DOI:
10.1039/C7RA06817D
(Paper)
RSC Adv., 2017,
7, 41282-41288
Structure and photoluminescence properties of novel Sr6Ca4(PO4)6F2:Re (Re = Eu2+, Mn2+) phosphors with energy transfer for white-emitting LEDs
Received
19th June 2017
, Accepted 16th August 2017
First published on 23rd August 2017
Abstract
A series of Sr6Ca4(PO4)6F2:Eu2+,Mn2+ phosphors were synthesized through traditional solid state reaction. The structural and spectroscopic properties of the series samples along with the energy transfer from Eu2+ to Mn2+ ions have been investigated in detail. Under excitation at 295 or 365 nm, Eu2+ singly doped phosphors exhibit a broad emission band centered at 450 nm. When Eu2+ and Mn2+ were co-doped in Sr6Ca4(PO4)6F2, a broad blue emission band peaking at 450 nm and an orange emission band at 570 nm appeared simultaneously upon ultraviolet (365 nm) excitation, which result from the 4f–5d transition of Eu2+ ions and the 4T1–6A1 transition of Mn2+ ions, respectively. By controlling the doping concentration of Mn2+ ions, a series of tunable luminescent colors including white light are obtained with a wide band emission and a correlated color temperature of 6662 K. The possible energy transfer mechanism was proposed to be dipole–quadrupole, with regard to the analysis of photoluminescence spectra and decay curves experimental results of the series samples. The critical distance between the Eu2+ and Mn2+ ions has been calculated by both the concentration quenching method (11.53 Å) and the spectral overlap method (9.43 Å). Overall, this research indicated that the prepared samples may be potentially applied in white-light-emitting diodes under UV-pumping.
1 Introduction
As a next-generation light source, white-light-emitting diodes (w-LEDs) have gained tremendous attention because of their high color rendering, eco-friendliness, and high brightness characteristics compared to traditional fluorescent light sources.1–6 At present, most of the commercially phosphor-converted LEDs involve a blue emitting (In,Ga)N chip with a (Y,Gd)3Al5O12:Ce3+ phosphor. Meanwhile due to the lack of the red emission spectra, these phosphors suffer from low color rendering index and high correlated color temperature. In order to overcome these major drawbacks, an effective method is to obtain a phosphor which is a blend of blue, green, and red tricolor phosphors (RGB phosphors), which is radiated by ultraviolet (300–400 nm) light.7–10 Using this method, a kind of w-LEDs with excellent color points can be realized. However, these phosphors exhibit low efficiencies in blue emission due to re-absorption by the other phosphors. Therefore, developing a kind of single-phase white-emitting phosphor consisting of an activator and a sensitizer with the same host is indispensable.11–15
One approach to realize a single phase white emitting phosphor is to co-doped Eu2+ and Mn2+ ions.16–18 The Mn2+ ions doped into a sample show a broad band emission, which was assigned from the 4T1 → 6A1 transition. However, electrons of the 3d shell are strongly influenced by the effect of site symmetry and crystal field strength, and Mn2+ emission band changes from green (week crystal field of the tetrahedrally coordinated Mn2+) to red (strong crystal field of the octahedrally coordinated Mn2+) region.19–21 Additionally, owing to the forbidden d–d transitions of Mn2+, the absorption and emission energy of Mn2+ are relatively weak. As a kind of an efficient sensitizer, Eu2+ can transfer energy to Mn2+ in many hosts. The broad emission bands covering from blue to red spectral range in Eu2+/Mn2+ co-doped host lattices complement each other to obtain white light because of additive color mixing. Therefore, the process of the Eu2+ to Mn2+ energy transfer has been found in many systems and the corresponding mechanism has been investigated for a long time.22–24 Earlier reports on Eu2+–Mn2+ co-doped phosphors that realize white light emission include Mg2Al4Si5O18, Ba1.3Ca0.7SiO4, BaCa2Si3O9, and NaScSi2O6, etc., on account of their stabilization of ionic charge, low price, and excellent thermal stability in comparison with other phosphors.25–28 Besides, phosphate-based phosphors with multi-sites structure have attracted much attention and reported continuously, such as Na2.5Y0.5Mg7(PO4)6:Eu2+,Tb3+,Mn2+,24 Ca9Ce(PO4)7:Eu2+,Mn2+.29 Moreover, because of fluorine atom exhibits strongest attractive electron ability and has the largest electronegative, phosphors which contain fluorine atoms usually show good luminescence properties and good stability. For instance, Ca9Mg(PO4)6F2:Eu2+,Mn2+ phosphor16 and Sr5(PO4)4F:Eu2+,Mn2+ phosphor,30 etc. These phosphors display excellent properties and promising value in lighting applications when built into activator ions.
