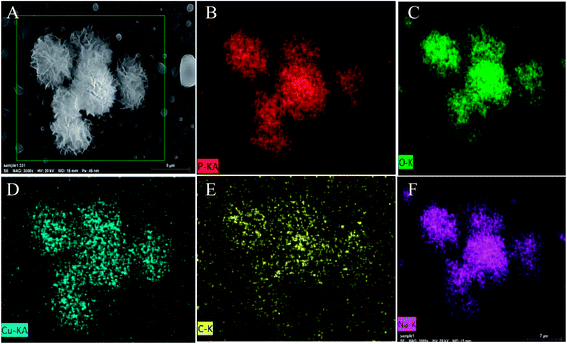 |
| Fig. 2 (A) SEM image of SC–Cu3(PO4)2·3H2O nanoflowers. SEM element mapping analyses of nanoflowers: (B) P, (C) O, (D) Cu, (E) C, (F) Na. | |
FT-IR and XRD analysis
FT-IR spectroscopy directly confirms the formation of 3D nanoflowers. In the spectrum of Cu3(PO4)2·3H2O and SC–Cu3(PO4)2·3H2O nanoflowers (Fig. 3A), the peaks at 1054 and 1150 cm−1 were attributed to P–O and P
O vibrations,36 while the bands at 566 and 603 cm−1 were attributed to the bending vibrations of bridging phosphorous such as O
P–O which indicated the existence of phosphate groups.35 The weak peak at 1620 cm−1 came from the crystal water in Cu3(PO4)2·3H2O. Compared to the spectrum of Cu3(PO4)2·3H2O, the typical bands of SC at 2800–3000 cm−1 for –CH2 and –CH3 were also observed in the spectrum of SC–Cu3(PO4)2·3H2O nanoflowers, indicating the presence of SC component in the hybrid material. Moreover, the spectrum of hybrid nanoflowers didn't show significant shift of the peaks or any new adsorption peaks, indicating the hybrid nanoflowers formed via self-assembly instead of covalent bonding.
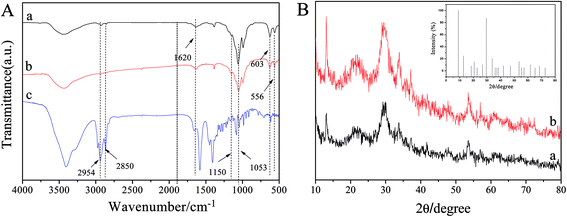 |
| Fig. 3 (A) FT-IR spectra of (a) SC–Cu3(PO4)2·3H2O nanoflowers; (b) Cu3(PO4)2; (c) SC; (B) XRD patterns of (a) Cu3(PO4)2 and (b) SC–Cu3(PO4)2·3H2O nanoflowers, inset is the standard card of Cu3(PO4)2·3H2O (JPSCD00-022-0548). | |
XRD analysis (Fig. 3B and the inset) were performed to further confirm the crystallinity of the nanoflowers. It can be seen that the positions and intensities of the diffraction peaks of Cu3(PO4)2·3H2O are consistent with those obtained from the JCPDS card (00-022-0548). Thus, it can be concluded that even after incorporating SC, the SC–Cu3(PO4)2·3H2O nanoflowers were still well crystallized.33
Effects of the dosage of SC on the morphologies of SC–Cu3(PO4)2·3H2O nanoflowers
In order to explore the effect of the dosage of biosurfactants on the morphologies of SC–Cu3(PO4)2·3H2O nanoflowers, the concentrations of SC which ranged from 0 to 0.5 mg mL−1 were first investigated as a sample. As shown in Fig. 4, in the absence of SC, large amorphous crystals of Cu3(PO4)2·3H2O, but no nanoflowers were formed (Fig. 4A1 and A2). However, when SC was added, the flower-like nanostructures emerged. When the concentration of SC was 0.05 mg mL−1, there were many slices that resembled the petals of flowers with rough fringes (Fig. 4B1). Besides, some nanoflowers with an average size of ∼20 μm were also formed in this concentration (Fig. 4B2). With the increase of SC concentration from 0.05 to 0.1 mg mL−1, the petal-like structure gradually formed flowerlike structure (Fig. 4C1 and C2), while the average size of the nanoflowers decrease from ∼20 μm to ∼15 μm when SC concentration increased to 0.5 mg mL−1 (Fig. 4D1 and D2). The decrease of the average size of the nanoflowers may be attribute to the steric hindrance's enhancement with increase the SC molecules attached to Cu3(PO4)2·3H2O crystal nucleus. The results demonstrated that SC worked as the size- and shape-controlling agent in the synthesis process and the sizes of the nanoflowers were strongly dependent on SC concentration.33,36
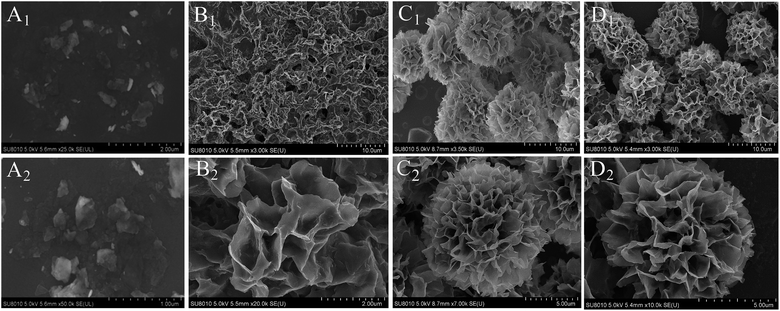 |
| Fig. 4 Effect of different concentrations of SC on the morphologies of SC–Cu3(PO4)2·3H2O nanoflowers hybrid nanoflowers. (A1, A2) 0 mg mL−1; (B1, B2) 0.05 mg mL−1; (C1, C2) 0.1 mg mL−1; (D1, D2) 0.5 mg mL−1. (A2, B2, C2 and D2) are the local partial enlarged images of (A1, B1, C1 and D1), respectively. Other conditions: 120 mmol L−1 Cu2+, 0.1 mol L−1 PBS at pH = 7.4, and reaction for 72 h at 25 °C. | |
Effects of the growth time on the morphologies of SC–Cu3(PO4)2·3H2O nanoflowers
In order to get more information about the growth mechanism of SC–Cu3(PO4)2·3H2O nanoflowers, the morphologies of the hybrid materials were recorded by SEM images as a function of the growth time of the nanoflowers. The experiments were carried out in identical concentrations but for different incubation time (1, 3, 6, 12 and 15 h) and the SEM images shown in Fig. 4 suggest a progressive process of nanoflower assembly. It can be observed that the blue precipitates can be obtained as soon as the addition of CuSO4·5H2O solution to PBS solution which contains SC. At an early growth stage (1 h), both primary crystals of Cu3(PO4)2·3H2O and copper–cholate complexes which formed mainly through coordination between carboxyl groups in the SC backbone and Cu2+ were appeared.41–43 Moreover, these complexes can provide a location for nucleation of the primary crystals of Cu3(PO4)2·3H2O (Fig. 5A1 and A2). When the reaction time reached to 3 h (Fig. 5B1 and B2), the copper–cholate complexes combined into large agglomerates. Subsequently, the kinetically controlled growth of Cu3(PO4)2·3H2O crystals occured on the surface of these agglomerates. As time sequentially increased to 6–12 h, anisotropic growth of these crystals led to the formation of flower-like structure (Fig. 5C1, C2, D1 and D2). When the reaction time reached 15 h, a branched flower-like structure completely formed (Fig. 5E1 and E2). That is to say, in this growth process, SC induced the nucleation of the Cu3(PO4)2·3H2O crystals to form the scaffold for the petals, and also served as a “glue” to bind the petals together.33 Based on the experimental results, the formation mechanism of SC–Cu3(PO4)2·3H2O flowerlike structures as a function of the growth time was shown in Scheme 1.
