DOI:
10.1039/C7RA11283A
(Paper)
RSC Adv., 2017,
7, 54485-54490
Electron transportation path build for superior photoelectrochemical performance of Ag3PO4/TiO2†
Received
13th October 2017
, Accepted 22nd November 2017
First published on 28th November 2017
Abstract
TiO2 is an attractive photoanode material with its large band gap, whilst its performance largely suffers from low efficiency on both charge separation and solar conversion. Herein, a self-organized TiO2 nanotube arrays (TNT) is prepared by anodized Ti foil in ethylene glycol electrolyte to ameliorate charge transmission ability. Ag3PO4 is further synthesized on TNT substrate by dipping method. HRTEM images results indicate Ag3PO4 nanoparticles are successfully deposited on the surfaces of TNT. Photoelectrochemical tests show the Ag3PO4/TiO2 heterojunction has a higher photocurrent density of 2.34 mA cm−2 at 0 V than that of pure TNT (0.38 mA cm−2). This is attributed to an Ag “pump” reduced on the interface of Ag3PO4/TiO2, therefore electron transportation path is built between Ag3PO4 and TiO2 leading to photogenerated electrons and holes effective separation. This high photocurrent density array films facilitates it a desirable photoelectrochemical material for water splitting.
1 Introduction
Photoelectrochemica (PEC) water splitting is recognized as one of the most promising strategies in solar energy conversion. Titanium dioxide (TiO2) has been specifically selected out among various PEC materials since Honda–Fujishima first found the ability of TiO2 for photo-splitting water under ultraviolet light in 1972.1 In 1976, Carey found that PCBs in TiO2 suspension solution successfully dechlorinated under UV irradiation.2 In 1991, Graztel successfully developed a dye-sensitized solar cell by using TiO2 nanoparticles.3 But TiO2 suffers from its poor charge separation and high charge transmission resistance.4,5 One dimensional (1-D) nanostructure allows a short diffusion length for holes in radial direction, whereas the long axial direction of the structure becomes the preferred electron channel that provides enough length of light attenuation as well.6,7 Besides these above, 1-D nanostructures also provide excellent photovoltaic, photocatalytic, and PEC properties relative to random-shaped particles.8–12 Therefore, the 1-D self-organized TiO2 nanotube array (TNT) is expected to design. In 2001, Grimes and co-workers successfully prepared TNT by anodization in hydrofluoric acid electrolyte.13 Further, Macak synthesized TNT by anodizing in glycerol electrolyte containing 0.5% NH4F, the morphology more smooth than that obtained in aqueous electrolyte, and the length of nanotube reached above 10 μm.14 Also, TNT exhibits more attractive PEC performance and larger specific surface area than TiO2 nanoparticles or nanosheets.15,16 However, band gap of TiO2 at 3.0–3.2 eV can only absorb ultraviolet light corresponding for 5% of sunlight, which means the solar utilization efficiency is less than satisfactory.
Ag3PO4 a n-type semiconductor can absorb visible light wavelengths less than 520 nm (indirect band gap of 2.4 eV), and the quantum yield reaches more than 90%, which aroused attention of many researchers;17–19 but its conduction band (CB) is at 0.45 V vs. NHE, so Ag+ of Ag3PO4 will be easily reduced to elemental Ag under visible light irradiation.10,20–26 Wang et al. studied Ag3PO4/AgBr/Ag degradation of organic matter, due to better stability of AgBr and plasma effect of Ag nanoparticles, which improved Ag3PO4 stability and photocatalytic capacity.27 Teng found that Ag/Ag3PO4/TiO2 can photodegrade chlorophenol efficiently, and Ag nanoparticles can effectively prevent the occurrence of Ag3PO4 light corrosion, and photocurrent density at 0.28 mA cm−2.28 Xu et al. reported Ag3PO4/TiO2/Fe3O4 photodegraded benzene sulfonate and had a great bactericidal effect.29 Photodegradation properties on Ag3PO4 or its composites can be found in many research, but seldom on PEC properties, which is closely relative to photo-water-splitting. Moreover, all of works is not enough to achieve high performance because electrons randomly flow after the electron–hole separation resulting in fast electron–hole recombination.