In this work, a series of blue to orange phosphors Sr6Ca4(PO4)6F2:Eu2+,Mn2+ phosphors (SCPF:Eu2+,Mn2+) irradiated by UV excitation, which were prepared by conventional solid state routine. The structural characteristics, luminescence behaviors of excitation and emission spectra, and luminescence quenching properties were investigated. In comparison with Sr5(PO4)3F:Eu2+,Mn2+ phosphors, the multi-Sr2+/Ca2+ sites in SCPF host make it possible to provide different crystal environment to Eu2+/Mn2+ ions and give rise to about 10 nm spectral red-shift of Mn2+, which is more suitable for w-LEDs upon n-UV chip excitation. While in Ca5(PO4)3F:Eu2+,Mn2+ phosphors, similar emission behaviors can be obtained upon n-UV excitation, but only near white-light-emitting can be obtained.31 This present work developed a new single phase, color-tunable Eu2+/Mn2+-doped silicate phosphor and white-light-emitting can be realized in SCPF:Eu2+,Mn2+ phosphors with the suitable color temperature value of 6662 K upon 365 nm excitation, which will match well with n-UV LEDs. In addition, all of results obtained from the experimental data and theoretical calculation are thoroughly and systematically and it can be beneficial to understand the mechanism of Eu/Mn co-doped phosphors.
2 Synthesis and characterization
2.1 Sample synthesis
The designed phosphors with a composition of (SCPF):xEu2+ (x = 0–0.06) and (SCPF):0.02Eu2+,yMn2+ (x = 0–0.8) powers were prepared through a high temperature solid state reaction. Power samples of SrCO3 (A.R.), CaCO3 (A.R.), CaF2 (A.R.), NH4H2(PO4)2 (A.R.), MnCO2 (A.R.), and Eu2O3 (99.99%) were used as the precursor materials and mixed them thoroughly for 3 h using a ball mill to obtain homogeneous samples. Afterwards, the raw materials were transferred to alumina crucibles, with subsequently sintering at 1250 °C for 4 h in reducing atmosphere, and then the as-prepared samples were cooled down to normal room temperature. Finally, all the phosphors were ground once again for further analysis.
2.2 Characterization and computational details
The structure and phase purity of as-prepared samples were analyzed by using powder X-ray diffraction (XRD) and XRD patterns are performed on D8 Focus diffractometer (Bruker) in the range from 10° to 80°, with graphite monochromatized CuKα radiation (λ = 0.15405 nm). The room temperature excitation spectra (PLE) and emission spectra (PL) were measured using a Hitachi F-4600 fluorescence spectrophotometer, which was equipped with a 150 W xenon lamp as the excitation source. Decay curves were measured on a spectrophotometer (FLS920) equipped with both a xenon lamp (450 W) and a 450 nm pulsed laser as the lighting source. UV-Vis diffuse reflectance spectra (DRS) were carried out with a UV-Vis spectrophotometer (TU-901) using BaSO4 as reference. The quantum yields (QY) of the samples were measured with an integrating sphere and a FLS 920 fluorescence spectrophotometer. The temperature dependence of PL spectra was measured by using the FS-2 system and the temperature ranging from 303 K to 463 K was controlled using a homemade temperature controlled system.
To investigate the electronic structures of pure SCPF, the crystals' optimization was performed in the CASTEP program using the generalized gradient approximation (GGA) under the Perdew–Burke–Ernzerhof (PBE) formulation. During the geometry optimization, the atomic positions were optimized. The kinetic cutoff energy was 480 eV, and a K-point sampling scheme of a 5 × 5 × 5 Monkhorst–Pack grid was used. The convergence tolerance for geometry optimization was 5.0 × 10−6 eV per atom, the maximal ionic Hellmann–Feynman force was 1.0 × 10−2 eV Å−1, the stress tensor was 2.0 × 10−2 GPa and the maximal displacement being within 5.0 × 10−4 Å.