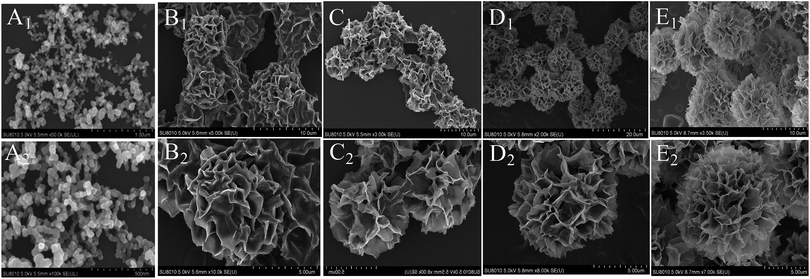 |
| Fig. 5 Effect of different growth time on the morphologies of SC–Cu3(PO4)2·3H2O nanoflowers. (A1, A2) 1 h; (B1, B2) 3 h; (C1, C2) 6 h; (D1, D2) 12 h; (E1, E2) 15 h. (A2, B2, C2, D2 and E2) are the local partial enlarged images of (A1, B1, C1, D1 and E1), respectively. These series of experiments were carried out in identical concentrations: 120 mmol L−1 Cu2+, 0.1 mol L−1 PBS at pH 7.4, 0.1 mg mL−1 SC, and reaction at 25 °C. | |
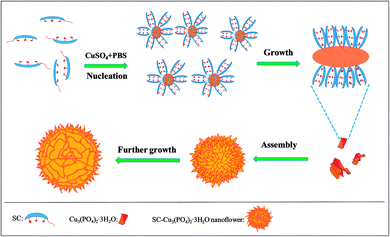 |
| Scheme 1 The formation mechanism of SC–Cu3(PO4)2·3H2O flowerlike structures as a function of the growth time. | |
Catalytic behaviors for the degradation of cationic dyes
It's known that copper compound possess intrinsic peroxidase-like activity in the presence of H2O2.44–46 Therefore, the peroxidase-like activities of the copper–cholate complexes in biosurfactant-incorporated nanoflowers were investigated. Three cationic dyes RhB, R6G and MB (structures of the three dyes were shown in Fig. S1†) were chosen to investigate the peroxidase-like activity of SC–Cu3(PO4)2·3H2O nanoflowers. Firstly, the concentration of each dye detected by UV-vis absorption at different time points during the degradation process were measured and the results were shown in Fig. 5. It can be seen that a visual color change of cationic dyes solution (12.5 μg mL−1) occured only when H2O2 and nanoflowers were co-existence which indicated that the SC–Cu3(PO4)2·3H2O nanoflower had degraded all the three dyes dramatically and exhibited excellent catalytic activities for cationic dyes degradation reaction in the presence of H2O2 (Fig. 6A–C and S5†). The morphology of nanoflower after degradation had also been investigated, as is shown in Fig. S6,† it can be seen that after the degradation of dyes, partial nanoflowers had disaggregated into nanoplates.
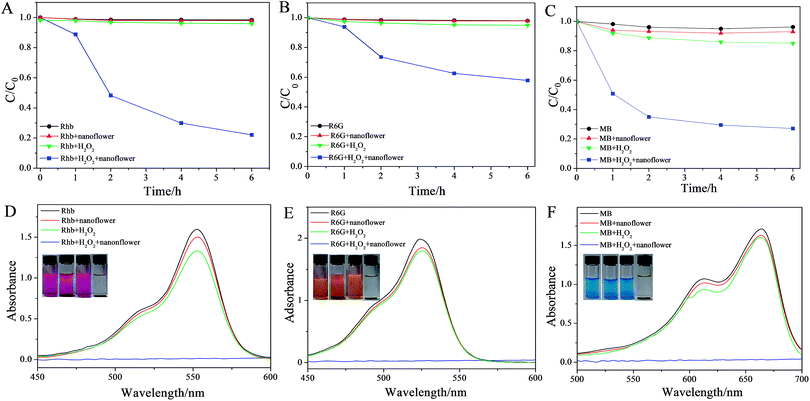 |
| Fig. 6 Catalytic activity of SC–Cu3(PO4)2·3H2O nanoflower for degradation of (A) RhB; (B) R6G; (C) MB, and UV-vis spectrum of (D) RhB; (E) R6G; (F) MB. Inset photos were the color changes of cationic dyes solutions (from left to right): blank control (12.5 μg mL−1 dye solutions), 125 μg mL−1 SC–Cu3(PO4)2·3H2O nanoflower, 125 mmol L−1 H2O2, 125 μg mL−1 SC–Cu3(PO4)2·3H2O nanoflower and 125 mmol L−1 H2O2. The reaction condition is under room temperature, reaction time is 15 h. | |
Meanwhile, in order to trace the degradation process, the changing concentrations of cationic dyes solutions over time were detected by UV-vis (Fig. S5A–C†). The comparison of degradation rates for different dyes shown in Fig. S5D† revealed that at the first stage (1 h), the degradation rates were MB > RhB > R6G. Subsequently the degradation rate of RhB increased, while MB and R6G were both degraded with a lower speed. In general, R6G had the lowest degradation rate, MB performed the highest degradation speed. The linear fit of the ln(Ct/C0) data reveals that the catalytic reaction exhibits pseudo-first-order kinetics for the degradation of the three dyes (Fig. S7†). The rate constant k of the oxidation reaction was determined to be 0.89, 0.25 and 0.22 h−1, corresponding to MB, RhB and R6G respectively, based on eqn (1) and (2). The degradation rate for the three dyes are MB > RhB > R6G.
