DOI:
10.1039/C7RA06688K
(Paper)
RSC Adv., 2017,
7, 38335-38341
Photoresponsive amphiphilic block macrocycles bearing azobenzene side chains†
Received
15th June 2017
, Accepted 19th July 2017
First published on 3rd August 2017
Abstract
Topological molecular architectures play a crucial role in the self-assembly of amphiphilic block copolymers. Many characteristics of macromolecules originate from the special properties of the ends of molecules. Herein, we synthesized well-defined cyclic block copolymers bearing hydrophobic azobenzene and hydrophilic carboxyl moieties in their side-chains, cyclic-PBHMEm-b-PAAn, via sequential atom transfer radical polymerization (ATRP) and intramolecular Cu(I)-catalyzed azide/alkyne cycloaddition (CuAAC) cyclization reaction followed by the selective hydrolysis of tert-butyl ester. The successful synthesis of the amphiphilic block copolymers was fully characterized via conventional gel permeation chromatography, triple detection gel permeation chromatography, nuclear magnetic resonance, Fourier transform infrared spectroscopy, and matrix-assisted laser desorption ionization time-of-flight mass spectrometry. Via investigating the properties of the cyclic polymer, we observed that the cyclic structure displayed faster photoisomerization in solution than its linear precursor. Moreover, the cyclic copolymer exhibits larger changes in the size and morphology of the self-assembled aggregates upon 365 nm light irradiation as compared to its linear counterpart. These interesting findings show that the topological cyclic architecture greatly affects the photoisomerization of azobenzene and copolymeric packing behavior in micellar aggregates.
Introduction
Aggregates with various morphologies can be achieved via the self-assembly of amphiphilic block copolymers in solution.1–3 These functional polymeric nanoparticles have become progressively important for the development of new materials, such as drug delivery vehicles, and as biological cell models.4–7 Over the last few decades, the synthesis, self-assembly, and extensive applications of new polymeric amphiphiles have been receiving significant attention.
Responsive polymers, capable of responding to external stimuli such as pH, redox, and light irradiation, exhibit novel and interesting performances owing to reversible or irreversible changes in their physical properties.8–12 As is known, the reversible transformation between trans- and cis-azobenzene can be induced via irradiation with UV light and reversed upon heating or irradiation with visible light. The photoisomerization of azobenzene chromophores can trigger the change in the properties of azobenzene compounds due to the differences in the shape and dipole moment of trans- and cis-azobenzene.13–17 In addition, azobenzene can undergo cleavage of the azo bond upon treatment with some reductives or azoreductase. Notably, polymeric azobenzene amphiphiles display versatile properties, such as micelle dissociation, colloid deformation, and vesicle bursting and fusion, and promising applications for various nanostructures in the self-assembly systems.18–28 Polymer-based azobenzene, as one of the most attractive responsive materials, has been subjected to extensive investigation.13,29–35
As one of the most important topological polymers, cyclic polymers represent an interesting class of materials since they usually exhibit unique and improved properties as compared to their linear analogues.36–45 Recent advances in macrocycles have demonstrated that the cyclic architecture displays profound effects on the photo-responsive properties, such as liquid crystal formation, birefringence, fluorescence emission, and photoinduced surface relief grating formation, of azobenzene-containing polymers.46–48 Moreover, the self-assembly behavior and properties of amphiphilic copolymers are also found to be sensitive to the endless topology such as morphology, particle dimension, and stability of the self-assembled aggregates.49–56
Rational macromolecular design inspired us to construct cyclic polymeric azobenzene amphiphiles to gain new insights into their structure–property relationship. Herein, we designed and synthesized cyclic amphiphilic block copolymers bearing pendent hydrophobic azobenzene and hydrophilic carboxyl groups, cyclic-PBHMEm-b-PAAn, via successive atom transfer radical copolymerization (ATRP), Cu(I)-catalyzed azide/alkyne cycloaddition (CuAAC) click chemistry,57 and selective hydrolysis of tert-butyl ester. The synthetic routes for cyclic-PBHMEm-b-PAAn are shown in Scheme 1. Furthermore, we mainly investigated the self-assembly behavior and photoresponsive performance of the resultant cyclic amphiphile; it was observed that the cyclic topology endowed unique properties to cyclic-PBHMEm-b-PAAn as compared to that of its linear counterpart.