In this study, Ag3PO4 nanoparticles were chemically deposited on 1-D TNT. A heterojunction of Ag3PO4/TiO2 was formed and linear sweep voltammetry test showed photocurrent was about 6 times bigger than pure TNT and the band flat potentials of Ag3PO4/TiO2 positively shifted, which meant solar absorption range of TiO2 was significantly broadened. Ag was also synthesized on the interface between Ag3PO4 and TiO2 due to light illumination, which formed an efficient system for separation of photo-generated charges and improvement of PEC properties.
2 Experimental
2.1 Sample Ag3PO4/TiO2 preparation
Ti foil of 1 cm2 was provided with anodization in ethylene glycol electrolyte containing 4 wt% H2O and 0.25 wt% NH4F for 1 h under constant potential (50 V) at room temperature.14,30 The samples were washed with distilled water and dried at 60 °C. The as-prepared TiO2 was thermally treated at 450 °C for 2 h under atmospheric conditions.
Ag3PO4 nanoparticles were deposited into the crystallized TiO2 nanotubes by sequential chemical bath deposition method. Typically, the sample was successively immersed in four different beakers for 3 minutes in each beaker. One beaker contained 5 mM AgNO3 aqueous solution, another contained 5 mM NaH2PO4, and the other two contained distilled water to rinse the samples from the excess of each precursor solution. Such an immersion cycle was repeated several times, typically between 2 and 8 cycles.
2.2 Characterization
The surface morphology was observed through a field-emission scanning electron microscope (Hitachi S-4800, Japan). Transmission electron microscopy (TEM) images were taken with JEOL JEM-2100 transmission electron microscope at 200 kV. The absorbance was measured with UV-vis spectrophotometer (Hitachi U-3900, Japan). The elemental chemical status was measured by X-ray photoelectron spectroscopy (Thermo ESCALAB 250Xi), equipped with Al Kα radiation. All energies were calibrated to spurious carbon at 284.8 eV.
The PEC properties of samples were investigated by a three-electrode configuration electrochemical workstation (CHI 760E), employing the samples, Ag/AgCl and Pt mesh as working, reference and counter electrode, respectively (Fig. S4†). The supporting electrolyte used was 1 M KOH (pH = 14) aqueous solution. Mott–Schottky plots were measured at 1000 Hz. Electrochemical impedance spectroscopic (EIS) measurements were performed between 105 Hz and 10−2 Hz dark. The working electrode was illuminated with a 300 W xenon lamp. The photocurrent was recorded concurrently with the light switching on and off without applied voltage.
3 Results and discussion
3.1 Electron transportation analysis
After deposited Ag3PO4, a heterojunction of Ag3PO4/TiO2 first formed and promoted separation of the photogenerated carriers.25,31,32 Additionally, Ag+ of Ag3PO4 was proved photoreduced and decomposed to weakly active Ag under photo-illumination,31–35 which could obviously enhance visible light absorbance above 700 nm (Fig. 1c) and accelerate photogenerated carrier separation due to its surface plasma resonance (SPR).28,36 As reported by many researchers, SPR in Ag can be directly excited under visible light illumination to generate and inject hot carriers into the CB of semiconductors.37,38 This role is just like a “pump” (Fig. 1a and b), electrons in Ag3PO4 are absorbed by Ag and then “pumped” into higher energy level, the CB of TiO2, due to lower conductivity of Ag3PO4 and SPR of Ag which assisted electrons to get over the barrier of Ag3PO4/TiO2 junction. Then electrons transported from the CB of TiO2 to Ti base along 1-D electron channel of TNT. Equally, holes were injected from the valence band (VB) of TiO2 into the VB of Ag3PO4, and then participated in oxidation reaction. The transfer route of electron can be ECB(Ag3PO4) → Ef(Ag) → ECB(TiO2). If without the SPR of Ag, charges transportation route are shown in Fig. S4,† the light induced electrons transported from higher conductor band (−0.612 V vs. NHE) of TiO2 to lower conductor band (+0.45 V vs. NHE) of Ag3PO4, and the holes were accumulated in the valence band of TiO2, which is inconsistent with experimental results of electron transportation from TiO2 to Ti foil.
