DOI:
10.1039/C7RA10822B
(Paper)
RSC Adv., 2017,
7, 54178-54187
In vitro and in vivo characterization of a novel long-acting GLP-1 receptor agonist, exendin-4–Fc fusion protein†
Received
30th September 2017
, Accepted 26th October 2017
First published on 24th November 2017
Abstract
Exendin-4 (Ex-4), one of the important glucagon-like peptide-1 receptor (GLP-1R) agonists, has proven to be an effective antidiabetic agent for type 2 diabetes (T2D). However, its therapeutic potential is limited due to its short half-life (t1/2), which is subcutaneously administered twice daily. The aim of this study is to develop a safe, efficacious and longer acting potential anti-diabetic agent. The bioactivity of (Ex-4)2–Fc was examined in U2OS cells and we used molecular docking software to test the stability of the (Ex-4)2–Fc/GLP-1 receptor complexes. The pharmacokinetics of (Ex-4)2–Fc was studied in male Sprague-Dawley (SD) rats and the pharmacodynamics was assessed in various mouse models. The bioactivity of (Ex-4)2–Fc was over 10-fold higher than that of Ex-4–Fc in vitro and the stability of the (Ex-4)2–Fc/GLP-1 receptor complexes was better than that of the Ex-4–Fc/GLP-1 receptor complexes. The pharmacokinetics of (Ex-4)2–Fc showed that it had a more than 200-fold prolongation in plasma half-life compared with that of Ex-4, which was 122 h and 0.56 h, respectively. The treatment with (Ex-4)2–Fc every 6 days, compared to the vehicle control, effectively reduced body weight, decreased food intake, and improved glucose metabolism in high-fat-diet induced obesity (DIO) mice, leptin-deficient ob/ob and leptin receptor-defective db/db mice. Our studies suggest that (Ex-4)2–Fc retains the bioactivity of the GLP-1 receptor agonist with a prolonged half-life in vivo, providing a promising drug candidate for the treatment of type 2 diabetes.
1. Introduction
Obesity and the associated diseases have become a growing global health problem. According to World Health Organization's 2014 report, more than 1.9 billion adults worldwide are overweight and over 600 million are obese.1 Obesity exacerbates dyslipidemia, insulin resistance, hyperinsulinemia, and hyperglycemia.2 There are many anti-obesity drugs and current agents available; however, most of these drugs have treatment-limiting side effects.3 Low toxicity and long-acting drugs are required to be researched and developed to improve life quality. Glucagon-like peptide-1 (GLP-1), a polypeptide consisting of 30 amino acids, is an incretin hormone secreted by L-cell in the distal portion of the intestine in response to food ingestion. GLP-1 also stimulates insulin secretion from the pancreatic islet β-cell.4 As a potential therapeutic agent in the treatment of type II diabetes mellitus (T2DM),5 GLP-1 enables weight control, inhibits gastric emptying, and enhances the glucose disposal; but the endogenous GLP-1 is rapidly degraded by dipeptidyl peptidase IV (DPP-IV) with a half-life of 1–2 min, therefore limiting the native GLP-1 clinical therapeutic potential.6,7 Thus, GLP-1 analogues and their long-acting formulations have been developed.
Exendin-4 (Ex-4) is a 39 amino acid peptide isolated from the salivary glands of the Gila monster lizard (Heloderma suspectum), which shares 53% sequence homology with GLP-1. Unlike GLP-1, Ex-4 is resistant to degradation by DPP-IV,8 so it is a stable peptide. Ex-4 treatment has been demonstrated to promote a significant reduction in high-fat-diet (HFD) induced obesity, food intake, and gastric emptying.9 Although Ex-4 exhibits an extended half-life compared to the native GLP-1, it still requires once- or twice-daily injections necessitating the development of many long-acting analogues.10 For example, bydureon and lixisenatide, two analogues of GLP-1R agonists, were approved by the US Food and Drug Administration (FDA) for the treatment of T2DM in 2012 (ref. 11 and 12) and 2016,13,14 respectively. Bydureon, a long-acting formulation of exenatide, requires once-weekly injection, whereas lixisenatide requires once-daily injection.
The aim of this study is to develop a safe, efficacious and longer acting potential anti-diabetic agent. Currently, several peptide–Fc fusion proteins have been developed15,16 and the protein fusion technologies have contributed to the development of long-acting GLP-1 analogues.17–19 In the current study, we designed a long-acting GLP-1R agonist, exendin-4–IgG4 Fc fusion protein ((Ex-4)2–Fc), in which Ex-4 was fused to a mutated immunoglobulin G4 (IgG4) Fc fragment. Although peptide–Fc fusion technologies have been widely used, no reports have demonstrated that two tandem copies of Ex-4 molecules were fused to a mutant human IgG4 constant heavy-chain. A single subcutaneous (s.c.) injection of (Ex-4)2–Fc significantly prolonged its half-life in plasma (∼122 h) in male Sprague Dawley (SD) rats, potentially allowing once-weekly dosing. In addition, diet-induced obesity (DIO) mice as well as ob/ob and db/db mice with the treatment of (Ex-4)2–Fc, once every six days, effectively decreased body weight and food intake and improved glucose intolerance. Our results suggest that this Fc-fusion protein can prolong the half-life, improve glucose metabolism effectively, and provide a new therapy for diabetes and obesity.
2. Materials and methods
2.1 Design and expression of Ex-4–Fc and (Ex-4)2–Fc
Recombinant DNA fragments of Ex-4–Fc and (Ex-4)2–Fc, encoding the respective fusion proteins, were synthesized by Invitrogen (Shanghai, China). The recombinant DNA fragments were then digested by the restriction enzymes, Nhe I and Xba I (New England Biolabs, Ipswich, MA, USA), and inserted into the pcDNA3.1 vector (Invitrogen, Carlsbad, CA, USA) to obtain pcDNA3.1-Ex-4–Fc and pcDNA3.1-(Ex-4)2–Fc. Chinese hamster ovary (CHO) cells obtained from American Type Culture Collections (ATCC, #CCL-61) were cultured at 37 °C in a 5% CO2 humidified incubator in Iscove's Modified Dulbecco Medium (IMDM) (Gibco, Thermo Fisher, Grand Island, USA) supplemented with 10% fetal bovine serum (FBS) (Gibco, Thermo Fisher, Grand Island, USA). The recombinant plasmids were transfected into the CHO cells with Lipofectamine® 2000 Reagent (Invitrogen, Carlsbad, CA, USA) according to the manufacturer's instructions. The high-yield stable clones were selected by G418 (Gibicol, Thermo Fisher, Grand Island, USA) and adapted in a serum-free medium (Hyclone, GE Healthcare, Piscataway, NJ, USA).