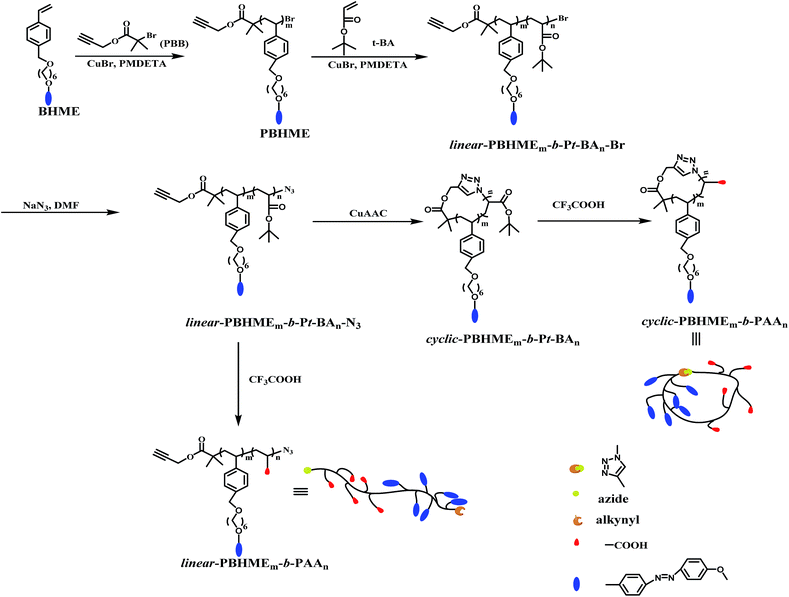 |
| Scheme 1 Synthetic routes for linear-PBHMEm-b-PAAn and cyclic-PBHMEm-b-PAAn. | |
Results and discussion
Synthesis and characterization of linear-PBHMEm-b-Pt-BAn and cyclic-PBHMEm-b-Pt-BAn
The synthetic routes for linear- and cyclic-PBHMEm-b-PAAn are shown in Scheme 1. The ATRP initiator propargyl-2-bromoisobutyrate (PBB) with an terminal alkyne and styrene monomer bearing azobenzene [(6-(4-benzyloxy)hexyloxy)-(4-methoxyphenyl-azo)]ethylene (BHME) were prepared according to previous study.58 First, the homopolymer (PBHME) with α-alkyne-ω-bromine was synthesized via ATRP polymerization of the functionalized monomer BHME in anisole using the PBB initiator. The molecular weights of the PBHME were roughly evaluated from the feeding ratio of the reactants and polymerization conversion. PBHME10 with 10 repeating BHME units (m) was obtained, based on the 1H NMR spectra of PBHME (Fig. S1†) and calculation according to Formula S1.† The block copolymers with α-alkyne-ω-bromine, linear-PBHMEm-b-Pt-BAn-Br, were then prepared via ATRP chain-extension using PBHME10 as the macro-ATRP initiator and t-BA as the second monomer. The number-average molecular weights (Mn) determined by gel permeation chromatography (GPC) increased to 5600 g mol−1 from the original value of 5100 g mol−1, and narrow molecular weight distributions (Mw/Mn) were exhibited after chain-extension (Fig. S2†). The repeating t-BA units (n) of the block copolymer were calculated based on the 1H NMR spectra of the copolymer (Fig. S1†) and Formula S2,† which indicated the synthesis of the copolymer linear-PBHME10-b-Pt-BA3-Br. The linear precursor linear-PBHME10-b-t-BA3-N3 was prepared from linear-PBHME10-b-t-BA3-Br via nucleophilic substitution reaction with NaN3. Cyclic-PBHME10-b-t-BA3 was prepared via the copper(I)-catalyzed azide–alkyne cycloaddition (CuAAC) of linear-PBHME10-b-Pt-BA3-N3 in highly diluted DMF solutions (the detailed process is provided in the ESI†). The successful preparation of the cyclic polymer was verified by gel permeation chromatography (GPC), triple-detection GPC (TD-GPC), Fourier transform infrared (FTIR) and proton nuclear magnetic resonance (1H NMR) spectroscopy, and matrix assisted laser desorption/ionization time of flight (MALDI-TOF) mass spectrometry. The GPC curves of cyclic-PBHME10-b-Pt-BA3 and its linear-precursor present a symmetric peak with a narrow molecular weight distribution (Fig. 1). The cyclic-sample shifted to a longer elution time as compared to the linear precursor; this can be attributed to the fact that the more compact cyclic architecture reduces the hydrodynamic volume. The number-average molecular weight (Mn,GPC and Mn,NMR) of the linear- and cyclic-PBHME10-b-Pt-BA3 obtained by GPC measurement and calculated based on the 1H NMR spectra are shown in Table 1. By monitoring the FTIR spectra, it was observed that the characteristic signals of azide at 2100 cm−1 and alkyne at 3300 cm−1 for linear-PBHME10-b-Pt-BA3 disappeared after CuAAC cyclization (Fig. S3(A)†). The comparison of the 1H NMR spectra of the linear- and cyclic-copolymers (Fig. 1(B)) shows that the signal at around 2.3–2.4 ppm (a), which corresponds to the terminal alkynyl proton of the linear-sample, disappears