As we all know, oxidation is the most common method used to degrade dyes and other organic pollutants. In addition to a prompt removal of the colors, OH· radicals was simultaneously able to fully oxidize the dyes, with a complete mineralization of carbon into CO2. Sulfur heteroatoms were converted into innocuous SO42− ions. The mineralization of nitrogen was more complex. Nitrogen atoms in the −3 oxidation state, such as in amino-groups remain at this reduction degree and produced NH4+ cations, subsequently and very slowly converted into NO3− ion. The feasibility of such a degradation could be determined by varying the chemical structures.47 The degradation mechanism of the RhB mainly involved the broken of benzene ring in RhB combined with de-ethylation and hydroxylation process,48 while for MB, it mainly involved the broken of C
N, C
S–C bond the MB central aromatic ring by H+/electron transfer followed with demethylation.49 As we know, it was more effortless to break C
N and C
S–C bond than the benzene ring, leading to a higher degradation rate for MB than RhB. As for RhB and R6G which have similar structures, the difference between degradation rate may due to that RhB had more ethyls than R6G, which was beneficial to accelerate de-ethylation and hydroxylation process.
It is known that copper compound possess intrinsic peroxidase-like activity in the presence of H2O2, which has been reported by many groups.38,45 To verify the peroxidase-like activity is actually occurring in their system, A. K. Dutta et al. tested the as-prepared CuS NPs through the catalytic oxidation of a peroxidase substrate, TMB in the presence of H2O2. Similar to Fe2+or Fe3+, this Cu2+ ion may acts as Fenton-like reagent and interact with the substrate in presence of hydrogen peroxide, resulting in a colored reaction product.45
The reason of the catalytic activity of SC–Cu3(PO4)2·3H2O nanoflowers was associated with Cu3(PO4)2·3H2O framework while the biosurfactants were only induced the nucleation of Cu3(PO4)2·3H2O crystals to form the nanoflowers. The reaction equations are displayed as follows:
Cu2+ + H2O2 → Cu1+ + HOO· + H+ |
Cu1+ + H2O2 → Cu2+ + ·OH + OH− |
In order to further investigate the influence factors of the catalytic activity, MB solution (12.5 μg mL−1) was taken as a sample to investigate the influence of concentrations of nanoflowers and H2O2 on dye degradation. A visible color disparity of MB solution was observed whether change concentration of hybrid nanoflowers (Fig. 7A) or H2O2 (Fig. 7B). The UV/vis absorption spectra also confirm a more thorough degradation of MB solutions with the higher concentration of hybrid nanoflowers or H2O2. What's more, the recycling experiment for the degradation of MB using SC–Cu3(PO4)2·3H2O nanoflower/H2O2 system was also taken, the degradation process was shown in Scheme 2. After 6 cycles, the nanoflowers still performed excellent catalytic activity and the final degradation rate of MB solution remained above 95% in each cycle, which indicated a stable structure and outstanding catalytic activity of our nanoflowers. The degradation rates of MB solution which contains nanoflowers and H2O2 for the six cycles were displayed in Fig. 7C.