2.2 Purification of fusion proteins
The fusion proteins were produced in a 5 L scale bioreactor (Sartorius, German) using the serum-free medium for 10–12 days. The culture supernatant was concentrated by hollow-fiber ultrafiltration (10 kDa) (GE healthcare). The fusion proteins were obtained by two-step purification with MabSelect affinity chromatography and Q anion-exchange chromatography (GE Healthcare). Then, the fusion proteins were analyzed by SDS-PAGE and western blot, sterile-filtered and stored at −20 °C.
2.3 In vitro activity of (Ex-4)2–Fc
Recombinant U2OS cells derived from human osteosarcoma and stably expressing human GLP-1 receptor obtained from Thermo Fisher Scientific (Thermo, #R04-097-01) were maintained in Dulbecco's Modified Eagle's Medium (DMEM) (Gibco, Thermo Fisher, Grand Island, USA) supplemented with 10% FBS, G418 (0.5 mg mL−1), streptomycin (100 μg mL−1), and penicillin-G (100 U mL−1) under an atmosphere of 5% CO2 at 37 °C. The cells were plated in 96-well plates at a density of 6 × 103 cells per well. After a 3 day maintenance period, the cells were incubated for 30 min with 100 μL of Ex-4–Fc or (Ex-4)2–Fc diluted in DMEM at various concentrations (200, 50, 12.5, 3.125, 0.78, 0.195, 0.049, 0.0122, and 0.003 μg mL−1). The amount of cAMP in the cells was assessed using cAMP Parameter Assay Kit (R&D Systems, Minneapolis, MN).
2.4 Internalization of GLP1R–EGFP stimulated with (Ex-4)2–Fc
Recombinant U2OS cells derived from stably expressing human GLP-1 receptor was fused to the N-terminus of an enhanced green fluorescent protein (EGFP). The cells were plated in 96-well plates at a density of 6 × 103 cells per well. 100 nM Ex-4–Fc or (Ex-4)2–Fc was added to the cell plate and then it was incubated for 1 h at 37 °C in a 5% and 95% humidity incubator, respectively. After 1 h, the cells were washed 4 times with 200 μL PBS per well per wash and then 100 μL 1 μM Hoechst staining solution was added. The cells were incubated at room temperature for 30 min before imaging.
2.5 Molecular docking
The model of the interaction between the extracellular domain of GLP-1 receptor and the fusion proteins of Ex-4–Fc and (Ex-4)2–Fc was performed using the ZDOCK module in Accelrys Discovery studio 3.5 software.
2.6 Analysis of purified (Ex-4)2–Fc
The single chain samples without glycosylation were denatured and reduced using 6 M guanidine hydrochloride in 10 mM dithiothreitol (DTT) at 56 °C for 30 min and then treated with PNGase F.
A 5600+ QTOF mass spectrometer (AB SCIEX, USA) and an ACQUITY UPLC Protein BEH C4 Column (300 Å, 1.2 μm 2.1 mm × 100 mm (Agilent, USA)) were used to measure the molecular weights of (Ex-4)2–Fc fusion protein and its subunits. Approximately 10 μg of each sample was injected into the column at 80% mobile phase A (0.1% FA in water) and 20% mobile phase B (0.1% FA in acetonitrile). After 7 min, the percentage of mobile phase B was increased to 60% for 16 min. The column was thoroughly washed and then equilibrated with 20% mobile phase B before the next injection. Throughout the analysis, the flow-rate was set at 200 μL min−1 and the column temperature was set at 80 °C. The mass spectrometer was run in the positive mode with the following settings: a scan range of 600–5000 m/z, gas temperature of 350 °C, ion source gas of 30 L min−1, and collision energy at 10 eV.
2.7 Animals and diets
All animal care and experimental procedures in this study were in compliance with the guidelines set by the Guide for the Care and Use of Laboratory Animals and approved by the Institutional Animal Care and Use Committee of Sichuan University (Chengdu, China). Six week-old male C57BL/6 mice and male Sprague-Dawley (SD) rats were purchased from Beijing Hua Fu Kang Biological Technology Co., Ltd. (Beijing, China). Male ob/ob and db/db mice were purchased from Model Animal Research Center (MARC) of Nanjing University (Nanjing, China). They were maintained at ambient temperature of 25 ± 1 °C with a constant humidity and a 12 h light/dark cycle and fed ad libitum. C57BL/6 mice were fed either a high-fat diet (HFD) containing 60% of calories as fat (D12492, Research Diets, New Brunswick, NJ, USA) or a standard laboratory diet (STD, Rat & Mouse Maintenance Diet) for 12 weeks and 6 week-old male ob/ob and db/db mice were fed the standard laboratory diet for 2 weeks before treatment.
2.8 Pharmacokinetics in rats
Six week old male SD rats (n = 8 per group) were divided into 4 groups and administered the following via a single s.c. injection: (1) (Ex-4)2–Fc (0.45 mg kg−1); (2) (Ex-4)2–Fc (1.35 mg kg−1); (3) Ex-4 (0.3 mg kg−1); and (4) phosphate buffered saline (PBS) as a vehicle control. The blood samples were collected from retro-orbital venous sinus before the injection and at various time points (0.5, 1, 2, 4, 6, 10 h, 1, 2, 4, 6, 8, 10, 14, and 21 days) after injection. After centrifugation, the serum from SD rats was obtained and stored at −80 °C until the analysis of the Ex-4 concentration. The serum concentration of Ex-4 was measured using Ex-4 Enzyme Immunoassay Kit (Phoenix Pharmaceuticals, Inc., Burlingame, CA), following the manufacturer's protocol.