in the cyclic-sample, and the characteristic signal at around 3.7 ppm (s) adjacent to the azide group in the linear-sample shifts to 5.0–5.5 ppm after ring closure due to the formation of triazole.59 Moreover, cyclic-PBHME10-b-Pt-BA3 was further confirmed by TD-GPC and MALDI-TOF mass spectrometry measurements. The number-average molecular weights (Mn,TD-GPC), absolute molecular weights (Mw,TD-GPC), intrinsic viscosity values ([η]), and hydrodynamic radius (Rh) of the linear- and cyclic-copolymers analyzed by TD-GPC are shown in Table 1 (the TD-GPC traces are displayed in Fig. S4†).60,61 It is observed that the Mw,TD-GPC of cyclic-PBHME10-b-Pt-BA3 is consistent with that of its linear precursor. Moreover, the cyclic polymer exhibits a smaller [η] value as compared to its linear counterpart; this can be ascribed to the cyclic topology. The MALDI-TOF mass spectra of cyclic- and linear-PBHME10-b-Pt-BA3 are shown in Fig. S5,† and the corresponding mass data are listed in Table S1.† All series of mass peaks of the linear- and cyclic-copolymers can be well assigned and are in good agreement with the theoretical peaks. These abovementioned results strongly support the successful preparation of well-defined cyclic-PBHME10-b-Pt-BA3.
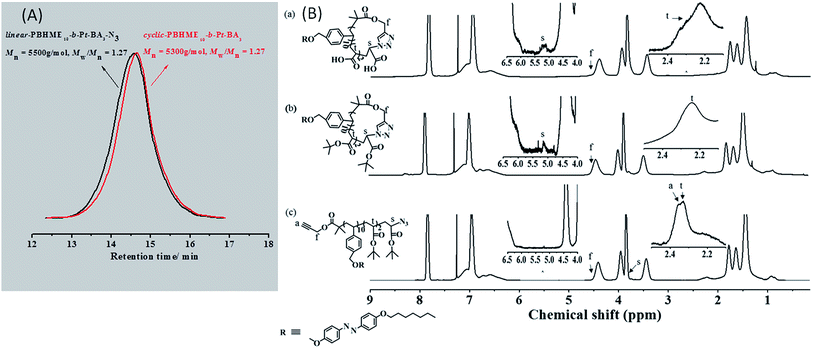 |
| Fig. 1 (A) GPC traces of cyclic-PBHME10-b-Pt-BA3 and linear-PBHME10-b-t-BA3-N3 using THF as the eluent. (B) 1H NMR spectra of cyclic-PBHME10-b-t-AA3 (a), cyclic-PBHME10-b-t-BA3 (b), and linear-PBHME10-b-t-BA3-N3 (c). | |
Table 1 The number-average molecular weights (Mn), molecular weight distribution (Mw/Mn), absolute molecular weights (Mw,TD-GPC), intrinsic viscosity ([η]), and hydrodynamic radius (Rh,TD-GPC) of cyclic-PBHME10-b-t-BA3 and linear-PBHME10-b-t-BA3-N3 determined by conventional GPC and three detection GPC (TD-GPC) as well as 1H NMR spectraa
|
GPC |
TD-GPC |
Mn,GPC (g mol−1) |
Mw/Mn |
Mn,NMR (g mol−1) |
Mn,TD-GPC (g mol−1) |
Mw,TD-GPC (g mol−1) |
Mw/Mn |
[η] (mL g−1) |
Rh,TD-GPC (nm) |
dn/dcb (mL g−1) |
L: linear-PBHME10-b-t-BA3-N3, C: cyclic-PBHME10-b-t-BA3. The dn/dc values for the linear polymer were determined by Wyatt Optilab rEX using at least three different concentrations. The dn/dc value of the cyclic polymer was assumed to be equal to that of the linear precursor.60,61 |
L |
5500 |
1.27 |
5000 |
9700 |
11 900 |
1.23 |
5.9 |
2.1 |
0.1977 |
C |
5300 |
1.27 |
5000 |
9500 |
11 100 |
1.18 |
5.5 |
2.0 |
0.1977 |
Selective hydrolysis of linear- and cyclic-PBHMEm-b-Pt-BAn
The linear- and cyclic-P(BHME10-b-t-BA3) were then reacted with trifluoroacetic acid at room temperature to convert t-BA into acrylic acid (AA) via selective hydrolysis.59 The successful selective hydrolysis was confirmed by FT-IR, 1H NMR, and 13C NMR (Fig. 1(B) and S3†). The 13C NMR and FT-IR spectra in Fig. S3† showed that the tert-butyl ester groups entirely disappeared and transformed into carboxyl groups after the hydrolysis. Moreover, as compared to those of the 1H NMR spectra of the cyclic-copolymers obtained before and after hydrolysis, the two characteristic peaks at around 4.4 ppm (f) of the methylene protons adjacent to the ester groups were exactly the same, indicating that the ester groups in the cyclic backbone were not damaged during the selective hydrolysis.