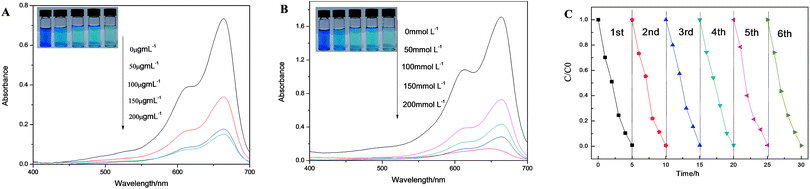 |
| Fig. 7 (A) UV/vis absorption spectra of MB solutions containing H2O2 upon the addition of different concentrations of hybrid nanoflowers (0–200 μg mL−1). (B) UV/vis absorption spectra of MB solutions containing hybrid nanoflowers upon the addition of different concentrations of H2O2 (0–200 mmol L−1). Inset photos were the color change of the solutions. Other conditions: 12.5 μg mL−1 MB solution, 125 μg mL−1 hybrid nanoflowers and reaction 15 h at 25 °C. (C) The first six cycling runs for degradation of 12.5 μg mL−1 MB solution which contains 125 μg mL−1 nanoflowers and 125 mmol L−1 H2O2. | |
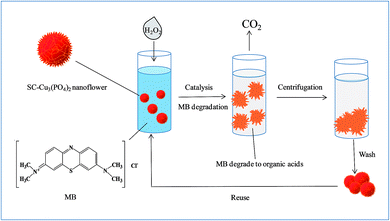 |
| Scheme 2 The scheme of recycling of nanoflowers as an efficient catalyst for degradation of MB. | |
Conclusion
In summary, a facile and effective self-assembled template method for low temperature synthesis of 3D flower-like Cu3(PO4)2·3H2O microstructures through biosurfactants-assisted self-assembly with copper ions were designed. Based on the comprehensive test results and analysis, the following main conclusions are obtained. It can be concluded that the biosurfactants were particularly employed as nucleation and growth sites for deposition of copper phosphate and as structural guiding supporters to form the flower morphology. What's more, the concentration of biosurfactants and the growth time were critical to the sizes and morphologies of the hybrid nanoflowers and our Cu3(PO4)2·3H2O nanoflowers can still performed high catalytic efficiency after reused 6 times, indicating its potential as an efficient and stable catalyst for degradation of organic pollutions in waste water.
Conflicts of interest
There are no conflicts to declare.
Acknowledgements
We gratefully acknowledge the financial support from the Young Scholars Program of Shandong University (2016WLJH20).
References
- A. Mohanty, N. Garg and R. Jin, A Universal Approach to the Synthesis of Noble Metal Nanodendrites and Their Catalytic Properties, Angew. Chem., Int. Ed., 2010, 49, 4962–4966 CrossRef CAS PubMed.
- K. Leung, S. Xuan, X. Zhu, D. Wang, C. Chak, S. Lee, W. Ho and B. Chung, Gold and iron oxide hybrid nanocomposite materials, Chem. Soc. Rev., 2012, 41, 1911–1928 RSC.
- Z. Bai, X. Yan, Y. Li, Z. Kang, S. Cao and Y. Zhang, 3D-Branched ZnO/CdS Nanowire Arrays for Solar Water Splitting and the Service Safety Research, Nature, 2007, 447, 1098–1101 CrossRef PubMed.
- Z. Hu, L. Wang, K. Zhang, J. Wang, F. Cheng, Z. Tao and J. Chen, MoS2 Nanoflowers with Expanded Interlayers as High-Performance Anodes for Sodium-Ion Batteries, Angew. Chem., Int. Ed., 2014, 53, 12794–12798 CrossRef CAS PubMed.
- C. Ma, X. Qi, B. Chen, S. Bao, Z. Yin, X. Wu, Z. Luo, J. Wei and H. Zhang, MoS2 nanoflower-decorated reduced graphene oxide paper for high-performance hydrogen evolution, Nanoscale, 2014, 6, 5624–5629 RSC.
- J. Du, Z. Liu, Z. Li, B. Han, Z. Sun and Y. Huang, Carbon nanoflowers synthesized by a reduction–pyrolysis–catalysisroute, Mater. Lett., 2005, 59, 456–458 CrossRef CAS.