2.9 In vivo evaluation of (Ex-4)2–Fc
The HFD-fed obese mice were treated with (Ex-4)2–Fc (1.8 mg kg−1) or PBS as the vehicle control once every 6 days for 14 days by s.c. injection. The 3rd group of the age-matched control mice on the standard diet received PBS for 14 days. Body weight and food intake were recorded daily. At the end of the experiment, mice were sacrificed by cervical dislocation. Next, inguinal white adipose tissue (iWAT) and epididymal white adipose tissue (eWAT) were immediately dissected, weighed, fixed in 4% paraformaldehyde or snap-frozen in liquid nitrogen, and then stored at −80 °C. Blood was collected and stored at −80 °C. Similar to the DIO mice, ob/ob and db/db mice were treated with (Ex-4)2–Fc or the vehicle control for 14 days and the metabolic parameters were determined.
2.10 Glucose and insulin tolerance tests
To evaluate glucose tolerance, the overnight-fasted mice were administered a single intraperitoneal (i.p.) injection of glucose (2 g kg−1), followed by a tail vein blood sampling at 0, 30, 60, and 120 min for the DIO mice and at 0, 15, 30, 45, 60, 90, and 120 min for ob/ob and db/db mice. The blood glucose levels were monitored using the AccuChek Active glucometer (Roche Diagnostic, Mannheim, Germany). To determine insulin tolerance, the 6 h fasted DIO mice were i.p. injected with human insulin (0.5 U kg−1, Novo Nordisk, Bagsværd, Denmark), followed by the tail vein blood collection. The blood glucose levels were determined at the same intervals as described above.
2.11 Assays for plasma insulin level
Blood was collected from the tail vein, following 12 h fasting of mice before treatment and after 14 days treatment. The plasma level of insulin was determined using Mouse Insulin ELISA (Millipore, Billerica, MA).
2.12 Histological analysis
eWAT tissues fixed in 4% paraformaldehyde were processed for paraffin embedding, sectioned at 4 μm, and stained with hematoxylin and eosin (H&E). The mean adipocyte size was determined using NIH Image J software.
2.13 Statistical analysis
All data are presented as mean ± SEM. The differences between 2 groups were analyzed by unpaired two-tailed Student's t-test and multiple independent groups were compared by 2-way ANOVA with an appropriate post hoc comparison using GraphPad Prism 6 software (GraphPad Software, San Diego, CA). A value of p < 0.05 was considered as statistically significant.
3. Results
3.1 Design, expression, and purification of Ex-4–Fc and (Ex-4)2–Fc fusion proteins
The fusion proteins, (Ex-4)2–Fc and Ex-4–Fc, consisting of two copies and one copy of the Ex-4 molecules, respectively, were linked via a (GGGGS)3 linker to a mutant human IgG4 Fc segment (Fig. 1A). The purified (Ex-4)2–Fc and Ex-4–Fc proteins were analyzed using SDS-PAGE and Western Blotting (WB). The results showed that under the reducing electrophoretic conditions, the Ex-4–Fc and (Ex-4)2–Fc proteins existed as monomers of ∼31 kDa and 37 kDa, respectively (Fig. 1B and C).
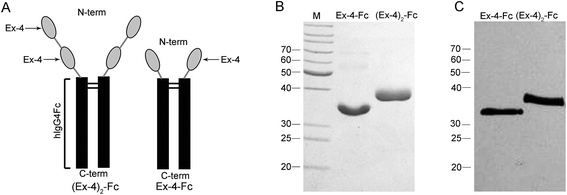 |
| Fig. 1 Design and purification of the Ex-4–Fc and (Ex-4)2–Fc fusion proteins. (A) One or two copies of Ex-4 were fused to the mutant human IgG4 Fc segment. (B) Fusion proteins were analyzed by SDS-PAGE and (C) western blotting with anti-Ex-4 antibody (lane 2, Ex-4–Fc; lane 3, (Ex-4)2–Fc). The fusion proteins appeared as 31 kDa monomer of Ex-4–Fc and 37 kDa monomer of (Ex-4)2–Fc under reducing conditions. | |
3.2 Bioassay of fusion proteins in vitro
The biological activity of (Ex-4)2–Fc and Ex-4–Fc was assessed in the U2OS cells derived from stably expressing GLP-1R, using an in vitro cyclic adenosine monophosphate (cAMP) bioassay. The results showed that (Ex-4)2–Fc and Ex-4–Fc displayed the activity with ED50 of approximately 0.64 mg L−1 and 7.11 mg L−1, respectively (Fig. 2A), indicating that the bioactivity of (Ex-4)2–Fc was over 10-fold stronger than that of Ex-4–Fc. Then, we studied the difference between two forms of the Ex-4 fusion proteins using the structural analysis. The molecular docking between each fusion protein and the extracellular domain of GLP-1 receptor was performed using the ZDOCK module in the Accelrys Discovery Studio 3.5 software. The structures of (Ex-4)2–Fc and Ex-4–Fc were first confirmed by homology modeling method using the solution conformation of Ex-4 and the crystallography conformation of IgG4 as templates. According to the docking results, Ex-4 can form an unabridged alpha-helix when binding to the GLP-1 receptor, but the Ex-4 fragment of Ex-4–Fc can only maintain a shorter alpha-helix to conform to the binding site (Fig. 2B). Moreover, two Ex-4 arms in (Ex-4)2–Fc play different roles in the ligand–receptor complex: one arm was directly bound to the GLP-1 receptor and the other arm played an auxiliary role, such that it increases the stability of the (Ex-4)2–Fc/GLP-1 receptor complexes.