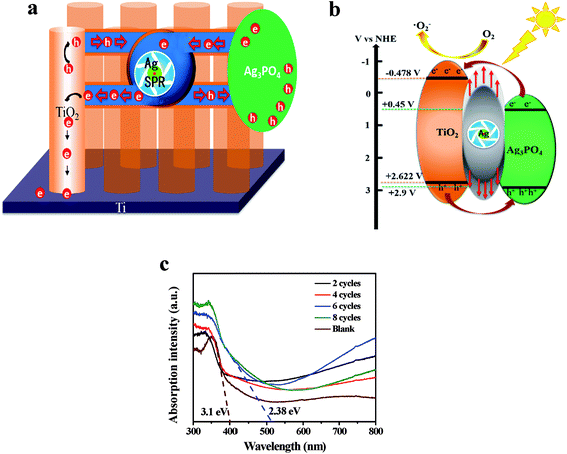 |
| Fig. 1 Schematic diagram of bidirectional photo-induced electron transportation (a), photogenerated electron–hole pairs separation process (b) and UV-vis spectra (c). | |
3.2 XPS analysis
Ag3PO4/TiO2 sample was examined by X-ray photoelectron spectroscopy (XPS), and the results are shown in Fig. 2. The binding energies of the XPS spectra were calibrated by C 1s (284.8 eV). The carbon peak is due to the adventitious hydrocarbon from the XPS instrument itself (Fig. S2†). In Fig. 2a, the characteristic peak at 458.5 eV and 464.2 eV assigned to Ti4+ in TiO2 are depicted.39 Two binds at 367.7 and 373.7 eV, are ascribed to Ag 3d5/2 and Ag 3d3/2 bonding energies in Fig. 2b. These bands could be further deconvoluted into two peaks, respectively, at 366.4, 367.3 eV and 372.8, 373.7 eV, where the bands at 366.4 and 372.8 eV are ascribed to the Ag+ of Ag3PO4, and those at 367.3 and 373.7 eV are attributed to the metallic Ag0. As some papers have reported,40,41 these results verify the existence of metallic Ag0 on Ag3PO4/TiO2 photocatalysts after the reaction.
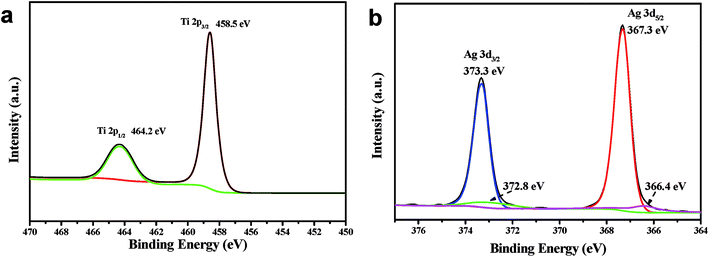 |
| Fig. 2 High resolution XPS spectra of (a) Ti 2p and (b) Ag 3d of Ag3PO4/TiO2. | |
3.3 Morphology characterization
Diameter of the self-organized pure TNT prepared by anodizing method is about 60 to 90 nm (Fig. 3a and b). Fig. 3b showed SEM images of the sample for 4 cycles deposited Ag3PO4 on TNT, and the nanoparticles of Ag3PO4 covered the surface with a diameter of about 10 nm. Also, some particles with a diameter of 40 to 50 nm were formed because of severe agglomeration for 8 cycles, shown in Fig. S3.† Illustration of EDS indicated that the atomic proportion of Ag and P is 2.44 to 1, close to stoichiometric ratio of 3 to 1 (Fig. 3c).
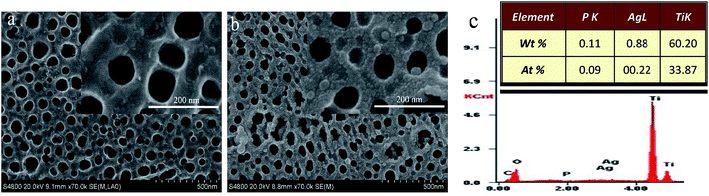 |
| Fig. 3 SEM images of pure TiO2 (a), Ag3PO4/TiO2 4 cycles (b), insert is partial enlarged view of (a) and (b), EDS of 4 cycles (c). | |
TEM images of the sample for 4 cycles deposited Ag3PO4 on TNT were shown in Fig. 4a and b. Ag3PO4 nanoparticles located at both surface TNT and inside of nanotubes at a diameter of 10–20 nm. To further confirm the existence of Ag3PO4, HRTEM (Fig. 4c) showed the lattice fringe of 0.35 nm was consistent with (101) facet of anatase TiO2, while the lattice fringe of 0.42 nm was assigned to the (110) facet of Ag3PO4.