Glass transition temperature (Tg) and photoisomerization of the cyclic biblock amphiphilic copolymer in a THF solution
The glass transition temperature Tg of the linear- and cyclic-PBHME10-b-PAA3 was evaluated via differential scanning calorimetry (DSC). As shown in Table 2, the Tg of the cyclic sample was higher than that of its linear counterpart; this can be attributed to the absence of main-chain ends in the macrocycle.42,62 Both the linear and cyclic copolymers showed only one Tg during the DSC measurement perhaps because the AA units in the block copolymers have a smaller ratio.
Table 2 Glass transition temperatures (Tg) of linear- and cyclic-PBHME10-b-PAA3 determined by differential scanning calorimetry (DSC) and kinetic parameters for the trans-to-cis and cis-to-trans isomerization of linear- and cyclic-PBHME10-b-PAA3 in THF solutione
Sample |
Tg, °C |
ΔTg, °C |
kea × 10−3 s−1 |
ke/k′eb |
kHc × 10−4 s−1 |
kH/k′Hd |
Rate constant of trans-to-cis photoisomerization. Rate constant ratio of trans-to-cis isomerization of the cyclic-polymer relative to the linear-polymer. Rate constant of trans-to-cis recovery. Rate constant ratio of cis-to-trans recovery of the cyclic-polymer relative to the linear-polymer. L: linear-PBHME10-b-PAA3-N3 and C: cyclic-PBHME10-b-PAA3. |
L |
44 |
11 |
2.25 |
2.43 |
0.62 |
1.98 |
C |
55 |
5.46 |
1.23 |
The photoisomerization of trans-to-cis and cis-to-trans of the linear- and cyclic-PBHME10-b-PAA3 in THF was investigated and compared under 365 nm and 435 nm light irradiation at different time intervals until the photo-stationary state was achieved (Fig. 2). The first-order kinetics curves of the photoisomerization are plotted in Fig. 3 on the basis of the Formulas S3 and S4,† and then, the rate constants (ke and kH) of the cyclic- and linear-PBHME10-b-PAA3 and the cyclic/linear ratio were calculated and are summarized in Table 2. The cyclic product displays larger ke and kH values, indicating that the cyclic sample has faster photoisomerization than its linear precursor. We speculate that the more flexible linear polymer may have a higher degree of random coil conformations as compared to the cyclic polymer without main-chain ends, which probably leads a more transition resistance for its trans-to-cis isomerization.14,48
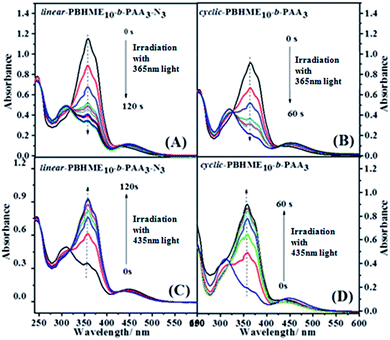 |
| Fig. 2 UV-vis absorption changes of linear- and cyclic-PBHME10-b-PAA3 in THF at different time intervals as a function of 365 nm (1 mW cm−2) (A and B) and 435 nm (60 mW cm−2) (C and D) light irradiation at room temperature, respectively. The concentration of the solution is 2.0 × 10−5 g mL−1. | |
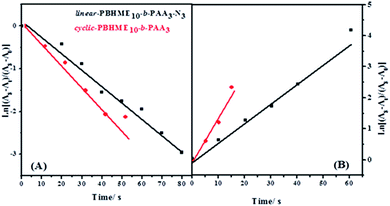 |
| Fig. 3 First-order kinetic curves for the trans-to-cis photoisomerization of linear- and cyclic-PBHME10-b-PAA3 during irradiation with 365 nm UV light (1 mW cm−2) (A) and first-order kinetics curves for cis-to-trans recovery during irradiation with 435 nm light (60 mW cm−2) (B). The concentration of the polymers in THF is 2.0 × 10−5 g mL−1. | |
Self-assembly behaviors and photo-responsive performance of the aggregates from linear- and cyclic-PBHMEm-b-PAAn
The self-assembly behavior of cyclic-PBHME10-b-PAA3 was investigated using a mixture solution of water/THF. The self-assembly of the linear precursor was conducted under the same conditions as its cyclic counterpart to identify the influence of the cyclic structure on its properties. A representative self-assembly solution was directly prepared by slowly adding water to a solution of the polymer in THF with an initial concentration of 0.20 mg mL−1 until the mixture solution reached the ratio of 3/10 (v/v) of water/THF. The morphology and sizes of the self-assembled micellar aggregates were verified via transmission electron