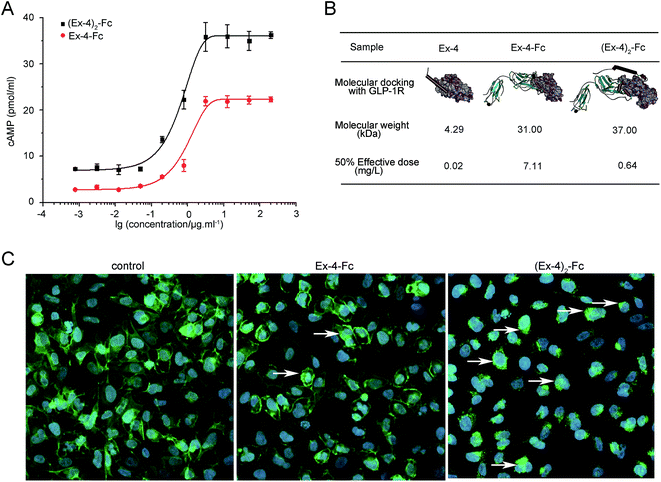 |
| Fig. 2 The biological activity of the Ex-4 fusion proteins on GLP-1R. Functional activity of fusion proteins was investigated using recombinant U2OS cells derived from stably expressing human GLP-1R fused to the N-terminus of enhanced green fluorescent protein (EGFP). (A) Dose response curves of Ex-4–Fc and (Ex-4)2–Fc assessed by in vitro bioassay for detecting cAMP production in the U2OS cell lysates. (B) Molecular docking of Ex-4–Fc or (Ex-4)2–Fc with the GLP-1 receptor by the ZDOCK module. (C) Majority of GLP-1R–EGFP was internalized in endosomes after Ex-4–Fc or (Ex-4)2–Fc stimulated U2OS cells. | |
To assess the effects of the GLP-1R activation, Ex-4–Fc and (Ex-4)2–Fc were investigated using the recombinant U2OS cells that stably express human GLP-1 receptor fused to the N-terminus enhanced green fluorescent protein (EGFP). The majority of GLP-1R–EGFP of the unstimulated cell is localized in the plasma membrane and the majority of GLP-1R–EGFP of the stimulated cell is internalized in endosomes. The results showed that more GLP-1R–EGFP of cells was internalized in endosomes after treated with (Ex-4)2–Fc than treated with Ex-4–Fc (Fig. 2C), suggesting that (Ex-4)2–Fc was more effective in activation of GLP-1R than Ex-4–Fc. These results confirmed that (Ex-4)2–Fc had more potent bioactivity than that of Ex-4–Fc.
3.3 Analysis of (Ex-4)2–Fc fusion protein
The collected fractions were first analyzed for intact molecular weight by LC-MS to detect posttranslational modifications that are present at significant levels with substantial molecular weight differences compared to the theoretical molecular weight calculated from the known amino acids. The deconvoluted mass spectra are shown in Fig. 3A. The molecular weight of the predominant species in the main peak fraction is 74
397.6 Da, which is in agreement with the theoretical molecular weight (74
394.4 Da) of (Ex-4)2–Fc with G0F on both of the Fc chains. Three additional peaks correspond to (Ex-4)2–Fc with other glycoforms. The peak with the molecular weight of 74
559.7 Da corresponds to (Ex-4)2–Fc with G0F on one chain and G1F on the other chain. The peak with the molecular weight of 74
721.7 Da corresponds to (Ex-4)2–Fc with either G1F on both heavy chains or G0F on one chain and G2F on the other chain.
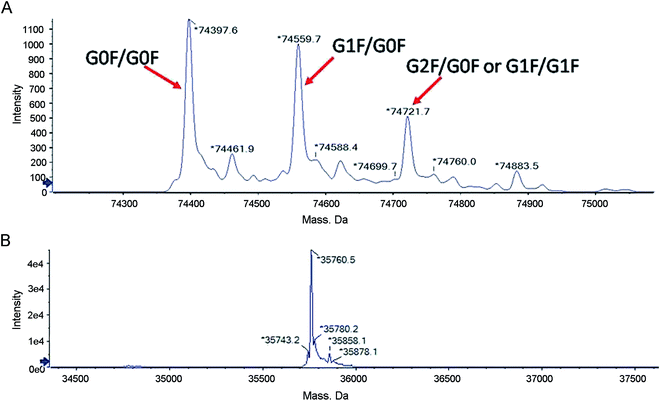 |
| Fig. 3 LC-MS analysis of the (Ex-4)2–Fc molecular weight. (A) LC-MS analysis of posttranslational modifications of (Ex-4)2–Fc. (B) LC-MS analysis of (Ex-4)2–Fc after digested using PNGase F. | |
The proteins were reduced and then digested using PNGase F to generate a single chain and then analyzed by LC-MS to further confirm the observed molecular weight. The deconvoluted mass spectra are shown in Fig. 3B. The molecular weight of the peak is 35
760.5 Da, which is in agreement with the theoretical molecular weight of 35
758.7 Da. The observation of the molecular weight indicates the correct expression of the protein with no unexpected modifications.
3.4 The pharmacokinetics of (Ex-4)2–Fc in SD rats
The pharmacokinetics of a single dose of (Ex-4)2–Fc was studied in SD rats in comparison to Ex-4. After a single dose of 0.3 mg kg−1 Ex-4 by s.c. injection, the plasma concentration of Ex-4 rapidly reached the peak value (Cmax of 382.27 ng mL−1) and declined with a half-life (t1/2) of 0.56 h (Fig. 4A). Moreover, a single s.c. dose of (Ex-4)2–Fc at 0.45 and 1.35 mg kg−1 had a slower absorption phage and slowly reached Cmax of 51.20 and 128.33 ng mL−1, respectively. The half-life of (Ex-4)2–Fc was 122.16 and 122.40 h, respectively, much longer than that of Ex-4 (Fig. 4B). The pharmacokinetic parameters of (Ex-4)2–Fc in SD rats are summarized in (Table 1). These results suggest that (Ex-4)2–Fc could effectively prolong the half-life compared with Ex-4.
