DOI:
10.1039/C7RA07247C
(Paper)
RSC Adv., 2017,
7, 38458-38462
A benzimidazole functionalized NDI derivative for recyclable fluorescent detection of cyanide in water†
Received
30th June 2017
, Accepted 30th July 2017
First published on 4th August 2017
Abstract
A fluorescent chemosensor (L2) has been designed and synthesized via a simple one step reaction through rationally combining benzimidazole and naphthalene diimide (NDI) moieties together. The structure of L2 has been confirmed by single crystal X-ray diffraction. The sensor L2 shows a highly selective and sensitive fluorescence response for CN−. Upon the addition of a CN− water solution into the DMSO solution of sensor L2, the solution of L2 shows an instant fluorescence enhancement and other anions couldn't interfere in the CN− detection process. The CN− sensing process shows reversible properties by adding H+ into the sensor L2 solution treated by CN−. The detection limit of the sensor towards CN− is 8.32 × 10−7 M, which could be distinguished by the naked eye in a UV lamp. The sensor L2 could serve as a practical and convenient recyclable test kit to detect CN−.
1. Introduction
As we all know, ions play a fundamental role in many chemical, biological, medical and technological processes.1–9 Cyanide ions (CN−) are one of the most toxic anions, being extremely harmful to the environment and human health.10–12 When cyanide enters the body by oral, inhalation or dermal exposure, it exerts its acute effects by complexing with ferric iron atoms in metalloenzymes, resulting in histotoxic anoxia through inhibition of cytochrome c oxidase. The maximum permissive level of cyanide in drinking water is therefore set at as low as 1.9 mM by the World Health Organization (WHO).13 However, large quantities of cyanide salts are widely used in industrial settings such as metallurgy, electroplating, and the synthesis of fine chemicals. In addition, a higher level of cyanide can also be accumulated through the consumption of certain foods and plants.14 Therefore, much interest has been sparked in the design of new methods to monitor CN− in biological and environmental samples.
Thanks to the enthusiastic efforts of scientists, a large number of good sensors for cyanide have been invented.15,16 Among the various sensors, fluorescent chemosensors present numerous advantages, including high sensitivity, low cost, and easy operation.17–19 Several fluorescent chemosensor systems for CN− detection reported to date are based on the mechanism of coordination,20–24 hydrogen-bonding interactions,25–27 nucleophilic addition reactions,28–36 sonogashira cross-coupling reaction37–39 and so on. However, most of the CN− sensors often employ sophisticated structures and require complicated synthetic steps and high temperature or a long reaction time for detection of CN−. Thus, developments of simple and high efficiency fluorescent chemosensor for detecting CN− are very necessary.
In view of these and as a part of our research interests in ion recognition,40–48 we attempt to develop an easy-to-make and efficient fluorescent chemosensor which can sense CN− with specific selectivity and high sensitivity. Herein, we report a benzimidazole functionalized NDI-based49 fluorescent chemosensor L2 which could be obtained from an easy one step reaction (Scheme 1). The sensor L2 could efficiently detect CN− in water under mild conditions via a deprotonation and electric charge transferring mechanism, which could be used for highly selective and sensitive fluorescence enhancement detection of cyanide in aqueous media (Scheme 1).
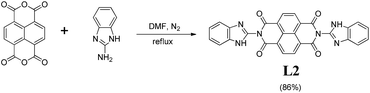 |
| Scheme 1 Synthesis of the sensor molecule L2. | |
2. Experimental
The fluorescent chemosensor L2 was synthesized through a simple one-step reaction (Scheme 1). 1,4,5,8-naphthalenetetracarboxylic dianhydride (2.68 g, 10 mmol) and 2-aminobenzimidazole (2.66 g, 20 mmol) were mixed in DMF (25 mL) in a round-bottom flask (50 mL). The reaction mixture was stirred at 120 °C for 24 h. After cooling to room temperature, the mixture was poured into H2O (15 mL). The precipitate was collected by filtration, washed with ethanol (20 mL × 3), and then dried in vacuum, give a yellow powder compound L2. Yield: 4.43 g. 86%. mp > 300 °C. 1H NMR (600 MHz, DMSO-d6, Fig. S1†) δ 12.68 (s, 2H), 8.83 (s, 4H), 7.69 (dd, J = 12.0, 6.0 Hz, 4H), 7.33 (dd, J = 12.0, 6.0 Hz, 4H). 13C NMR (150 MHz, DMSO-d6, Fig. S2†) 13C NMR (150 MHz, DMSO-d6) δ 162.95, 142.27, 141.16, 134.38, 131.68, 127.59, 126.97, 123.70, 122.31, 119.85, 112.52. IR (KBr, Fig. S3†) v: 1330 (–C
N–), 1681 (–C
O), 3509 (–NH) cm−1. MS: ESI m/z for L2 [C28H14N6O4 + H]+ found: 499.01, calcd: 499.11 (Fig. S4†).