- X. Ma and B. Yuan, Fabrication of carbon nanoflowers by plasma-enhanced chemical vapor deposition, Appl. Surf. Sci., 2009, 255, 7846–7850 CrossRef CAS.
- S. Thongtem, P. Singjai, T. Thongtem and S. Preyachoti, Growth of carbon nanoflowers on glass slides using sparked iron as a catalyst, Mater. Sci. Eng. A, 2006, 423, 209–213 CrossRef.
- J. Bian, S. Shu, J. Li, C. Huang, Y. Li and R. Zhang, Reproducible and recyclable SERS substrates: Flower-like Ag structures with concave surfaces formed by electrodeposition, Appl. Surf. Sci., 2015, 333, 126–133 CrossRef CAS.
- Z. Wang, J. Zhang, J. M. Ekman, J. A. Kenis and Y. Lu, DNA-Mediated Control of Metal Nanoparticle Shape: One-Pot Synthesis and Cellular Uptake of Highly Stable and Functional Gold Nanoflowers, Nano Lett., 2010, 10, 1886–1891 CrossRef CAS PubMed.
- S. Cha, C. Mo, K. Kim and S. Hong, Ferromagnetic cobalt nanodots, nanorices, nanowires and nanoflowers by polyol process, J. Mater. Res., 2005, 20, 2148–2153 CrossRef CAS.
- L. Liu, J. Guan, W. Shi, Z. Sun and J. Zhao, Facile Synthesis and Growth Mechanism of Flower like Ni-Fe Alloy Nanostructures, J. Phys. Chem. C, 2010, 114, 13565–13570 CAS.
- D. Bin, B. Yang, K. Zhang, C. Wang, J. Wang, J. Zhong, Y. Feng, J. Guo and Y. Du, Design of PdAg Hollow Nanoflowers through Galvanic Replacement and Their Application for Ethanol Electrooxidation, Chem. – Eur. J., 2016, 22, 16642–16647 CrossRef CAS PubMed.
- S. Kim, J. Lee, H. Ahn, H. Song and J. Jang, Facile Route to an Efficient NiO Supercapacitor with a Three Dimensional Nanonetwork Morphology, ACS Appl. Mater. Interfaces, 2013, 5, 1596–1603 CAS.
- H. Heli and A. Rahi, Synthesis and Applications of Nanoflowers, Recent Pat. Nanotechnol., 2016, 10, 86–115 CrossRef CAS PubMed.
- B. I. Kharisov, A Review for Synthesis of Nanoflowers, Recent Pat. Nanotechnol., 2008, 2, 190–200 CrossRef CAS PubMed.
- M. Zeng, Y. Li, F. Liu, Y. Yang, M. Mao and X. Zhao, Cu doped OL-1 nanoflower: A UV-vis-infrared light-driven catalyst for gas-phase environmental purification with
very high efficiency, Appl. Catal., B, 2017, 200, 521–529 CrossRef CAS.
- S. K. Arya, S. Saha, J. E. Ramirez-Vick, V. Gupta, S. Bhansali and S. P. Singh, Recent advances in ZnO nanostructures and thin films for biosensor applications: Review, Anal. Chim. Acta, 2012, 737, 1–21 CrossRef CAS PubMed.
- K. Huang, Y. Liu, Y. Liu and L. Wang, Molybdenum disulfide nanoflower-chitosan-Au nanoparticles composites based electrochemical sensing platform for bisphenol A determination, J. Hazard. Mater., 2014, 276, 207–215 CrossRef CAS PubMed.
- S. He, C. Hu, H. Hou and W. Chen, Ultrathin MnO2 nanosheets supported on cellulose based carbon papers for high-power supercapacitors, J. Power Sources, 2014, 246, 754–761 CrossRef CAS.
- D. Wang, Z. Pan, Z. Wu, Z. Wang and Z. Liu, Hydrothermal synthesis of MoS2 nanoflowers as highly efficient hydrogen evolution reaction catalysts, J. Power Sources, 2014, 264, 229–234 CrossRef CAS.