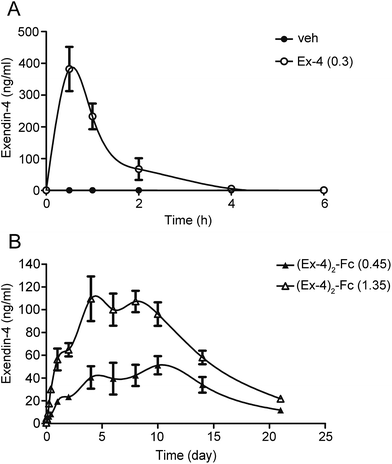 |
| Fig. 4 Pharmacokinetics of (Ex-4)2–Fc in the SD rats. (A) The results of pharmacokinetics studies in the SD rats after a single s.c. injection of 0.3 mg kg−1 of Ex-4. (B) The results of pharmacokinetics studies in the SD rats after a single s.c. injection of 0.45 mg kg−1 of (Ex-4)2–Fc (▲) and 1.35 mg kg−1 of (Ex-4)2–Fc (△). The serum samples were collected and the Ex-4 concentrations were determined by an immunoassay. The data were expressed as mean ± SEM (n = 8, male). | |
Table 1 Pharmacokinetic parameters of Ex-4 and (Ex-4)2–Fc in SD ratsa
Samples |
Tmax (h) |
Cmax (ng mL−1) |
AUC0–21 d (day per ng per mL) |
t1/2 (h) |
MRT (h) |
Tmax, time to reach the maximum plasma concentration; Cmax, the maximum plasma concentration; AUC0–21 d, the area under the curve from zero to 21 days; t1/2, elimination half-life; MRT, mean residence time. |
Ex-4 (0.3) |
0.50 ± 0.14 |
382.27 ± 20.88 |
19.97 ± 0.64 |
0.56 ± 0.05 |
1.10 ± 0.11 |
(Ex-4)2–Fc (0.45) |
240.00 ± 42.33 |
51.20 ± 7.75 |
681.05 ± 62.37 |
122.16 ± 10.13 |
281.04 ± 19.54 |
(Ex-4)2–Fc (1.35) |
96.00 ± 22.98 |
128.33 ± 19.62 |
1536.86 ± 76.09 |
122.40 ± 7.55 |
251.76 ± 16.94 |
3.5 Pharmacodynamics of (Ex-4)2–Fc in DIO mice
Male C57BL/6 mice on a high-fat diet (HFD) became obese (designated DIO mice) and weighed ∼30% more than those fed a standard diet (STD) after 12 weeks.20 The DIO mice were treated with a dose of (Ex-4)2–Fc (0.9 mg kg−1) by s.c. injection once every 3 days for 14 days or a dose of (Ex-4)2–Fc (1.8 mg kg−1) by s.c. injection once every 6 days for 14 days. We found that (Ex-4)2–Fc (3) and (Ex-4)2–Fc (6) groups significantly reduced body weight in the DIO mice, but there was no significant difference between (Ex-4)2–Fc (3) and (Ex-4)2–Fc (6) groups (Fig. S1†).
In addition, the (Ex-4)2–Fc group was administered with (Ex-4)2–Fc once every 6 days via s.c. injection at a dose of 1.8 mg kg−1 for 14 days in the DIO mice and the vehicle control group was administered with PBS once every 6 days via s.c. injection. We found that such (Ex-4)2–Fc treatment significantly (p < 0.001) reduced the HFD-induced weight gain compared to the vehicle control (Fig. 5A) and also significantly reduced food intake (Fig. 5B). The adipocyte size in the (Ex-4)2–Fc treated mice fed with the HFD and the vehicle control mice fed with the STD showed significant reduction than that observed in the vehicle control mice fed with the HFD (Fig. 5C and D). Furthermore, epididymal and inguinal fat pad weight in the (Ex-4)2–Fc treated mice fed with the HFD and the vehicle control mice fed with the STD were significantly decreased compared to those in the vehicle control mice fed with the HFD (Fig. 5E). The effect of the (Ex-4)2–Fc group on biochemical indices is presented in Table S1.† Cholesterol (CHOl) and low-density lipoprotein (LDL) were significantly decreased (p < 0.01) and high-density lipoprotein (HDL) was significantly higher (p < 0.01) compared to those in the vehicle control mice fed with the HFD. There was no difference between the (Ex-4)2–Fc group and the vehicle control mice fed with the STD in the analysis of biochemical criterion (Table S1†).
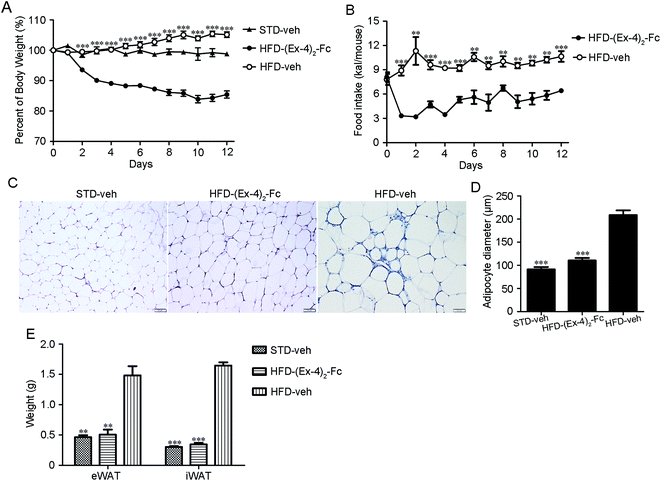 |
| Fig. 5 Metabolic effect of the (Ex-4)2–Fc treatment in the DIO mice. Mice were given (Ex-4)2–Fc (1.8 mg kg−1) or vehicle by s.c. injection once every 6 days for 14 days (n = 8 mice per group). Food intake and body weight were monitored daily. (A) The effects of (Ex-4)2–Fc on the percentage body weight. (B) Daily food intake. (C) Representative H&E staining of eWAT (magnification ×200). (D) Adipocyte size. (E) The weights of iWAT and eWAT. Data represent as mean ± SEM. *P < 0.05; **P < 0.01; ***P < 0.001 vs. HFD-veh. | |
3.6 (Ex-4)2–Fc improves glucose intolerance, increases insulin sensitivity in DIO mice
Since obesity is frequently associated with insulin resistance and glucose homeostasis,21 we next evaluated whether (Ex-4)2–Fc alters the systemic insulin resistance and glucose intolerance in the DIO mice by performing insulin tolerance (ITT) and glucose tolerance (GTT) tests. Interestingly, when the blood glucose levels were measured at 0, 30, 60, and 120 min after a glucose challenge, the (Ex-4)2–Fc administration significantly improved glycemic excursion and AUC compared with the vehicle control in the DIO mice (Fig. 6A), suggesting that (Ex-4)2–Fc improved glucose intolerance. Moreover, the (Ex-4)2–Fc-treated DIO mice, compared to the untreated ones, exhibited significantly reduced blood glucose levels at 60 and 120 min after insulin administration (Fig. 6B), indicating that (Ex-4)2–Fc attenuated insulin resistance. In addition, plasma insulin and glucose concentrations were normalized after the (Ex-4)2–Fc administration at the end of 14 day treatment (Fig. 6C and D). These data suggest that (Ex-4)2–Fc improves glucose metabolism and insulin resistance in the DIO mice.