microscopy (TEM) and dynamic light scattering (DLS). Both the cyclic and linear amphiphilic polymers formed spherical aggregates via self-assembly in the water/THF mixture solution (Fig. 4 and 5). The aggregate sizes of the cyclic and linear copolymers were both around 150 ± 30 nm, as observed from the TEM image, and about 550 nm, as observed from the hydrodynamic diameters measured by DLS. The sizes obtained via TEM are smaller than those obtained from DLS owing to sample shrinkage during the sample preparation process for TEM measurements. Subsequently, the photo-responsive behaviors of the self-assembled aggregates were investigated by the trans-to-cis photoisomerization of azobenzene under UV light irradiation (365 nm, 1 mW cm−2) and then thermally-driven cis-to-trans return by placing the irradiated sample in the dark at room temperature until the photo-stationary states were reached. Moreover, the UV-vis absorption spectra of the aggregate solutions under the abovementioned condition of photoisomerization were obtained. As shown in Fig. 6, the absorbance at around 450 nm corresponding to the cis isomers increased gradually together with a decrease in the absorbance at around 358 nm, which corresponded to the trans isomers upon UV light irradiation. Then, cis-to-trans isomerization reversal occurred when the irradiated sample was placed in the dark. It was noted that a portion of the spherical aggregates of both the linear- and cyclic-polymers became smaller after exposing the aggregate solutions to 365 nm light, and then, most of the aggregates re-formed after thermally-driven back-isomerization in the dark for a period of time (Fig. 5). The photo-responsive behavior of the aggregates was further confirmed by DLS measurement, which showed that the aggregate sizes reduced to around 450 nm for the linear structure and 220 nm for the cyclic structure from the initial size of 550 nm upon UV light irradiation, and then, both sizes recovered and became slightly larger (about 660 nm) after thermal cis-to-trans relaxation. In addition, gaps appeared outside of the spherical aggregates after UV light irradiation, and especially distinct larger gaps similar to holes were observed for the cyclic-sample as compared to those for the linear sample. The reversible changing of disruption and re-formation of micellar aggregates can be attributed to the unique trans-to-cis photoisomerization of azobenzene. The curved cis-configuration of azobenzene has a larger dipole moment and a shorter length between the 4 and 4′ positions of two benzene rings as compared to the clavated trans-configuration. The variation in the configuration and polarity between the trans and cis isomers of azobenzene mesogens can transform the hydrophilic/hydrophobic balance of amphiphilic copolymers in selective solvents during the trans-to-cis isomerization process. Upon UV light irradiation, the hydrophobic PBHME block in the micellar aggregates formed a more compact region because of its configuration change via trans-to-cis photoisomerization.26 As a result, the THF solvent, which was wrapped in the aggregates, diffused into the solution, resulting in gaps. The cyclic structure exhibits more sensitive photo-responsive properties such as larger changes in the size and morphology of the aggregate micelles as compared to the linear structure upon 365 nm light irradiation. We speculate that the cyclic amphiphile may self-assemble into relatively loose aggregates due to the rigidity of its main chain, which is imposed by the cyclic conformation, as compared to the linear amphiphile.51,53
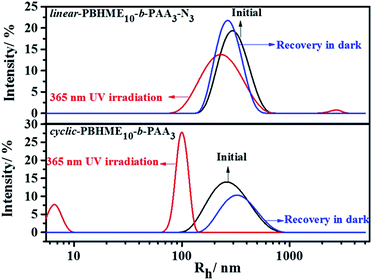 |
| Fig. 4 Hydrodynamic radius (Rh) distribution of the aggregates formed by linear-PBHME10-b-PAA3-N3 and cyclic-PBHME10-b-PAA3 upon 365 nm light irradiation (50 μW cm−2) and stored in the dark for an appropriate period of time. | |