3 Results and discussion
3.1 Phase and structure
Fig. 1 shows the standard data for SCPF (JCPDS#72-2399) and as-prepared samples of SCPF:0.02Eu2+,yMn2+ (a–e) with different Mn2+ content. All diffraction peaks of the XRD pattern were coincided well with that of the standard data of SCPF and the series samples can be indexed as the pure phase, which indicated that different doping concentration does not result in any other phase.
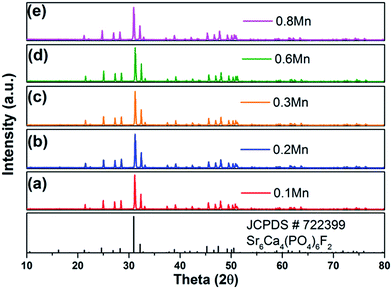 |
| Fig. 1 XRD patterns of as-prepared SCPF:0.02Eu2+,yMn2+ (y = 0.1–0.8) samples and the standard data for SCPF (JCPDS#72-2399). | |
SCPF crystallizes in a hexagonal structure with space group P63(173) and three kinds of sites exist in per unit cell. The dimensions of the unit cell are a = b = 9.6300 Å, c = 7.2200 Å, and V = 579.86 Å3. A suitable viewing direction from the c axis is shown in Fig. 2(a). From the c-direction, the basic structural feature of the SCPF crystal includes CaO6, SrO8, and Ca/SrO5F polyhedra are depicted. The polyhedral of Ca2+ and Sr2+ is exhibited individually in Fig. 2(b), which is consisting of the regular octahedron of Ca1, the dodecahedron of Sr2, and the distorted octahedron of Ca3/Sr3. Based on the consideration of the ionic radius, charge and coordination, when the substitution occurs, Eu2+ ions prefer to occupy all the sites of Ca/Sr. Nevertheless, it worth noting that Mn2+ is likely to substitute Ca1 and Ca3/Sr3 sites, due to the limitation of the coordination number and the corresponding data is shown in Table 1.
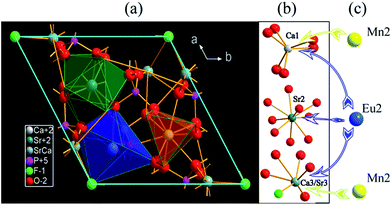 |
| Fig. 2 Crystal structure of SCPF in a hexagonal system with space group P63(173). | |
Table 1 Cations in the lattice: coordination numbers (CN) and radii
Ion |
P5+ |
Ca2+ |
Sr2+ |
Eu2+ |
Mn2+ |
CN |
4 |
6 |
9 |
6 |
9 |
Radii (Å) |
0.38 |
1.18 |
1.26 |
1.18 |
1.30 |
1.17 |
0.83 |
No |
The calculated atomic band structure, and total density of states (TDOS), as well as partial density of states (PDOS) for SCPF are shown in Fig. 3. The direct band gap of SCPF is about 5.182 eV from the top of the valence band (VB) to the bottom of the conduction band (CB) at the G point. The density of states of SCPF was calculated and shown in Fig. 3(b), which is beneficial to understand the composition of the energy band. It can be observed that the valence band is dominated by O 2p states and the conduction band of SCPF is composed of Sr 5s and Sr 3d states. Additionally, the partial density of states of P and F states contributes to a small extent to the valence/conduction band. With such a large band gap, the SCPF host may provide a suitable band gap for Eu2+, which the energy level of the 4f65d → 4f7 transition has small influence on the CB and VB.32
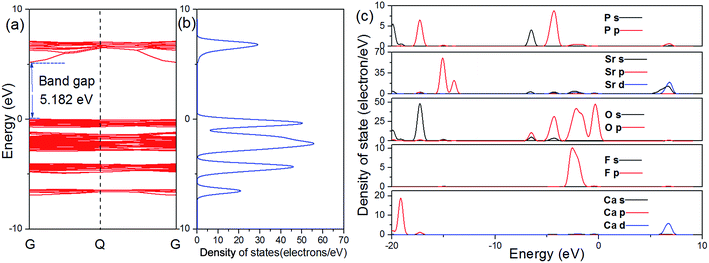 |
| Fig. 3 Band structure (a), total density of states (b) and partial density of states of SCPF (c). | |
3.2 Spectroscopy properties
3.2.1 Diffuse spectra of SCPF:xEu2+ (x = 0–0.06). The absorption of the host lattice derives from the state electrons were excited from VB to CB. Fig. 4 shows reflection spectra of the SCPF host and SCPF:xEu2+ (x = 0–0.06) phosphors. The host shows an obvious reflection from 200 to 800 nm. Notably, when the Eu2+ dopants were introduced at 1%, it can clearly be observed that a sharply enhanced broad reflection band arranging from 200 to 420 nm and a weak declined broad reflection band from 420 nm to 700 nm appeared. With further increasing concentration of Eu2+, the refractive index of the series samples decrease continuously from x = 0.01 to 0.04, which was attributed to the 4f7–4f65d1 absorption of Eu2+. However, once the Eu2+ content is more than 4%, the index of refraction of Eu2+ becomes strengthened.