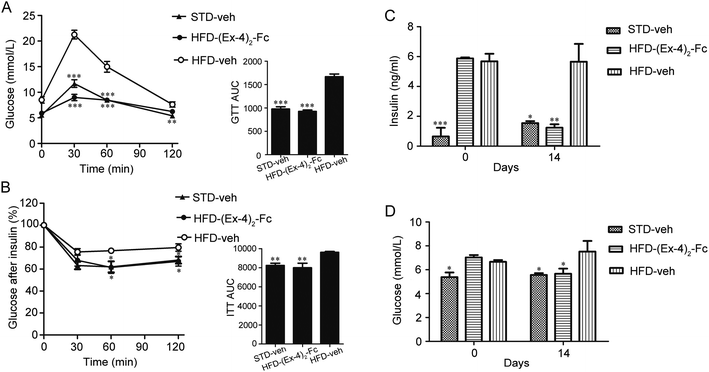 |
| Fig. 6 Administration of (Ex-4)2–Fc improves glucose intolerance and insulin resistance in the DIO mice. (A) Glucose time–response curve (0 to 120 min) and the area under the curve (AUC0–120). Intraperitoneal (i.p.) glucose tolerance test (IPGTT; 2 g glucose per kg body weight, n = 6) was performed in the overnight-fasted mice. (B) Glucose concentration curve as a function of time and the area under the curve (AUC0–120). Insulin tolerance test (ITT; 0.5 U kg−1 body weight, n = 6) was performed in mice fasted for 6 h and was assessed on day 14. (C) Insulin concentration of day 0 and 14. (D) Glucose concentration of day 0 and 14. All data are presented as mean ± SEM. *P < 0.05; **P < 0.01; ***P < 0.001 vs. HFD-veh. | |
3.7 Weight-reducing effect and glucose tolerance of (Ex-4)2–Fc in ob/ob and db/db mice
To assess whether (Ex-4)2–Fc has efficacy in other mouse models of obesity, leptin-deficient ob/ob or leptin receptor-defective db/db mice were treated with (Ex-4)2–Fc (1.8 mg kg−1) by s.c. injection once every 6 days over a 14 day period. We found that the (Ex-4)2–Fc treatment resulted in a sustained and significant decrease in body weight of ob/ob mice by 10% (p < 0.001) (Fig. 7A) and db/db mice by 8% (p < 0.01) (Fig. 7C), compared to the vehicle treatment. It is worth mentioning that the treatment with (Ex-4)2–Fc caused a marked decrease in food intake in the ob/ob and db/db mice on the first day of treatment, but food intake was then recovered rapidly (Fig. 7B and D). After 14 days of treatment, we evaluated the glucose-lowering effects of (Ex-4)2–Fc in ob/ob and db/db mice during an intraperitoneal glucose tolerance test (IPGTT) (2 g of glucose per kg of body weight). The blood glucose levels were monitored at 0, 15, 30, 45, 60, 90, and 120 min after the administration of the glucose load. The administration of (Ex-4)2–Fc significantly improved glucose tolerance by 20.2% and 20.4% (assessed as total AUC during IPGTT) in ob/ob and db/db mice, respectively (Fig. 7E and F). Moreover, after 14 days of treatment, ob/ob and db/db mice were fasted for 6 h and then intraperitoneally injected with insulin (0.5 units perkg of body weight). Indeed, in ob/ob and db/db mice, the glucose-lowering effect after i.p. insulin injection in the (Ex-4)2–Fc group was significantly greater than that in the vehicle control group (Fig. S2†). Collectively, the decrease in body weight, food intake, and the improvement of glucose tolerance and insulin tolerance by (Ex-4)2–Fc was also evident in ob/ob and db/db mice.
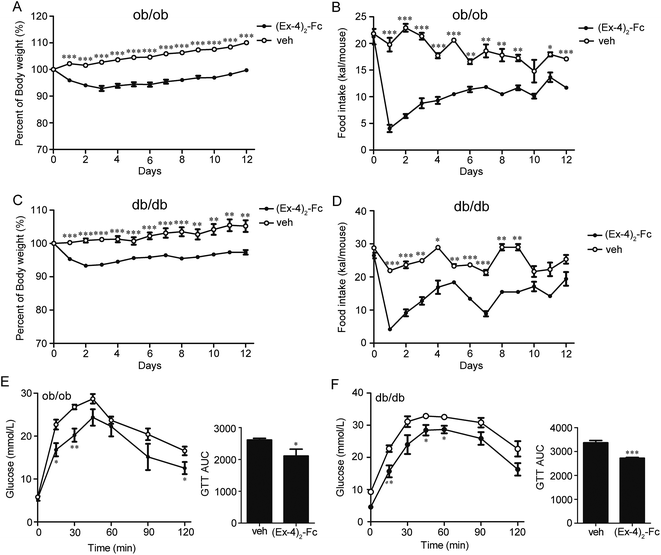 |
| Fig. 7 Metabolic effects of (Ex-4)2–Fc in ob/ob and db/db mice. (A) Percentage body weight curves of ob/ob mice treated with (Ex-4)2–Fc or vehicle (n = 6). (B) Food intake curves of ob/ob mice. (C) Percentage body weight curves of db/db mice treated with (Ex-4)2–Fc or vehicle (n = 6). (D) Food intake curve of db/db mice. (E) Glucose time–response curve (0 to 120 min) and the area under the curve (AUC0–120) of ob/ob mice. (F) Glucose time–response curve (0 to 120 min) and the area under the curve (AUC0–120) of db/db mice. Intraperitoneal (i.p.) glucose tolerance test (IPGTT; 2 g glucose per kg body weight, n = 6) was performed in overnight fasted mice. All data are presented as mean ± SEM. *P < 0.05; **P < 0.01; ***P < 0.001 vs. veh. | |
4. Discussion
GLP-1 receptor agonists are new drugs for the treatment of diabetes in recent years, which have the potential to reduce glucose levels while reducing the side effects of hypoglycemia and weight gain. Endogenous GLP-1 is rapidly degraded by DPP-4, thus a number of GLP-1 analogs that are resistant to degradation by DPP-4 have been developed. Existing members of short-acting and long-acting GLP-1 analogs that have been approved by FDA for the treatment of T2DM include exenatide (twice-daily),22 liraglutide (once-daily), and albiglutide (once-weekly). Interestingly, a C16 acyl chain attached to lysine 26 and lysine 34 is substituted with arginine in liraglutide,22,23 while albiglutide is a dimer of two repeats of human GLP-1 (7–36) fused to human albumin and a single amino acid substitution (alanine 8 → glycine).24 In this study, we successfully engineered two forms of fusion proteins, Ex-4–Fc and (Ex-4)2–Fc, which were constructed from exendin-4 and a mutant human IgG4–Fc. While Ex-4–Fc contains only a single Ex-4 fragment fused to IgG4 Fc, (Ex-4)2–Fc consisted of two Ex-4 fragments fused to IgG4 Fc. Using the molecular docking of the fusion proteins with GLP-1R and an in vitro bioactivity assay, we demonstrated that (Ex-4)2–Fc was a more potent GLP-1R agonist than Ex-4–Fc. Furthermore, the (Ex-4)2–Fc fusion protein can self-assemble to covalently linked stable homodimer by MALDI-TOF mass spectrometry analyzation, while Ex-4–Fc tends to form multimers (data not shown).