- Y. Liu, Y. Jiao, Z. Zhang, F. Qu, A. Umar and X. Wu, Hierarchical SnO2 Nanostructures Made of Intermingled Ultrathin Nanosheets for Environmental Remediation, Smart Gas Sensor, and Supercapacitor Applications, ACS Appl. Mater. Interfaces, 2014, 6, 2174–2184 CAS.
- L. Hu, Y. Ren, H. Yang and Q. Xu, Fabrication of 3D Hierarchical MoS2/Polyaniline and MoS2/C Architectures for Lithium-Ion Battery Applications, ACS Appl. Mater. Interfaces, 2014, 6, 14644–14652 CAS.
- W. Yang, Z. Gao, J. Wang, J. Ma, M. Zhang and L. Liu, Solvothermal One-Step Synthesis of Ni-Al Layered Double Hydroxide/Carbon Nanotube/Reduced Graphene Oxide Sheet Ternary Nanocomposite with Ultrahigh Capacitance for Supercapacitors, ACS Appl. Mater. Interfaces, 2013, 5, 5443–5454 CAS.
- Y. Huang, X. Ran, Y. Lin, J. Ren and X. Qu, Self-assembly of an organic-inorganic hybrid nanoflower as an efficient biomimetic catalyst for self-activated tandem reactions, Chem. Commun., 2015, 51, 4386–4389 RSC.
- Y. Xu and W. Zhang, Morphology-controlled synthesis of Ag3PO4 microcrystals for high performance photocatalysis, CrystEngComm, 2013, 15, 5407–5411 RSC.
- H. Yao, H. Fang, X. Wang and S. Yu, Hierarchical assembly of micro-/nano-building blocks:bio-inspired rigid structural functional materials, Chem.Soc.Rev., 2011, 40, 3764–3785 RSC.
- N. Xiao and B. J. Venton, Rapid sensitive detection of neurotransmitters at microelectrodes modified with self-assembled SWCNT forests, Anal. Chem., 2012, 84, 7816–7822 CrossRef CAS PubMed.
- G. Zan and Q. Wu, Biomimetic and Bioinspired Synthesis of Nanomaterials/Nanostructures, Adv. Mater., 2016, 28, 2099–2147 CrossRef CAS PubMed.
- Z. Zhang, X. Kong, K. Xiao, G. Xie, Q. Liu, Y. Tian, H. Zhang, J. Ma, L. Wen and L. Jiang, A Bioinspired Multifunctional Heterogeneous Membrane with Ultrahigh Ionic Rectification and Highly Efficient Selective Ionic Gating, Adv. Mater., 2016, 28, 144–150 CrossRef CAS PubMed.
- S. S. Nadar, S. D. Gawas and V. K. Rathod, Self-assembled organic-inorganic hybrid glucoamylase nanoflowers with enhanced activity and stability, Int. J. Biol. Macromol., 2016, 92, 660–669 CrossRef CAS PubMed.
- C. Altinkaynak, S. Tavlasoglu, N. Özdemir and I. Ocsoy, A new generation approach in enzyme immobilization: Organic-inorganic hybrid nanoflowers with enhanced catalytic activity and stability, Enzyme Microb. Technol., 2016, 93, 105–112 CrossRef PubMed.
- Z. Lin, Y. Xiao, Y. Yin, W. Hu, W. Liu and H. Yang, Facile Synthesis of Enzyme-Inorganic Hybrid Nanoflowers and Its Application as a Colorimetric Platform for Visual Detection of Hydrogen Peroxide and Phenol, ACS Appl. Mater. Interfaces, 2014, 6, 10775–10782 CAS.
- J. Sun, J. Ge, W. Liu, M. Lan, H. Zhang, P. Wang, Y. Wang and Z. Niu, Multi-enzyme co-embedded organic-inorganic hybrid nanoflowers: synthesis and application as a colorimetric sensor, Nanoscale, 2014, 6, 255–262 RSC.
- Z. Lin, Y. Xiao, L. Wang, Y. Yin, J. Zheng, H. Yang and G. Chen, Facile synthesis of enzyme-inorganic hybrid nanoflowers and their application as an immobilized trypsin reactor for highly efficient protein digestion, RSC Adv., 2014, 4, 13888–13891 RSC.