The structure of the sensor was further confirmed by single-crystal X-ray diffraction. The single crystal (CCDC: 1553559) was obtained by slowly vaporizing the DMSO solution of L2. As shown in Fig. 1a, in the molecular of L2, the benzimidazole moiety and the naphthalene diimide (NDI) moiety don't on one plan. The dihedral angle of benzimidazole group and naphthalene diimide (NDI) moiety is 99.1°. Moreover, as shown in Fig. 1b, the benzimidazole moiety of one molecular of L2 shows parallel stacking with naphthalene diimide (NDI) moiety on adjacent molecular of L2. The distance between the two plan is 3.62 Å, which indicate that there are intermolecular π–π interaction50 existing among the neighboring L2 molecular. In addition, in the powder the X-ray diffraction (XRD) of L2 (Fig. 2), we observed a sharp diffraction peak with d-spacing of 3.62 Å at 2θ = 24.42°, which suggested that π–π stacking exists between the between molecular of L2.
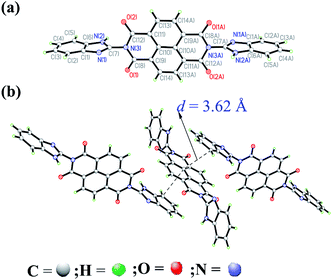 |
| Fig. 1 Different perspectives of L2's crystal structure. | |
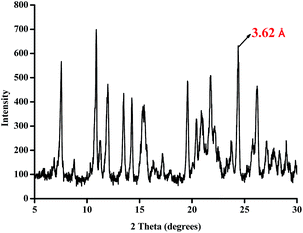 |
| Fig. 2 XRD diagram of the sensor of L2. | |
3. Results and discussion
In order to investigate the anion recognition abilities of the sensor L2 in water, we carried out a series of host–guest recognition experiments. The recognition profiles of the chemosensor L2 toward various anions, including F−, Cl−, Br−, I−, AcO−, H2PO4−, HSO4−, ClO4− and CN−, were primarily investigated using fluorescence spectroscopy. As shown in Fig. 3, in the fluorescence spectrum of L2 DMSO solution, the maximum emission of L2 appeared at 510 nm in water when excited at λex = 455 nm. When 10 equiv. of CN− water solution was added to the DMSO solution of sensor L2, the fluorescence emission band at shifted to 550 nm and shows obvious enhancement. The apparent fluorescence emission change could be distinguished by the naked eye under UV lamp (Fig. 3). To validate the selectivity of sensor L2, the same tests were also conducted using F−, Cl−, Br−, I−, AcO−, H2PO4−, HSO4−, ClO4− ions, and none of these anions induced any significant changes in the fluorescent spectrum of the sensor (Fig. 4). Therefore, the sensor L2 could selectively sense CN− in water solution.