Currently, an increasing number of Fc fusion proteins have been developed for therapeutic applications, primarily with the aim to improve the bioactivity through dimerization and to extend half-life, mediated by the neonatal Fc receptor (FcRn), for example, BR3–Fc fusion protein (briobacept), rFIXFc, and rFVIII–Fc.25 Immunoglobulins of the IgG1, IgG2 and IgG4 subclass can exhibit a long half-life of 3–4 weeks by a FcRn-mediated recycling process although their half-life is also affected by antigen-dependent elimination, such as through receptor-mediated endocytosis and intracellular degradation.26,27 A previous study has shown that Ex-4/Fc, a fusion protein consisting of exendin-4 and mouse IgG1 heavy chain constant region (IgG1 Fc), improves energy and lipid metabolism.17 IgG1 Fc has generally been used as a conjugate to prolong the half-life of a bioactive molecule, but has its shortcomings, such as complement-dependent and antibody-dependent cell-mediated cytotoxicity.28 When compared together, there is a 10-fold difference in the ability of IgG1 and IgG4 to bind to Fcγ RI (CD64, high-affinity Fc receptor).29
Therefore, we replaced IgG1 with a variant IgG4, containing 3 amino acid substitutions relative to native IgG4 Fc (S228P, F234A, and L235A) and deleted the C-terminal lysine of the IgG4 Fc to lower the immunogenicity and stabilize the IgG4 peptide.30. (Ex-4)2–Fc was prepared using mammalian cell lines with CHO cell lines. In addition to the extended half-life, the Fc domain could also provide other benefits for the fusion protein, such as enhancing expression and secretion using Protein-A chromatography to provide a facile purification strategy as well as improving solubility and stability. The plasma pharmacokinetic profiles of (Ex-4)2–Fc have shown a dramatically longer half-life and larger AUC0–21 d compared with those of the Ex-4 administered as a single injection in the SD rats. The half-life of (Ex-4)2–Fc was prolonged by five days, almost 250-fold longer than that of Ex-4 in rats, suggesting that the injection frequency could be reduced from daily to weekly.
To eliminate the potential ability of body weight reduction and glucose improvement in rodents, the treatment of the DIO mice with (Ex-4)2–Fc for 14 days resulted in a significant attenuation of weight gain as compared with the treatment with the vehicle control. It also significantly reduced food intake, decreased fasting blood, insulin and glucose levels, and improved glucose tolerance and insulin sensitivity. We also used genetically modified obese ob/ob mice and db/db mice to confirm the effects of (Ex-4)2–Fc on reducing body weight, food intake, and glucose level. By cross-comparison of the potency of (Ex-4)2–Fc in three mouse models of diabetes, we noticed that compared with the vehicle control treatment, the (Ex-4)2–Fc treatment resulted in a greater than ∼20% reduction in body weight in the DIO mice and ∼10% reduction in body weight in ob/ob or db/db mice. Furthermore, the administration of (Ex-4)2–Fc modestly improved glucose intolerance and reduced food intake in ob/ob and db/db mice, which is in agreement with the findings of the previous studies.31 These data indicated that the (Ex-4)2–Fc fusion protein occurred without compromising its biological activity and the in vitro potency and in vivo efficacy were sustained.
In summary, the low-administration frequency of (Ex-4)2–Fc was shown to have the long-lasting effects on body weight reduction and glucose tolerance improvement in a variety of rodents. The pharmacokinetics and pharmacodynamics profiles of (Ex-4)2–Fc strongly indicate that (Ex-4)2–Fc could be potentially used as a clinical treatment for obesity and diabetes.
Author contributions
We gratefully thank L. L. designed the study, researched the data, and contributed to the discussion, review, editing, and final approval of the manuscript. X. S. researched the data. Y. W., Y. L., J. Y., L. X., and X. G. contributed to the discussion and review. Y. M., Y. T., F. Y., B. Z., Y. F., X. Z., R. H., Y. K., H. D., and Q. Z. researched the data and operated the animals. G. H. and L. J. researched the data. L. Y. is the guarantor of this work and, as such, had full access to all of the data in this study and takes responsibility for the integrity of the data and the accuracy of the data analysis.
Conflicts of interest
The authors declare no conflict of interest.
Acknowledgements
This work was supported by National Major Scientific and Technological Special Project for “Significant New Drugs Development” during the Twelfth Five-year Plan Period (2013ZX09301304-001). We thank Shile Huang, Health Sciences Center, Louisiana State University, for help in the review of this manuscript.