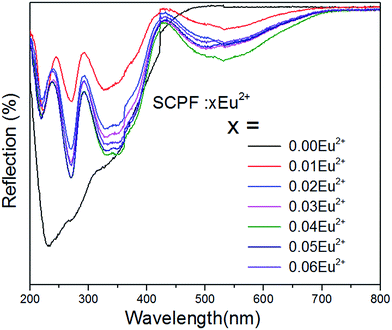 |
| Fig. 4 Reflection spectra of SCPF:xEu2+ accompanying with different contents of Eu2+ (0–0.06), respectively. | |
3.2.2 PL spectra and energy transfer process of SCPF:Eu2+ and SCPF:Eu2+,Mn2+. Fig. 5(a) displays the PL/PLE spectra of SCPF:0.02Eu2+ phosphor. It can be seen that the PLE shows a broad band, which is due to the 4f7–4f65d1 transition of Eu2+ ions. Upon irradiation at 295 or 365 nm, the emission spectra of SCPF:0.02Eu2+ exhibits a broad symmetric band from 400 to 550 nm, with its emission peak at 450 nm. Fig. 5(b) shows the relative emission spectra of various Eu2+ concentrations excited at 365 nm. The PL intensity of Eu2+ increases initially with increasing its concentration until the Eu2+ content reaches at x = 0.02. However, once Eu2+ content is further increased, the emission intensity of Eu2+ decreased, which is because of the typical concentration quenching effect. Notably, even the addition of Eu2+ is 0.05 mol, its emission intensity is closely to that of the optimal concentration (0.02 mol) of Eu2+. Herein, the critical distance between Eu2+ ions for concentration quenching was calculated by the following equation:33 |
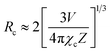 | (1) |
where V represents the volume of the unit cell, χc represents the critical concentration of the activator, and Z is the number of formula units. For the SCPF host, Z = 1, χc = 0.02, and V = 579.86 Å. Thus, the calculated Rc = 38.12 Å. In generally, Rc is 5 Å typically belongs to the exchange interaction in a forbidden transition. Therefore, the type of energy transfer does not belong to exchange interactions but to electric multi-pole to multi-pole interactions.
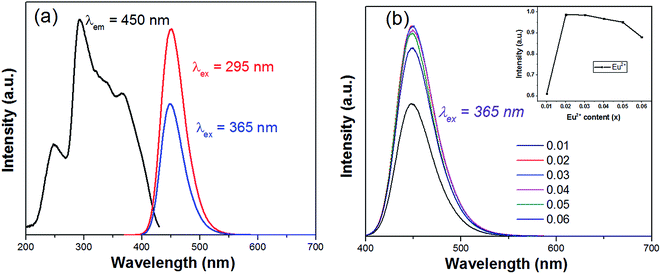 |
| Fig. 5 (a) PLE/PL spectra of SCPF:0.02Eu2+; (b) emission spectra of different Eu2+ contents under 365 nm excitation. | |
In Fig. 6(a), the emission spectra and excitation spectra of SCPF:0.02Eu2+ are presented, while that of SCPF:0.6Mn2+ are shown in Fig. 9(b). The PL spectra of SCPF:0.02Eu2+ exhibits broad band from 400 to 520 nm peaking at 450 nm, while the corresponding excitation spectra of SCPF:0.6Mn2+ is composed of several sharp lines originated from the spin-forbidden transitions from 6A1(S) to 4T1(P) (319 nm), 4E(D) (343 nm), 4T2(D) (362 nm), (4A1(G), 4E(G)) (406 nm), 4T2(G) (433 nm) and 4T1(G) (470 nm), etc., which is covering the region of 300 to 500 nm. The effective spectral overlap areas of PL of Eu2+ and PLE of Mn2+ ensure the existence of the energy transfer (ET) between Eu2+ and Mn2+ (marked as yellow). Additionally, the corresponding excitation spectra of co-doped samples (Eu2+ and Mn2+) were monitored at characteristic emission wavelengths of Eu2+ (450 nm) and Mn2+ (570 nm) are displayed in Fig. 6(c). In addition, it was clearly seen that the shape of excitation of Mn2+ was similar to that of Eu2+, which means Mn2+ could be radiated more effectively under the sensitization of Eu2+.