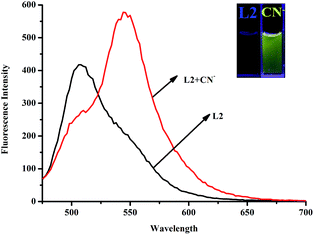 |
| Fig. 3 Fluorescence spectra of L2 (20 mM) upon excitation at 455 nm DMSO/H2O (9 : 1, v/v) before and after addition of CN− (50 equiv.) water solution. Inset: Photographs showing the change in the fluorescence of L2 after addition of CN− (10 equiv.) in DMSO/H2O (9 : 1, v/v) HEPES buffered solution. | |
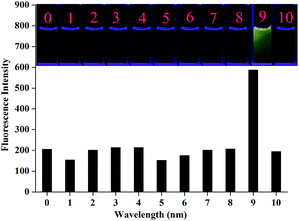 |
| Fig. 4 Histogram of fluorescence emission of L2 (c = 2 × 10−5 M) in the presence of various anions (50 equiv., λex = 455 nm) in DMSO/H2O (9 : 1 v/v) HEPES buffered solution. Inset: Color changes observed for L2 upon the addition of various anions in water. From 0 to 10: L2, Cl−, Br−, I−, H2PO4−, F−, AcO−, HSO4−, ClO4−, CN−, SCN−. | |
To further investigate the CN− detection property of the sensor L2, the fluorescence emission spectral variation of L2 in DMSO/H2O (9
:
1, v/v) HEPES buffered solution was monitored during titrations with different concentrations of CN− in water from 0 to 25 equivalents (Fig. 5). With an increasing amount of CN−, the emission peak at 510 nm gradually shows a bathochromic effect, however, the emission peak at 550 nm increased. The scatter plot indicated that the reaction basic achieved balance when the concentration of CN− ions increased to 25.0 equivalent. Furthermore, as shown in Fig. S5,† the detection limit of the fluorescent spectrum changes calculated on the basis of 3δ/s (ref. 51) is 8.82 × 10−7 mol L−1 for CN−, which is far lower than the WHO guideline of 1.9 μmol L−1 cyanide.13
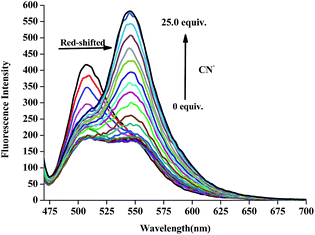 |
| Fig. 5 Fluorescence spectra of L2 in the presence of different concentrations of CN− in DMSO/H2O (9 : 1, v/v) HEPES buffered solution. | |
To further exploit the utility of the chemosensor L2 as an anion-selective sensor for CN−, competitive experiments were carried out in the presence of 10 equiv. of CN− and 50 equiv. of various anions in DMSO/H2O (9
:
1, v/v) HEPES buffered solution. As shown in Fig. 6, none of the competing anions interfered in the detection of CN−. This result displays the highly selectivity of the chemosensor L2 toward CN− over the other anions analytes mentioned above.
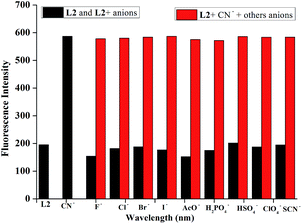 |
| Fig. 6 Fluorescence intensity at 587 nm of L2 (c = 2 × 10−5 M) exposed to 50 equiv. various anions and to the mixture of 10 equiv. CN− with other 50 equiv. ions in DMSO/H2O (9 : 1, v/v) HEPES buffered solution. | |
The selectivity of L2 to CN− was also examined over the range of pH values. The detection of CN− can work well in the pH range of 6.0–9.0 in HEPES buffered solution in DMSO/H2O (9
:
1, v/v) (Fig. 7).
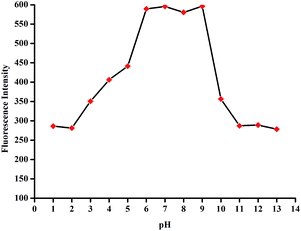 |
| Fig. 7 Influence of pH on the fluorescence of L2–CN− (c = 2.0 × 10−5 M) in HEPES buffered solution in DMSO/H2O (9 : 1, v/v) HEPES buffered solution. (c = 0.01 M, λex = 455). | |
Moreover, the recyclability is very important for chemosensors. Therefore, we carefully investigated the reversibility of the CN− recognition properties by adding H+ into the solution of CN− treated sensor L2. As a result, by alternating the addition of CN− and HClO4, the fluorescence of the sensor shows ‘‘off–on–off’’ changes several cycles with little loss of fluorescence efficiency (Fig. 8). These properties made sensor L2 act as recyclable CN− sensor as well as a fluorescent switch.