References
- M. Tauber, Rev. Du. Prat. La, 2015, 65, 1263 Search PubMed.
- C. A. Maggio and F. X. Pi-Sunyer, Endocrinol. Metab. Clin. North Am., 2003, 32, 805–822 Search PubMed.
- M. E. Molitch, Am. J. Manag. Care, 2013, 19, S136–S142 Search PubMed.
- D. J. Drucker, Cell Metabol., 2006, 3, 153–165 CrossRef CAS PubMed.
- M. Gutniak, C. Orskov, J. J. Holst, B. Ahren and S. Efendic, N. Engl. J. Med., 1992, 326, 1316–1322 CrossRef CAS PubMed.
- E. N. Murage, G. Gao, A. Bisello and J. M. Ahn, J. Med. Chem., 2010, 53, 6412–6420 CrossRef CAS PubMed.
- L. L. Baggio and D. J. Drucker, Gastroenterology, 2007, 132, 2131–2157 CrossRef CAS PubMed.
- B. Thorens, A. Porret, L. Buhler, S. P. Deng, P. Morel and C. Widmann, Diabetes, 1993, 42, 1678–1682 CrossRef CAS PubMed.
- B. Gallwitz, Drugs, 2011, 71, 1675–1688 CrossRef CAS PubMed.
- M. Davies, S. Heller, S. Sreenan, H. Sapin, O. Adetunji, A. Tahbaz and J. Vora, Diabetes Care, 2013, 36, 1368–1376 CrossRef CAS PubMed.
- N. A. Painter, C. M. Morello, R. F. Singh and S. E. McBane, J. Am. Board Fam. Med., 2013, 26, 203–210 CrossRef PubMed.
- P. L. McCormack, Drugs, 2014, 74, 325–351 CrossRef CAS PubMed.
- V. A. Fonseca, R. Alvarado-Ruiz, D. Raccah, G. Boka, P. Miossec, J. E. Gerich and E. F. C. G.-M. S. Investigators, Diabetes care, 2012, 35, 1225–1231 CrossRef CAS PubMed.
- J. Wroge and N. T. Williams, Ann. Pharmacother., 2016, 50, 1041–1050 CrossRef CAS PubMed.
- S. J. Kim, H. H. Kwak, S. Y. Cho, Y. B. Sohn, S. W. Park, R. Huh, J. Kim, A. R. Ko and D. K. Jin, Mol. Pharm., 2015, 12, 3759–3765 CrossRef CAS PubMed.
- K. J. Pasi, K. Fischer, M. Ragni, B. Nolan, D. J. Perry, R. Kulkarni, M. Ozelo, J. Mahlangu, A. D. Shapiro, R. I. Baker, C. M. Bennett, C. Barnes, J. Oldenburg, T. Matsushita, H. Yuan, A. Ramirez-Santiago, G. F. Pierce, G. Allen and B. Mei, Thromb. Haemostasis, 2017, 117, 508–518 CrossRef PubMed.
- R. Liu, D. Ma, Y. Li, R. Hu, Y. Peng and Q. Wang, Acta Biochim. Biophys. Sin., 2014, 46, 675–681 CrossRef CAS PubMed.
- H. S. Chung, J. Y. Oh, S. B. Yoo, S. M. Lee and H. S. Cho, Regul. Pept., 2011, 170, 1–3 CrossRef CAS PubMed.
- Y. Gan, N. Dang, Z. Qu, R. Shi, L. Ding, L. Wang and S. Pang, Exp. Clin. Endocrinol. Diabetes, 2015, 123, 371–375 CrossRef CAS PubMed.
- P. D. Lambert, K. D. Anderson, M. W. Sleeman, V. Wong, J. Tan, A. Hijarunguru, T. L. Corcoran, J. D. Murray, K. E. Thabet, G. D. Yancopoulos and S. J. Wiegand, Proc. Natl. Acad. Sci. U. S. A., 2001, 98, 4652–4657 CrossRef CAS PubMed.
- E. D. Rosen and B. M. Spiegelman, Nature, 2006, 444, 847–853 CrossRef CAS PubMed.
- U. Ritzel, U. Leonhardt, M. Ottleben, A. Ruhmann, K. Eckart, J. Spiess and G. Ramadori, J. Endocrinol., 1998, 159, 93–102 CrossRef CAS PubMed.
- T. Vilsboll, Expet Opin. Invest. Drugs, 2007, 16, 231–237 CrossRef CAS PubMed.
- J. M. Trujillo and W. Nuffer, Ann. Pharmacother., 2014, 48, 1494–1501 CrossRef CAS PubMed.
- C. Huang, Curr. Opin. Biotechnol., 2009, 20, 692–699 CrossRef CAS PubMed.
- M. A. Tabrizi, C. M. Tseng and L. K. Roskos, Drug discovery today, 2006, 11, 81–88 CrossRef CAS PubMed.
- N. L. Dirks and B. Meibohm, Clin. Pharmacokinet., 2010, 49, 633–659 CrossRef CAS PubMed.
- O. H. Brekke and J. E. Thommesen, Meth. Mol. Biol., 2003, 207, 383–391 CAS.
- S. M. Canfield and S. L. Morrison, J. Exp. Med., 1991, 173, 1483–1491 CrossRef CAS PubMed.
- W. Glaesner, A. M. Vick, R. Millican, B. Ellis, S. H. Tschang, Y. Tian, K. Bokvist, M. Brenner, A. Koester, N. Porksen, G. Etgen and T. Bumol, Diabetes Metabol. Res. Rev., 2010, 26, 287–296 CrossRef CAS PubMed.
- A. A. Young, B. R. Gedulin, S. Bhavsar, N. Bodkin, C. Jodka, B. Hansen and M. Denaro, Diabetes, 1999, 48, 1026–1034 CrossRef CAS PubMed.
Footnotes |
† Electronic supplementary information (ESI) available. See DOI: 10.1039/c7ra10822b |
‡ Two authors contributed equally to this work. |
|
This journal is © The Royal Society of Chemistry 2017 |
Click here to see how this site uses Cookies. View our privacy policy here.