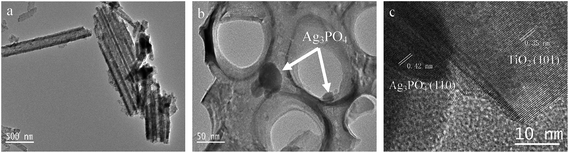 |
| Fig. 4 TEM (a, b) and HRTEM (c) images of Ag3PO4/TiO2, 4 cycles. | |
3.4 Photoelectrochemical properties
Under scanning potential between −0.9 V to 0.3 V vs. Ag/AgCl, the current density of TNT deposited Ag3PO4 were bigger than that of the pure TNT (Fig. 5a), only 0.387 mA cm−2 at 0 V vs. Ag/AgCl, whereas the current density of 4 cycles deposition was almost 5 times higher, reached to 2.340 mA cm−2. It reported that maximum photocurrent of Ag3PO4/TiO2 nanowire array heterostructure photoelectrodes was about 0.7 mA cm−2 at an applied bias 0.23 V vs. Ag/AgCl.42 The improvement of photocurrent could be ascribed to Ag “pump”, preferred electron transportation direction along TNT and large absorption spectrum of Ag3PO4.29,43 UV-vis spectra (Fig. 1c) proved the band gap of pure TNT and Ag3PO4 at 3.1 eV and 2.38 eV, respectively. And the recombination of photogenerated charge carriers was significantly reduced in the heterojunction (Fig. S1b†). Under intermittent light irradiation, time-dependent photocurrent generation is presented in Fig. 5b. The photocurrents respond exactly to the presence of solar light was interrupted every 50 s (light on/off) under 0 V vs. Ag/AgCl, and the steady state behavior of each photoanode follows the same trend as that of the I–V curves (Fig. 5a). Furthermore, the loading amounts of Ag3PO4 on TNT increase with the increasing of cycle time (Fig. 3c and S3c†). Whatever I–V curves or I–t curves showed photocurrent increased initially and then decreased with increase loading amount of Ag3PO4. Therefore appropriate loading amounts of Ag3PO4 is fatal to enhance PEC performance of TNT. In addition, there are no appreciable dark currents, demonstrating the photochemical stability of all of photoanodes in the alkaline solution. The steady-state photocurrents do not show any significant degradation with time.44
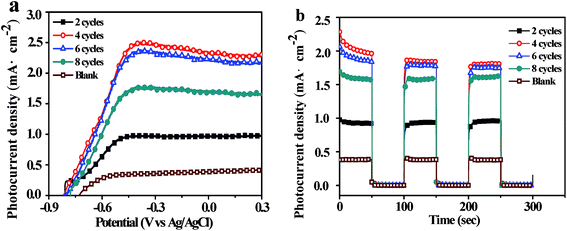 |
| Fig. 5 Ag3PO4/TiO2 composites linear sweeps voltammetry (a), i–t curve (b), UV-vis spectra. | |
Fig. 6a represented Nyquist diagrams from electrochemical impedance spectroscopy (EIS) for samples tested in dark. The equivalent circuit for this cell system was depicted in inset of Fig. 6a. Rb was the bulk resistance of the electrolyte. Csc and Rsc were the capacitance and the resistance of the solid-state interfacial layer which was formed at the highly charged state due to the passivation reaction between the electrolyte and the surface of the electrode. Cdl and Rct were the double layer capacitance and the charge transfer resistance. W was the Warburg resistance result from the diffusion resistance of redox couple.