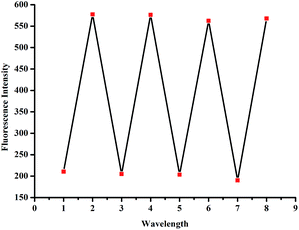 |
| Fig. 8 Fluorescence switching cycles of L2 (c = 2.0 × 10−5 M, λex = 455 nm), controlled by alternating the addition of CN− and HClO4. | |
In addition, it is well known that reaction-based chemosensors always have had the problem of a long response time. In our case, the detection of CN− using L2 was found to be relatively rapid (Fig. 9). After adding the cyanide anion, the fluorescence emission intensity of L2 increased and reached the plateau region in less than 1 min, suggesting that the whole process of the CN− detection might be completed rapidly.
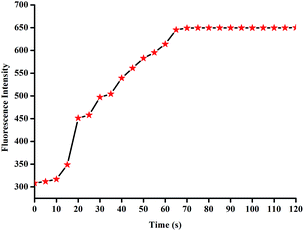 |
| Fig. 9 Fluorescence intensity of a solution of L2 (c = 2.0 × 10−5 M, λex = 455 nm, λem = 550 nm) in DMSO/H2O (9 : 1, v/v) HEPES buffered solution after addition of 50 equivalents CN− in water. | |
In order to further explore the sensing mechanism of sensor L2 to CN−, the 1H NMR titrations were investigated. As shown in Fig. 10, before the addition of cyanide ion, the 1H NMR chemical shifts of the Ha (–NH), Hb, Hc, Hd protons on L2 were at 12.71, 8.86, 7.72 and 7.33 ppm, respectively. Upon the addition of 0.2–2.0 equivalents of cyanide ion, the signal Ha (–NH) was disappeared and the Hb, Hc protons shifted upfield. These observations obviously indicate that the sensor L2 were deprotonated by excess amounts of CN−, which resulted in intramolecular charge transfer. The FT-IR spectroscopy (Fig. S6†) was also support the proposed mechanism. In the FT-IR spectroscopy of L2, a –NH vibration peak appeared at 3509 cm−1, while, upon the addition of 2.0 equiv. CN−, –NH vibration peak disappeared, which indicated that the deprotonation occurred. A possible mechanism for the formation of L2–CN is proposed in Scheme 2.
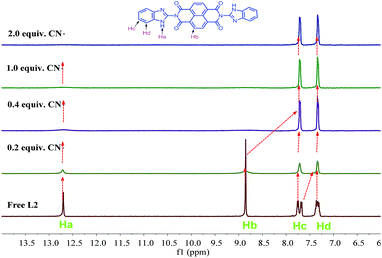 |
| Fig. 10 1H NMR spectra (400 MHz, DMSO-d6) of free L2 and in the presence of CN−. | |
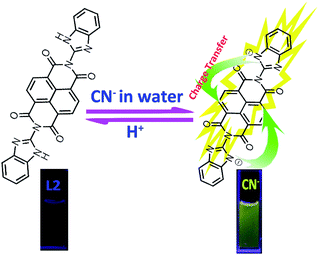 |
| Scheme 2 The possible sensing mechanism of L2 reaction with CN−. | |
4. Conclusions
In summary, a fluorescent chemosensor L2 has been designed and synthesized by an one-step reaction. The sensor L2 for could rapidly detect CN− with high selectivity and sensitivity via a deprotonation and intramolecular charge transfer mechanism. The detection limit of the chemosensor L2 toward CN− was 8.32 × 10−7 mol L−1, other anions couldn't interfere in the CN− detection process. The detection of CN− can work well in the pH range of 6.0–9.0. Moreover, the CN− sensing process shows reversible properties by adding H+ into the sensor L2 solution treated by CN−. The sensor L2 could act as a convenient recyclable test kits to CN−.
Acknowledgements
This study was supported by the National Natural Science Foundation of China (NSFC) (No. 21662031; 21661028; 21574104; 21262032) and the Program for Changjiang Scholars and Innovative Research Team in University of Ministry of Education of China (IRT 15R56).
Notes and references
- C. Caltagirone and P. A. Gale, Chem. Soc. Rev., 2009, 38, 520 RSC.