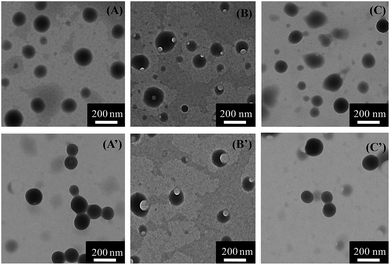 |
| Fig. 5 TEM images of the spherical aggregates of linear and cyclic-PBHME10-b-PAA3 formed in H2O/THF solvent (initial concentration: 0.20 mg mL−1 in THF solvent; H2O/THF (v/v) = 3/10). (A) Initial aggregates formed by linear-PBHME10-b-PAA3; (A′) initial aggregates formed by cyclic-PBHME10-b-PAA3; (B) aggregates formed by linear-PBHME10-b-PAA3 after UV irradiation for 4.2 min; (B′) aggregates formed by cyclic-PBHME10-b-PAA3 after UV irradiation for 5.5 min; (C) aggregates of the irradiated linear-PBHME10-b-PAA3 after storage in the dark for 6 h and (C′) aggregates of the irradiated cyclic-PBHME10-b-PAA3 after storage in the dark for 10 h. | |
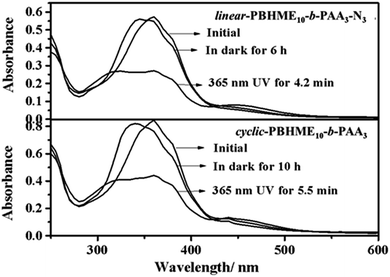 |
| Fig. 6 UV-vis absorption changes for linear-PBHME10-b-PAA3-N3 and cyclic-PBHME10-b-PAA3 in THF/water solution under 365 nm UV light irradiation (50 μW cm−2) and after the irradiated sample was placed in the dark at room temperature. The concentration of the solutions was 2.0 × 10−5 g mL−1. | |
Conclusions
In summary, well-defined cyclic block copolymers bearing hydrophobic azobenzene and hydrophilic carboxyl moieties in their side-chains, cyclic-PBHMEm-b-PAAn, were successfully prepared via sequential ATRP and intramolecular CuAAC cyclization reaction followed by the selective hydrolysis of tert-butyl ester. The investigations on the amphiphilic block macrocycles indicate that the cyclic amphiphilic biblock copolymers display faster photoisomerization of trans-to-cis and cis-to-trans in THF than their linear precursors. In addition, the self-assembled micellar aggregates of the cyclic architecture in the mixture solution of water/THF exhibit larger changes in size and morphology upon 365 nm light irradiation as compared to those of their linear counterparts. These interesting differences between the cyclic- and linear copolymers could be attributed to the topological cyclic architecture, which indicated that the cyclic architecture greatly affected the photoisomerization of azobenzene and polymeric packing behaviors in micellar aggregates. This study provides new insight into the structure–property relationship and gives guidance to future designs for the development of responsive-related functional materials. Further studies aimed at functional polymers based on azobenzene are being conducted by our research group.
Acknowledgements
This work was supported by the National Science Foundation of China (No. 21234005), the State and Local Joint Engineering Laboratory for Novel Functional Polymeric Materials, the Priority Academic Program Development of Jiangsu Higher Education Institutions (PAPD), and the Program of Innovative Research Team of Soochow University.
Notes and references
- Y. Mai and A. Eisenberg, Chem. Soc. Rev., 2012, 41, 5969–5985 RSC.
- K. Yu and A. Eisenberg, Macromolecules, 1998, 31, 3509–3518 CrossRef CAS.
- D. Chen and M. Jiang, Acc. Chem. Res., 2005, 38, 494–502 CrossRef CAS PubMed.
- Z. Ge and S. Liu, Chem. Soc. Rev., 2013, 42, 7289–7325 RSC.
- C. Allen, Y. Yu, D. Maysinger and A. Eisenberg, Bioconjugate Chem., 1998, 9, 564–572 CrossRef CAS PubMed.
- Z. Zhang, R. Ma and L. Shi, Acc. Chem. Res., 2014, 47, 1426–1437 CrossRef CAS PubMed.
- C. Allen, D. Maysinger and A. Eisenberg, Colloids Surf., B, 1999, 16, 3–27 CrossRef CAS.
- D. Han, X. Tong and Y. Zhao, Macromolecules, 2011, 44, 437–439 CrossRef CAS.
- E. Fleige, M. Quadir and R. Haag, Adv. Drug Delivery Rev., 2012, 64, 866–884 CrossRef CAS PubMed.
- J. Hu and S. Liu, Macromolecules, 2010, 43, 8315–8330 CrossRef CAS.
- J. Jiang, X. Tong and Y. Zhao, J. Am. Chem. Soc., 2005, 127, 8290–8291 CrossRef CAS PubMed.