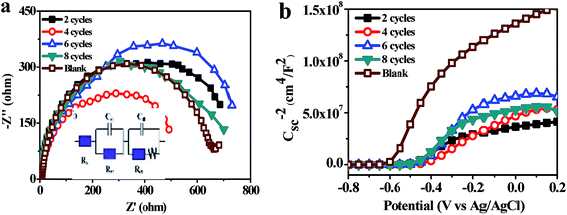 |
| Fig. 6 Ag3PO4/TiO2 Nyquist diagrams (a), insert is equivalent circuit fitting the EIS and Mott–Schottky plots (b). | |
The Mott–Schottky plots of Ag3PO4/TiO2 are used to analyze the flat band potential and carrier concentrations of semiconductor (Fig. 6b). Under the applied voltage, the Fermi level can be changed and bended the energy band, and flat band potential Vfb refers the applied potential which makes the inner electric field intensity of semiconductor approach zero. The carrier concentrations of semiconductor ND and the slope of linear part of Mott–Schottky plots have the relation as follow:
|
 | (1) |
where,
e is the elementary charge (1.602 × 10
−19 C),
ε is the dielectric constant of sample and the dielectric constant of anatase is 48,
ε0 is the vacuum dielectric constant (8.854 × 10
−14 F cm
−1),
m is the slope of the linear part of Mott–Schottky plots. The flat band potential
Vfb,
CSC and carrier concentrations
ND have a relationship, below:
|
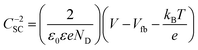 | (2) |
where,
CSC is the space charge capacitance,
ε0,
ε and
e are constant,
ND is carrier concentrations,
V and
Vfb is respectively the applied potential and the flat band potential (
vs. the potential of the reference electrode), (
kBT)/
e is 25.8 mV at room temperature.
The parameters obtained from EIS and Mott–Schottky plots are shown in Table 1. The charge transfer resistances Rct of 4 cycles deposition Ag3PO4 minimal only 404.7 ohm cm−2, since the carrier concentration ND of it was 2.6 times higher than that of the pure TiO2 about 4.85 × 1022 cm−3. Moreover, the carrier concentration of Ag3PO4/TiO2 samples was obviously higher than that of the pure TiO2. And valence bands of two semiconductors were very close,29,33,43,45,46 which inferred the heterojunction formed by depositing Ag3PO4 nanoparticles on TNT (Fig. 1b). Potentials of both conduction band (−0.612 V vs. NHE) and valence band (+2.588 V vs. NHE) of TiO2 are more negative than those of Ag3PO4 (conduction band potential: +0.45 V vs. NHE, valence band potential: +2.9 V vs. NHE).33 It benefits for transfer of photogenerated carriers and separation of photogenerated electron–hole pairs, also increases the carrier concentrations. The flat band potential (Vfb) is close to the conduction band which makes the curving band of space charge layer straight. The Vfb of TNT was −0.612 V, after deposition Ag3PO4 of 4 cycles Vfb lowed to −0.410 V. The reason for lower Vfb was the photogenerated electrons were “pumped” into CB of TiO2 by SPR of Ag. It meant the range of absorption spectrum broaden and photocatalytic properties enhancement. As shown in Fig. 1c, the absorbance of Ag3PO4/TiO2 was significantly increased than pure TiO2, implying Ag3PO4 is a very promising material for building fast electron transportation path to improve PEC performance of TiO2.
Table 1 Physics parameters of Ag3PO4/TiO2
Sample |
Rct (ohm cm−2) |
ND (cm−3) |
Vfb (V) |
2 cycles |
667.5 |
4.40 × 1022 |
−0.478 |
4 cycles |
404.7 |
4.85 × 1022 |
−0.410 |
6 cycles |
698.8 |
2.42 × 1022 |
−0.433 |
8 cycles |
621.4 |
3.16 × 1022 |
−0.468 |
Blank |
594.8 |
1.35 × 1022 |
−0.612 |
4 Conclusion
A preferred electron transportation path was designed to improve the photoelectrocatalytic activity through deposition Ag3PO4 nanoparticles to the self-organized TiO2 nanotube arrays. Ag3PO4/TiO2 effectively reduced the recombination of photogenerated electron–hole pairs due to unique 1-D nanostructure, as well as SPR of photoreduced Ag from Ag3PO4. The charge carrier concentration of Ag3PO4/TiO2 increased 2.6 times than that of pure TNT. The photocurrents of Ag3PO4/TiO2 were significantly improved by 5 times. Therefore, fabrication of Ag3PO4/TiO2 nanotube arrays is a highly efficient method to build a noteworthy photoelectrode for PEC water splitting.
Conflicts of interest
There are no conflicts to declare.
Acknowledgements
The financial support for this study by National Natural Science Foundation of China (No. 21476262). The Technology Development Plan of Qingdao (No. 14-2-4-108-jch). Research Funds for the Central Universities (No. 15CX05032A) are gratefully acknowledged.
References
- A. Fujishima and K. Honda, Nature, 1972, 238, 37–38 CrossRef CAS PubMed.