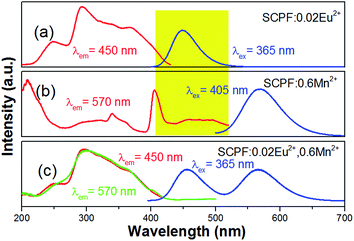 |
| Fig. 6 (a, b) PLE/PL spectra of SCPF:0.02Eu2+ and 0.6Mn2+, respectively; (c) PLE (monitored at 450 and 570 nm, respectively) and PL spectra of SCPF:0.02Eu2+,0.6Mn2+. | |
To further investigate the energy transfer between Eu2+ and Mn2+ in SCPF, the PL decay curves of the series samples are shown in Fig. 7(a). It can be seen that the decay curves of SCPF:0.02Eu2+,yMn2+ (y = 0.1–0.8) are well fitted with a typical double-exponential function:34
|
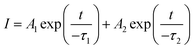 | (2) |
where
I represents the emission intensity;
t represents the time;
A1 and
A2 stand for constant;
τ1 and
τ2 are regarded as the short and long lifetimes for the exponential component, respectively. The lifetimes were calculated as follows:
|
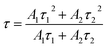 | (3) |
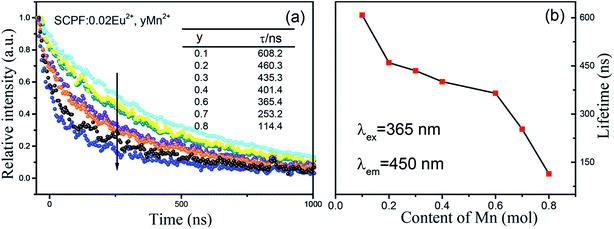 |
| Fig. 7 Decay curves of the series SCPF samples monitored at 450 nm (a) and the corresponding lifetimes of the Mn2+ content plotted in (b). | |
Calculated decay times of Eu2+ for SCPF:0.02Eu2+,yMn2+ samples varied from 608.2 to 114.4 ns when monitored at 450 nm (λex = 365 nm), showing a continuously decline. The corresponding lifetimes of the Mn2+ co-doped samples are plotted in Fig. 7b, which confirm the ET from Eu2+ to Mn2+.
Fig. 8(a) shows the detailed PL spectra of the series samples. When irradiated at 365 nm, two broad bands with emission peak at 450 nm and 570 nm are detected in Eu2+/Mn2+ co-doped samples with the quantum efficiency is 9.22%. With increasing the Mn2+ content, a continuous intensity decrease occurs in the emission band of Eu2+ (450 nm), while the emission intensity of Mn2+ (4T1(4G)–6A1(6S)) increases until the y = 0.6, and then declines slightly, which was ascribed to concentration quenching of Mn2+. The inset picture of Fig. 8(a) shows the efficiency of the ET process from Eu to Mn ions, and the ET efficiency (ηT) can be estimated by the follow formula:35
|
 | (4) |
where
I and
I0 are the emission intensities for the samples in the presence and absence of Mn
2+, respectively. The efficiency of ET was calculated to be 87.8% when
y = 0.6. Additionally, the critical distance based on the energy transfer from Eu
2+ to Mn
2+ (
REu–Mn) could be calculated through the concentration quenching method. The average distance
REu–Mn suggested by Blasse can be expressed as follows:
7 |
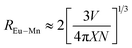 | (5) |
where
N is the sum number molecules in the unit cell,
V is the unit cell volume, and
X is the total concentration of the activator and sensitizer. For the above formula, the calculated result is 11.53 Å, which is larger than 5 Å. Therefore it is the electric multi-polar interaction instead of the exchange interaction that dominates in SCPF:0.02Eu
2+,
yMn
2+.