- S. Uchiyama and Y. Makino, Chem. Commun., 2009, 19, 2646–2648 RSC.
- Y. Zhao and T. Ikeda, Smart Light responsive Materials Azobenzene-Containing Polymers and Liquid Crystals, John Wiley & Sons, Inc., Hoboken, New Jersey, 2009 Search PubMed.
- A. Beharry and G. Woolley, Chem. Rev., 1989, 89, 1915–1925 CrossRef.
- O. Sadovski, A. Beharry, F. Zhang and G. A. Woolley, Angew. Chem., Int. Ed., 2009, 48, 1484–1486 CrossRef CAS PubMed.
- A. Beharry and G. Woolley, Chem. Soc. Rev., 2011, 40, 4422–4437 RSC.
- H. Rau, Angew. Chem., Int. Ed., 1973, 12, 224–235 CrossRef.
- J. Lu, F. Zhou, L. Li, Z. Zhang, F. Meng, N. Zhou and X. Zhu, RSC Adv., 2016, 6, 58755–58763 RSC.
- S. Luo, Y. Liu, F. Wang, Q. Fei, B. Shi, J. An, C. Zhao and C. H. Tung, Analyst, 2016, 141, 2879–2882 RSC.
- J. Rao, C. Hottinger and A. Khan, J. Am. Chem. Soc., 2014, 136, 5872–5875 CrossRef CAS PubMed.
- A. Wong, A. Prinzen and E. Gillies, Polym. Chem., 2016, 7, 1871–1881 RSC.
- Y. Zhao, Macromolecules, 2012, 45, 3647–3657 CrossRef CAS.
- R. Dong, B. Zhu, Y. Zhou, D. Yan and X. Zhu, Polym. Chem., 2013, 4, 912–915 RSC.
- K. Han, W. Su, M. Zhong, Q. Yan, Y. Luo, Q. Zhang and Y. Li, Macromol. Rapid Commun., 2008, 29, 1866–1870 CrossRef CAS.
- X. Tong, G. Wang, A. Soldera and Y. Zhao, J. Phys. Chem. B, 2005, 109, 20281–20287 CrossRef CAS PubMed.
- D. Wang and X. Wang, Prog. Polym. Sci., 2013, 38, 271–301 CrossRef CAS.
- E. Blasco, B. V. K. J. Schmidt, C. Barner-Kowollik, M. Pinol and L. Oriol, Macromolecules, 2014, 47, 3693–3700 CrossRef CAS.
- W. Sun, X. He, C. Gao, X. Liao, M. Xie, S. Lin and D. Yan, Polym. Chem., 2013, 4, 1939–1949 RSC.
- J. A. Lv, Y. Liu, J. Wei, E. Chen, L. Qin and Y. Yu, Nature, 2016, 537, 179–184 CrossRef CAS PubMed.
- R. Walker, H. Audorff, L. Kador and H. Schmidt, Adv. Funct. Mater., 2009, 19, 2630–2638 CrossRef CAS.
- J. Lu, A. Xia, N. Zhou, W. Zhang, Z. Zhang, X. Pan, Y. Yang, Y. Wang and X. Zhu, Chem.–Eur. J., 2015, 21, 2324–2329 CrossRef CAS PubMed.
- S. Iamsaard, S. J. Asshoff, B. Matt, T. Kudernac, J. Cornelissen, S. P. Fletcher and N. Katsonis, Nat. Chem., 2014, 6, 229–235 CrossRef CAS PubMed.
- J. Lu, G. Jiang, Z. Zhang, W. Zhang, Y. Yang, Y. Wang, N. Zhou and X. Zhu, Polym. Chem., 2015, 6, 8144–8149 RSC.
- P. Weis, D. Wang and S. Wu, Macromolecules, 2016, 49, 6368–6373 CrossRef CAS.
- Y. Zhao and J. He, Soft Matter, 2009, 5, 2686–2693 RSC.
- K. Zhang, M. A. Lackey, J. Cui and G. N. Tew, J. Am. Chem. Soc., 2011, 133, 4140–4148 CrossRef CAS PubMed.
- S. Honda, T. Yamamoto and Y. Tezuka, Nat. Commun., 2013, 4, 1–9 Search PubMed.
- S. H. Lahasky, X. Hu and D. Zhang, ACS Macro Lett., 2012, 1, 580–584 CrossRef CAS.
- J. Poelma, K. Ono, D. Miyajima, T. Aida, K. Satoh and C. J. Hawker, ACS Nano, 2012, 6, 10845–10854 CrossRef CAS PubMed.