- J. H. Carey, J. Lawrence and H. M. Tosine, Bull. Environ. Contam. Toxicol., 1976, 16, 697–701 CrossRef CAS PubMed.
- B. O'Regan and M. Gratzel, Nature, 1991, 353, 737–740 CrossRef.
- K. Park, Q. Zhang, D. Myers and G. Cao, ACS Appl. Mater. Interfaces, 2013, 5, 1044–1052 CAS.
- J. Low, B. Cheng and J. Yu, Appl. Surf. Sci., 2017, 392, 658–686 CrossRef CAS.
- W.-T. Sun, Y. Yu, H.-Y. Pan, X.-F. Gao, Q. Chen and L.-M. Peng, J. Am. Chem. Soc., 2008, 130, 1124–1125 CrossRef CAS PubMed.
- X. Feng, K. Shankar, O. K. Varghese, M. Paulose, T. J. Latempa and C. A. Grimes, Nano Lett., 2008, 8, 3781–3786 CrossRef CAS PubMed.
- H. Xiong, M. D. Slater, M. Balasubramanian, C. S. Johnson and T. Rajh, J. Phys. Chem. Lett., 2011, 2, 2560–2565 CrossRef CAS.
- B. Liu and E. S. Aydil, J. Am. Chem. Soc., 2009, 131, 3985–3990 CrossRef CAS PubMed.
- Q. Liang, Y. Shi, W. Ma, Z. Li and X. Yang, Phys. Chem. Chem. Phys., 2012, 14, 15657–15665 RSC.
- H. Cheng-Xing, Y. Lian-Qing, Z. Ya-Ping, D. Kai-Tuo and H. Lan-Zhong, J. Inorg. Mater., 2016, 31, 1237–1241 CrossRef.
- L. Yu, Y. Zhang, Q. Zhi, Q. Wang, F. Gittleson, J. Li and A. D. Taylor, Sens. Actuators, B, 2015, 211, 111–115 CrossRef CAS.
- D. Gong, C. A. Grimes and O. K. Varghese, et al., J. Mater. Res., 2001, 16, 3331–3334 CrossRef CAS.
- J. M. Macak, H. Tsuchiya, L. Taveira, A. Ghicov and P. Schmuki, J. Biomed. Mater. Res., Part A, 2005, 75, 928–933 CrossRef PubMed.
- Z. Zhang and P. Wang, Energy Environ. Sci., 2012, 5, 6506–6512 CAS.
- K. Lee, A. Mazare and P. Schmuki, Chem. Rev., 2014, 114, 9385–9454 CrossRef CAS PubMed.
- C. Feng, Y. Pang, Y. Wang, M. Sun, C. Zhang, L. Zhang, Y. Zhou and D. Li, Appl. Surf. Sci., 2016, 376, 188–198 CrossRef CAS.
- L. Ma, H. Han, L. Pan, M. Tahir, L. Wang, X. Zhang and J.-J. Zou, RSC Adv., 2016, 6, 63984–63990 RSC.
- X. Yang, H. Cui, Y. Li, J. Qin, R. Zhang and H. Tang, ACS Catal., 2013, 3, 363–369 CrossRef CAS.
- N. Umezawa, O. Y. Shuxin and J. H. Ye, Phys. Rev. B: Condens. Matter Mater. Phys., 2011, 83, 8 CrossRef.
- Y. Bi, H. Hu, S. Ouyang, Z. Jiao, G. Lu and J. Ye, J. Mater. Chem., 2012, 22, 14847–14850 RSC.
- Y. Bi, H. Hu, S. Ouyang, G. Lu, J. Cao and J. Ye, Chem. Commun., 2012, 48, 3748–3750 RSC.
- H. Hu, Z. Jiao, T. Wang, J. Ye, G. Lu and Y. Bi, J. Mater. Chem. A, 2013, 1, 10612–10616 CAS.
- H. Hu, Z. Jiao, H. Yu, G. Lu, J. Ye and Y. Bi, J. Mater. Chem. A, 2013, 1, 2387–2390 CAS.
- C. Cui, Y. Qiu, H. Hu, N. Ma, S. Li, L. Xu, L. Chaorong, J. Xu and T. Weihua, RSC Adv., 2016, 6, 43697–43706 RSC.