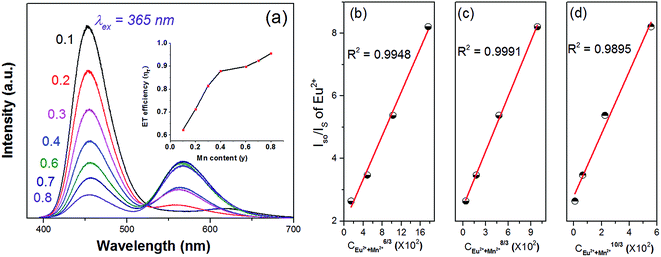 |
| Fig. 8 (a) PL spectra of SCPF:0.02Eu2+,yMn2+ (y = 0–0.8) with the ET efficiency plotted in the inset; dependence of I0/I (Eu2+) on CEu2++Mn2+6/3, CEu2++Mn2+8/3 and CEu2++Mn2+10/3 and the linear fitting results (b)–(d). | |
Sequentially, on the base of Dexter's energy transfer equation for multi-polar interactions, the type of energy transfer can be obtained through the spectral overlap method:9
where
I0 and
I represent the luminescence intensities for samples in the absence and the presence of Mn
2+, respectively, and
C is the total dopant concentration of Eu
2+ and Mn
2+. Using
eqn (6), the calculated results (
n = 6, 8, 10), which correspond to the exchange interaction for dipole–dipole, dipole–quadrupole, and quadrupole–quadrupole interactions, respectively, are depicted in
Fig. 8(c and d). A good linear correlation is obtained when
n = 8, indicating that the dipole–quadrupole mechanism dominated preferentially for the Eu
2+–Mn
2+ energy transfer. According to the spectral overlap theory,
REu–Mn could be also calculated by the formula below:
2,36 |
 | (7) |
where
Rc represents the critical distance, could be calculated using the emission wavelength of Eu
2+ (4500 Å),
fq stands for the oscillator strength of the involved absorption transition of the sensitizer (10
−10),
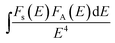
is the spectral overlap between the normalized photoluminescence spectra of the Eu
2+ emission
FS(
E) and the Mn
2+ excitation
FA(
E). Correspondingly, the critical distance was estimated to be 9.43 Å, which is basically in good agreement with the result obtained by the quenching method described. From the above discussion (in the part of
eqn (4)–(7)), it can be better understand the ET process through the ET related calculations.
The CIE coordinates of the series of samples upon 365 nm excitation, which are marked as blacked dots, are portrayed in Fig. 9(a). It can be seen that the CIE chromaticity coordinates move from blue to yellow as the amount of Mn2+ adds from 0 to 0.8 and the color images of SCPF:0.02Eu2+ and SCPF:0.02Eu2+,0.6Mn2+ are given in Fig. 9(b) and (c). The corresponding color temperature (CCT) values of the series samples are demonstrated in Table 2. With the changes of Mn2+ content, a warm white light emission can be obtained with a CCT of 6662 K and CIE coordinate (0.2996, 0.3118).