- K. P. Carter, A. M. Young and A. E. Palmer, Chem. Rev., 2014, 114, 4564 CrossRef CAS PubMed.
- S. V. Krivovichev, O. Mentré, O. I. Siidra, M. Colmont and S. K. Filatov, Chem. Rev., 2013, 113, 6459 CrossRef CAS PubMed.
- N. Busschaert, C. Caltagirone, W. V. Rossom and P. A. Gale, Chem. Rev., 2015, 115, 8038 CrossRef CAS PubMed.
- S. Dong, J. Yuan and F. Huang, Chem. Sci., 2014, 5, 247 RSC.
- Z. Zhang, Q. Zhao, J. Yuan, M. Antonietti and F. Huang, Chem. Commun., 2014, 50, 2595 RSC.
- K. Yang, Y. Pei, J. Wen and Z. Pei, Chem. Commun., 2016, 52, 9316 RSC.
- G. Yu, J. Zhou, J. Shen, G. Tang and F. Huang, Chem. Sci., 2016, 7, 4073 RSC.
- Y. Yao, M. Xue, Z. Zhang, M. Zhang, Y. Wang and F. Huang, Chem. Sci., 2013, 4, 3667 RSC.
- M. A. Holland and L. M. Kozlowski, Clin. Pharmacol., 1986, 5, 737 CAS.
- R. Koenig, Science, 2000, 10, 1737 CrossRef.
- F. J. Baud, Hum. Exp. Toxicol., 2007, 26, 191 CAS.
- Guidelines for Drinking-Water Quality, World Health Organization, Geneva, 2nd edn, 1996, vol. 2 Search PubMed.
- F. Wissing, in Cyanide in Biology, Academic Press, London, 1981 Search PubMed.
- Z. Xu, X. Chen, H. N. Kim and J. Yoon, Chem. Soc. Rev., 2010, 39, 127 RSC.
- M. Xue, X. Zhong, Z. Shaposhnik, Y. Q. Qu, F. Tamanoi, X. F. Duan and J. I. Zink, J. Am. Chem. Soc., 2011, 23, 8798 CrossRef PubMed.
- Y. Yang, Q. Zhao, W. Feng and F. Li, Chem. Rev., 2013, 113, 192 CrossRef CAS PubMed.
- J. H. Hu, J. B. Li, J. Qi and J. J. Chen, New J. Chem., 2015, 39, 843 RSC.
- Y. Shiraishi, M. Nakamura, N. Hayashi and T. Hirai, Anal. Chem., 2016, 88, 6805 CrossRef CAS PubMed.
- L. J. Tang and M. J. Cai, Sens. Actuators, B, 2012, 173, 862 CrossRef CAS.
- S. C. Wei, P. H. Hsu, Y. F. Lee, Y. W. Lin and C. C. Huang, ACS Appl. Mater. Interfaces, 2012, 4, 2652 CAS.
- I. J. Kim, M. Ramalingam and Y. A. Son, Sens. Actuators, B, 2017, 246, 319 CrossRef CAS.
- Y. Y. Guo, X. L. Tang, F. P. Hou, J. Wu, W. Dou, W. W. Qin, J. X. Ru, G. L. Zhang, W. S. Liu and X. J. Yao, Sens. Actuators, B, 2013, 181, 202 CrossRef CAS.
- B. A. Rao, J. Y. Lee and Y. A. Son, Spectrochim. Acta, Part A, 2014, 127, 268 CrossRef CAS PubMed.
- T. B. Wei, G. Y. Wu, B. B. Shi, Q. Lin, H. Yao and Y. M. Zhang, Chin. J. Chem., 2014, 32, 1238 CrossRef CAS.
- V. Kumar, M. P. Kaushik, A. K. Srivastava, A. Pratap, V. Thiruvenkatam and T. N. Row, Anal. Chim. Acta, 2010, 663, 77 CrossRef CAS PubMed.
- M. Shahid, S. S. Razi, P. Srivastava, R. Ali, B. Maiti and A. Misra, Tetrahedron, 2012, 68, 9076 CrossRef CAS.
- Y. H. Jeong, C. H. Lee and W. D. Jang, Chem.–Asian J., 2012, 7, 1562 CrossRef PubMed.