- J. Li, X. Ji, X. Li, X. Hu, Y. Sun, J. Ma and G. Qiao, Appl. Surf. Sci., 2016, 372, 30–35 CrossRef CAS.
- W.-S. Wang, H. Du, R.-X. Wang, T. Wen and A.-W. Xu, Nanoscale, 2013, 5, 3315–3321 RSC.
- W. Teng, X. Li, Q. Zhao and G. Chen, J. Mater. Chem. A, 2013, 1, 9060–9068 CAS.
- J.-W. Xu, Z.-D. Gao, K. Han, Y. Liu and Y.-Y. Song, ACS Appl. Mater. Interfaces, 2014, 6, 15122–15131 CAS.
- F.-Q. Xiong, X. Wei, X. Zheng, D. Zhong, W.-H. Zhang and C. Li, J. Mater. Chem. A, 2014, 2, 4510–4513 CAS.
- X. Yang, J. Qin, Y. Jiang, K. Chen, X. Yan, D. Zhang, R. Li and H. Tang, Appl. Catal., B, 2015, 166–167, 231–240 CrossRef CAS.
- X. Yang, J. Qin, Y. Jiang, R. Li, Y. Li and H. Tang, RSC Adv., 2014, 4, 18627–18636 RSC.
- W. Yao, B. Zhang, C. Huang, C. Ma, X. Song and Q. Xu, J. Mater. Chem., 2012, 22, 4050–4055 RSC.
- H. Tang, Y. Fu, S. Chang, S. Xie and G. Tang, Chin. J. Catal., 2017, 38, 337–347 CrossRef CAS.
- X. Yang, Z. Chen, J. Xu, H. Tang, K. Chen and Y. Jiang, ACS Appl. Mater. Interfaces, 2015, 7, 15285–15293 CAS.
- D. Wang, L. Li, Q. Luo, J. An, X. Li, R. Yin and M. Zhao, Appl. Surf. Sci., 2014, 321, 439–446 CrossRef CAS.
- S. Bai, X. Li, Q. Kong, R. Long, C. Wang, J. Jiang and Y. Xiong, Adv. Mater., 2015, 27, 3444–3452 CrossRef CAS PubMed.
- X. Yang, H. Tang, J. Xu, M. Antonietti and M. Shalom, ChemSusChem, 2015, 8, 1350–1358 CrossRef CAS PubMed.
- C. Wagner, W. Riggs, L. Davis, J. Moulder and G. Muilenberg, Handbook of X-ray Photoelectron Spectroscopy, Perkin-Elmer Corp, 1979 Search PubMed.
- P. Wang, B. Huang, Z. Lou, X. Zhang, X. Qin, Y. Dai, Z. Zheng and X. Wang, Chem.–Eur. J., 2010, 16, 538–544 CrossRef CAS PubMed.
- P. Wang, B. Huang, Q. Zhang, X. Zhang, X. Qin, Y. Dai, J. Zhan, J. Yu, H. Liu and Z. Lou, Chem.–Eur. J., 2010, 16, 10042–10047 CrossRef CAS PubMed.
- B. Jin, X. Zhou, J. Luo, X. Xu, L. Ma, D. Huang, Z. Shao and Z. Luo, RSC Adv., 2015, 5, 48118–48123 RSC.
- B. Lu, N. Ma, Y. Wang, Y. Qiu, H. Hu, J. Zhao, D. Liang, S. Xu, X. Li, Z. Zhu and C. Cui, J. Alloys Compd., 2015, 630, 163–171 CrossRef CAS.
- J. S. Jang, C. W. Ahn, S. S. Won, J. H. Kim, W. Choi, B.-S. Lee, J.-H. Yoon, H. G. Kim and J. S. Lee, J. Phys. Chem. C, 2017, 121, 15063–15070 CAS.
- S. B. Rawal, S. D. Sung and W. I. Lee, Catal. Commun., 2012, 17, 131–135 CrossRef CAS.
- L. Zhang, H. Zhang, H. Huang, Y. Liu and Z. Kang, New J. Chem., 2012, 36, 1541–1544 RSC.
Footnote |
† Electronic supplementary information (ESI) available. See DOI: 10.1039/c7ra11283a |
|
This journal is © The Royal Society of Chemistry 2017 |
Click here to see how this site uses Cookies. View our privacy policy here.