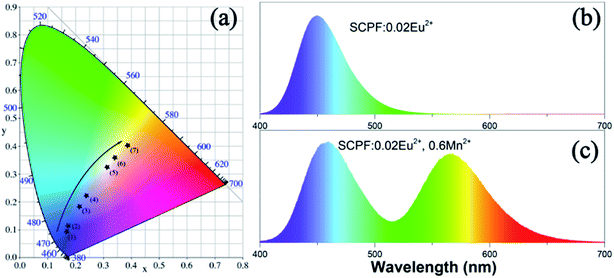 |
| Fig. 9 (a) CIE coordinates and luminescent digital photographs of the SCPF:0.02Eu2+,yMn2+ samples under 365 nm excitation; magnifications of the emission spectra of (b) SCPF:0.02Eu2+ and (c) SCPF:0.02Eu2+,0.6Mn2+. | |
Table 2 Comparison of the CIE chromaticity coordinates and CCT (K) for SCPF:0.02Eu2+,yMn2+ phosphors excited at 365 nm
Sample no. |
Sample composition (n) |
CIE coordinates (x, y) |
CCT (K) |
1 |
y = 0.1 |
(0.1537, 0.0854) |
5765 |
2 |
y = 0.2 |
(0.1633, 0.1077) |
12 836 |
3 |
y = 0.3 |
(0.2026, 0.1746) |
92 948 443 |
4 |
y = 0.4 |
(0.2245, 0.2096) |
86 420 |
5 |
y = 0.6 |
(0.2996, 0.3118) |
6662 |
6 |
y = 0.7 |
(0.3334, 0.3503) |
5160 |
7 |
y = 0.8 |
(0.3803, 0.3956) |
4065 |
4 Conclusions
In summary, a series of single-component color tunable SCPF:Eu2+,Mn2+ phosphors were synthesized through high temperature solid state reaction. The obtained phosphors of SCPF:Eu2+,Mn2+ have broad excitation bands ranging from 200 to 400 nm which match the available UV chips. The emission spectra consists of two emission bands (centered at 450 and 570 nm, respectively) by precisely controlling the ratio of Eu2+ to Mn2+. The emission color of phosphors SCPF:Eu2+,Mn2+ can be tuned from blue to yellow and the corresponding color hue can be changed from blue (0.1537, 0.0854) to white (0.2996, 0.3118) with CCT at 6662 K. Using the concentration quenching method and the spectral overlap method, the critical distance REu–Mn was calculated to be 11.53 Å and 9.43 Å, respectively. The energy transfer mechanism from the Eu2+ to Mn2+ ions has been demonstrated to be resonant type via dipole–quadrupole interaction. The investigation results indicate that the SCPF:0.02Eu2+,0.6Mn2+ phosphor might be a white-emitting phosphor for UV-excited WLEDs.
Conflicts of interest
There are no conflicts to declare.
References
- G. Li, Y. Zhang, D. Geng, M. Shang, C. Peng, Z. Cheng and J. Lin, ACS Appl. Mater. Interfaces, 2012, 4, 296–305 CAS.
- H. Zhou, Y. Jin, M. Jiang, Q. Wang and X. Jiang, Dalton Trans., 2015, 44, 1102–1109 RSC.
- H. Liu, Y. Luo, Z. Mao, L. Liao and Z. Xia, J. Phys. Chem. C, 2014, 2, 1619 CAS.
- Y. Liu, C. Zhang, Z. Cheng, Z. Zhou, J. Jiang and H. Jiang, Inorg. Chem., 2016, 55, 8628–8635 CrossRef CAS PubMed.
- Y. Zhu, L. Huang, R. Zou, J. Zhang, J. Yu, M. Wu, J. Wang and Q. Su, J. Mater. Chem. C, 2016, 4, 5690–5695 RSC.
- Y. Huang and H. J. Seo, Mater. Lett., 2015, 156, 86–89 CrossRef CAS.
- M. Jiao, Y. Jia, W. Lu, W. Lv, Q. Zhao, B. Shao and H. You, Dalton Trans., 2014, 43, 3202–3209 RSC.
- X. Y. Liu, H. Guo, S. Ye, M. Y. Peng and Q. Y. Zhang, J. Mater. Chem. C, 2015, 3, 5183–5191 RSC.
- M. Shang, G. Li, X. Kang, D. Yang, D. Geng and J. Lin, ACS Appl. Mater. Interfaces, 2011, 3, 2738–2746 CAS.
- R. Shi, G. Liu, H. Liang, Y. Huang, Y. Tao and J. Zhang, Inorg. Chem., 2016, 55, 7777–7786 CrossRef CAS PubMed.
- Y. Cao, J. Ding, X. Ding, X. Wang and Y. Wang, J. Mater. Chem. C, 2017, 5, 1184–1194 RSC.
- M. Jiao, Y. Jia, W. Lü, W. Lv, Q. Zhao, B. Shao and H. You, J. Mater. Chem. C, 2014, 2, 90–97 RSC.