- Y. M. Kim, H. Y. Zhao and F. P. Gabbai, Angew. Chem., 2009, 121, 5057 (Angew. Chem. Int. Ed., 2009, 48, 4957) CrossRef.
- J. Jo, A. Olasz, C. H. Chen and D. Lee, J. Am. Chem. Soc., 2013, 135, 3620 CrossRef CAS PubMed.
- R. Guliyev, S. Ozturk, E. Sahin and E. U. Akkaya, Org. Lett., 2012, 14, 1528 CrossRef CAS PubMed.
- M. Dong, Y. Peng, Y. M. Dong, N. Tang and Y. W. Wang, Org. Lett., 2012, 14, 130 CrossRef CAS PubMed.
- Y. M. Dong, Y. Peng, M. Dong and Y. W. Wang, J. Org. Chem., 2011, 76, 6962 CrossRef CAS PubMed.
- L. Yang, X. Li, J. B. Yang, Y. Qu and J. L. Hua, ACS Appl. Mater. Interfaces, 2013, 5, 1317 CAS.
- G. L. Fu and C. H. Zhao, Tetrahedron, 2013, 69, 1700 CrossRef CAS.
- Y. D. Lin, Y. S. Pen, W. T. Su, K. L. Liau, Y. S. Wen, C. H. Tu, C. H. Sun and T. J. Chow, Chem.–Asian J., 2012, 7, 2864 CrossRef CAS PubMed.
- R. Misra, T. Jadhav, B. Dhokale and S. M. Mobin, Dalton Trans., 2015, 44, 16052 RSC.
- T. S. Reddy, R. Maragani and R. Misra, Dalton Trans., 2016, 45, 2549 RSC.
- R. Maragani, T. S. Reddy and R. Misra, Tetrahedron Lett., 2016, 57, 3853 CrossRef CAS.
- Q. Lin, T. T. Lu, X. Zhu, T. B. Wei, H. Li and Y. M. Zhang, Chem. Sci., 2016, 7, 5341 RSC.
- W. J. Qu, T. B. Wei, Q. Lin, W. T. Li, J. X. Su, G. Y. Liang and Y. M. Zhang, Sens. Actuators, B, 2016, 232, 115 CrossRef CAS.
- J. Chen, Q. Lin, Q. Li, W. T. Li, Y. M. Zhang and T. B. Wei, RSC Adv., 2016, 6, 86627 RSC.
- B. B. Shi, P. Zhang, T. B. Wei, H. Yao, Q. Lin and Y. M. Zhang, Chem. Commun., 2013, 49, 7812 RSC.
- Q. Lin, F. Zheng, L. Liu, P. P. Mao, Y. M. Zhang, H. Yao and T. B. Wei, RSC Adv., 2016, 6, 111928 RSC.
- Q. Lin, P. P. Mao, L. Liu, J. Liu, Y. M. Zhang, H. Yao and T. B. Wei, RSC Adv., 2017, 7, 11206 RSC.
- Y. L. Leng, J. H. Zhang, Q. Li, Y. M. Zhang, Q. Lin, H. Yao and T. B. Wei, New J. Chem., 2016, 40, 8607 RSC.
- W. J. Qu, W. T. Li, H. L. Zhang, T. B. Wei, Q. Lin, H. Yao and Y. M. Zhang, Sens. Actuators, B, 2017, 242, 430 CrossRef.
- Q. Lin, X. Liu, T. B. Wei and Y. M. Zhang, Chem.–Asian J., 2013, 8, 3015 CrossRef CAS PubMed.
- T. S. Reddy, R. Maragani, B. Dhokale, S. M. Mobin and R. Misra, RSC Adv., 2016, 6, 7746 RSC.
- S. Tsuzuki, K. Honda, T. Uchimaru, M. Mikami and K. Tanabe, J. Am. Chem. Soc., 2002, 124, 104 CrossRef CAS PubMed.
- Analytical Methods Committee, Analyst, 1987, 112, 199 RSC.
Footnote |
† Electronic supplementary information (ESI) available. See DOI: 10.1039/c7ra07247c |
|
This journal is © The Royal Society of Chemistry 2017 |
Click here to see how this site uses Cookies. View our privacy policy here.