- Y. Yin, Y. Xiao, G. Lin, Q. Xiao, Z. Lin and Z. Cai, An enzyme-inorganic hybrid nanoflower based immobilized enzyme reactor with enhanced enzymatic activity, J. Mater. Chem. B, 2015, 3, 2295–2300 RSC.
- K. Kim, J. Jeong, S. Lee, B. Choi and K. Lee, Protein-directed assembly of cobalt phosphate hybrid nanoflowers, J. Colloid Interface Sci., 2016, 484, 44–50 CrossRef CAS PubMed.
- Z. Wu, Z. Wang, Y. Zhang, Y. Ma, C. He, H. Li, L. Chen, Q. Huo, L. Wang and Z. Li, Amino acids-incorporated nanoflowers with an intrinsic peroxidase-like activity, Sci. Rep., 2016, 6, 22412 CrossRef CAS PubMed.
- J. Ge, J. Lei and R. N. Zare, Protein-Inorganic Hybrid Nanoflowers, Nat. Nanotechnol., 2012, 7, 428–432 CrossRef CAS PubMed.
- L. Wang, Y. Wang, R. He, A. Zhuang, X. Wang, J. Zeng and J. Hou, A New Nanobiocatalytic System Based on Allosteric Effect with Dramatically Enhanced Enzymatic Performance, J. Am. Chem. Soc., 2013, 135, 1272–1275 CrossRef CAS PubMed.
- Y. Qiao, Y. Lin, Y. Wang, Z. Yang, J. Liu, J. Zhou, Y. Yan and J. Huang, Hierarchical Self-Assembled One-Dimensional Nanohelices, Nano Lett., 2009, 9, 4500–4504 CrossRef CAS PubMed.
- Y. Qiao, Y. Wang, Z. Yang, Y. Lin and J. Huang, Self-Templating of Metal-Driven Supramolecular Self-Assembly: A General Approach toward 1D Inorganic Nanotubes, Chem. Mater., 2011, 23, 1182–1187 CrossRef CAS.
- Q. Wang, W. Li and J. Shi, Biomolecule-assisted route for shape-controlled synthesis of 3D flower-like CdWO4 microstructures, RSC Adv., 2015, 5, 61330–61336 RSC.
- B. Zhang, X. Ye, W. Hou, Y. Zhao and Y. Xie, Biomolecule-Assisted Synthesis and Electrochemical Hydrogen Storage of Bi2S3 Flowerlike Patterns with Well-Aligned Nanorods, J. Phys. Chem. B, 2006, 110, 8978–8985 CrossRef CAS PubMed.
- A. K. Dutta, S. Das, S. Samanta, P. K. Samanta, B. Adhikary and P. Biswas, Nanoparticles as a mimic peroxidase for colorimetric estimation of human blood glucose level, Talanta, 2013, 107, 361–367 CrossRef CAS PubMed.
- W. P. Kwan and B. M. Volker, Rates of Hydroxyl Radical Generation and Organic Compound Oxidation in Mineral-Catalyzed Fenton-like Systems, Environ. Sci. Technol., 2003, 37, 1150–1158 CrossRef CAS PubMed.
- H. Lachhe, E. Puzenat and A. Houas, Photocatalytic degradation of various types of dyes (Alizarin S, Crocein Orange G, methyl red, congo red, methylene blue) in water by UV-irradiated titania, Appl. Catal., B, 2002, 39, 75–90 CrossRef.
- H. Liu, T. Peng, Z. Peng and K. Dai, Photocatalytic Degradation Mechanism of RhB over Dy-Doped WO3 Photocatalysts, Wuhan Univ. J. Nat. Sci., 2007, 2, 127–132 Search PubMed.
- Z. Yu and S. C. Chuang, Probing Methylene Blue Photocatalytic Degradation by Adsorbed Ethanol with In Situ IR, J. Phys. Chem. C, 2007, 111, 13813–13820 CAS.
Footnote |
† Electronic supplementary information (ESI) available. See DOI: 10.1039/c7ra06592b |
|
This journal is © The Royal Society of Chemistry 2017 |
Click here to see how this site uses Cookies. View our privacy policy here.