- F. Kang, Y. Zhang and M. Peng, Inorg. Chem., 2015, 54, 1462–1473 CrossRef CAS PubMed.
- Y. Liu, G. Liu, J. Wang, X. Dong and W. Yu, Inorg. Chem., 2014, 53, 11457–11466 CrossRef CAS PubMed.
- H. Zhu, Z. Xia, H. Liu, R. Mi and Z. Hui, Mater. Res. Bull., 2013, 48, 3513–3517 CrossRef CAS.
- K. Li, D. Geng, M. Shang, Y. Zhang, H. Lian and J. Lin, J. Phys. Chem. C, 2014, 118, 11026–11034 CAS.
- W. R. Liu, C. H. Huang, C. W. Yeh, J. C. Tsai, Y. C. Chiu, Y. T. Yeh and R. S. Liu, Inorg. Chem., 2012, 51, 9636–9641 CrossRef CAS PubMed.
- J. Ding, Q. Wu, Y. Li, Q. Long, C. Wang and Y. Wang, Dalton Trans., 2015, 44, 9630–9636 RSC.
- H. Wang, L. Wu, H. Yi, B. Wang, L. Wu, Y. Gu and Y. Zhang, Dalton Trans., 2015, 44, 1427–1434 RSC.
- L. Wu, B. Wang, Y. Zhang, L. Li, H. R. Wang, H. Yi, Y. F. Kong and J. J. Xu, Dalton Trans., 2014, 43, 13845–13851 RSC.
- J. Cheng, P. Li, Z. Wang, Y. Sun, Q. Bai, Z. Li, M. Tian, C. Wang and Z. Yang, J. Mater. Chem. C, 2017, 5, 127–133 RSC.
- Y. Sun, P. Li, Z. Wang, J. Cheng, Z. Li, C. Wang, M. Tian and Z. Yang, J. Phys. Chem. C, 2016, 120(36), 20254–20266 CAS.
- X. Chen, P. Dai, X. Zhang, C. Li, S. Lu, X. Wang, Y. Jia and Y. Liu, Inorg. Chem., 2014, 53, 3441–3448 CrossRef CAS PubMed.
- Y. Xu, X. Li, W. Feng, W. Li and K. Zhang, Dalton Trans., 2016, 45, 3983–3991 RSC.
- J. Chen, Y. Liu, M. Fang and Z. Huang, Inorg. Chem., 2014, 53, 11396–11403 CrossRef CAS PubMed.
- W. Lv, M. Jiao, Q. Zhao, B. Shao, W. Lu and H. You, Inorg. Chem., 2014, 53, 11007–11014 CrossRef CAS PubMed.
- M. Muller and T. Justel, Dalton Trans., 2015, 44, 10368–10376 RSC.
- Z. Xia, Y. Zhang, M. S. Molokeev and V. V. Atuchin, J. Phys. Chem. C, 2013, 117, 20847–20854 CAS.
- C. Wang, P. Li, Z. Wang, Y. Sun, J. Cheng, Z. Li, M. Tian and Z. Yang, Phys. Chem. Chem. Phys., 2016, 18, 28661–28673 RSC.
- Y. Feng, J. Huang, L. Liu, J. Liu and X. Yu, Dalton Trans., 2015, 44, 15006–15013 RSC.
- T. Wanjun and Z. Fen, Eur. J. Inorg. Chem., 2014, 2014, 3387–3392 CrossRef CAS.
- N. Zhang, C. Guo, J. Zheng, X. Su and J. Zhao, J. Phys. Chem. C, 2014, 2, 3988 CAS.
- Z. Xia and R.-S. Liu, J. Phys. Chem. C, 2012, 116, 15604–15609 CAS.
- X. Zhang, L. Zhou, Q. Pang, J. Shi and M. Gong, J. Phys. Chem. C, 2014, 118, 7591–7598 CAS.
- T. Li, P. Li, Z. Wang, S. Xu, Q. Bai and Z. Yang, Dalton Trans., 2015, 44, 16840–16846 RSC.
- K. Li, H. Lian, M. Shang and J. Lin, Dalton Trans., 2015, 44, 20542–20550 RSC.
|
This journal is © The Royal Society of Chemistry 2017 |
Click here to see how this site uses Cookies. View our privacy policy here.