- X. Zhu, N. Zhou, Z. Zhang, B. Sun, Y. Yang, J. Zhu and X. Zhu, Angew. Chem., Int. Ed., 2011, 50, 6615–6618 CrossRef CAS PubMed.
- X. Wan, T. Liu and S. Liu, Biomacromolecules, 2011, 12, 1146–1154 CrossRef CAS PubMed.
- M. D. Hossain, D. Lu, Z. Jia and M. J. Monteiro, ACS Macro Lett., 2014, 3, 1254–1257 CrossRef CAS.
- M. A. Cortez, W. T. Godbey, Y. Fang, M. E. Payne, B. J. Cafferty, K. A. Kosakowska and S. M. Grayson, J. Am. Chem. Soc., 2015, 137, 6541–6549 CrossRef CAS PubMed.
- C. W. Bielawski, D. Benitez and R. H. Grubbs, Science, 2002, 297, 2041–2044 CrossRef CAS PubMed.
- H. Zhang, W. Wu, X. Zhao and Y. Zhao, Macromolecules, 2017, 50, 3411–3423 CrossRef CAS.
- D. Han, X. Tong, Y. Zhao, T. Galstian and Y. Zhao, Macromolecules, 2010, 43, 3664–3671 CrossRef CAS.
- H. Zhang, N. Zhou, X. Zhu, X. Chen, Z. Zhang, W. Zhang, J. Zhu, Z. Hu and X. Zhu, Macromol. Rapid Commun., 2012, 33, 1845–1851 CrossRef CAS PubMed.
- Y. Sun, Z. Wang, Y. Li, Z. Zhang, W. Zhang, X. Pan, N. Zhou and X. Zhu, Macromol. Rapid Commun., 2015, 36, 1341–1347 CrossRef CAS PubMed.
- K. Heo, Y. Y. Kim, Y. Kitazawa, M. Kim, K. S. Jin, T. Yamamoto and M. Ree, ACS Macro Lett., 2014, 3, 233–239 CrossRef CAS.
- R. J. Williams, A. Pitto-Barry, N. Kirby, A. P. Dove and R. K. O'Reilly, Macromolecules, 2016, 49, 2802–2813 CrossRef CAS PubMed.
- X. Fan, B. Huang, G. Wang and J. Huang, Macromolecules, 2012, 45, 3779–3786 CrossRef CAS.
- S. Honda, T. Yamamoto and Y. Tezuka, J. Am. Chem. Soc., 2010, 132, 10251–10253 CrossRef CAS PubMed.
- D. E. Lonsdale and M. J. Monteiro, J. Polym. Sci., Part A: Polym. Chem., 2011, 49, 4603–4612 CrossRef CAS.
- Y. Dong, Y. Tong, B. Dong, F. Du and Z. Li, Macromolecules, 2009, 42, 2940–2948 CrossRef CAS.
- M. Schappacher and A. Deffieux, Science, 2008, 319, 1512–1515 CrossRef CAS PubMed.
- Y. Li, H. Su, X. Feng, K. Yue, Z. Wang, Z. Lin, X. Zhu, Q. Fu, Z. Zhang, S. Z. D. Cheng and W. Zhang, Polym. Chem., 2015, 6, 827–837 RSC.
- B. Laurent and S. Grayson, J. Am. Chem. Soc., 2006, 128, 4238–4239 CrossRef CAS PubMed.
- G. Huang, J. Zhu, Z. Zhang, W. Zhang, N. Zhou and X. Zhu, J. Macromol. Sci., Part A: Pure Appl. Chem., 2013, 50, 193–199 CrossRef CAS.
- Y. Cai, J. Lu, F. Zhou, X. Zhou, N. Zhou, Z. Zhang and X. Zhu, Macromol. Rapid Commun., 2014, 35, 901–907 CrossRef CAS PubMed.
- H. Durmaz, A. Dag, G. Hizal and U. Tunca, J. Polym. Sci., Part A: Polym. Chem., 2011, 49, 1195–1200 CrossRef CAS.
- H. Durmaz, A. Dag, G. Hizal and U. Tunca, J. Polym. Sci., Part A: Polym. Chem., 2010, 48, 5083–5091 CrossRef CAS.
- G. Nossarev and T. Hogen-Esch, Macromolecules, 2002, 35, 1604–1610 CrossRef CAS.
Footnote |
† Electronic supplementary information (ESI) available: Materials, polymer characterization data, DLS and UV-vis data. See DOI: 10.1039/c7ra06688k |
|
This journal is © The Royal Society of Chemistry 2017 |
Click here to see how this site uses Cookies. View our privacy policy here.