DOI:
10.1039/C6SC05581H
(Edge Article)
Chem. Sci., 2017,
8, 2588-2591
Cobalt-catalysed C–H carbonylative cyclisation of aliphatic amides†
Received
20th December 2016
, Accepted 5th January 2017
First published on 10th January 2017
Abstract
A cobalt-catalysed C–H carbonylation of aliphatic carboxamide derivatives is described, employing commercially available Co(II)-salts in the presence of a silver oxidant. This operationally simple process utilises an atmospheric pressure of CO and generates a range of substituted succinimide products bearing diverse functional groups that can be successfully accessed via this methodology.
The development of novel transition-metal catalysed processes for the selective functionalisation of C–H bonds is an important challenge within synthetic chemistry, enabling efficient and streamlined routes to complex scaffolds.1 Whilst noble metals represent the most extensively explored catalyst class for C–H activation, the development of low-cost, 1st-row transition-metal alternatives is receiving considerable attention.2 Cobalt was the first of these elements to be investigated, with Murahashi et al. reporting a successful carbonylative cyclisation procedure of imine and azobenzene substrates as early as 1955 (Scheme 1A).3 Since this seminal report, strategies have been developed that directly employ commercially available and bench stable Co(II) salts for C–H activation reactions.4 Monoanionic, bidentate directing groups,5 such as the 8-aminoquinoline group first utilized by Daugulis, have been found to be essential for C–H activation to occur via these oxidative reaction platforms, and are believed to stabilise postulated high-valent Co-intermediates (Scheme 1A).6–8 Whilst this reactivity mode has been widely exploited for the functionalisation of sp2-hybridised C–H bonds, analogous C(sp3)–H activation procedures remain underdeveloped, with only two known examples reported to date. Ge and co-workers described efficient intra- and intermolecular amidation reactions,9a and the Zhang group subsequently developed an alkynylation/cyclisation procedure,9b affording substituted pyrrolidinone products in excellent yields (Scheme 1B).10 Both processes require the 8-aminoquinoline directing group to promote efficient reaction.
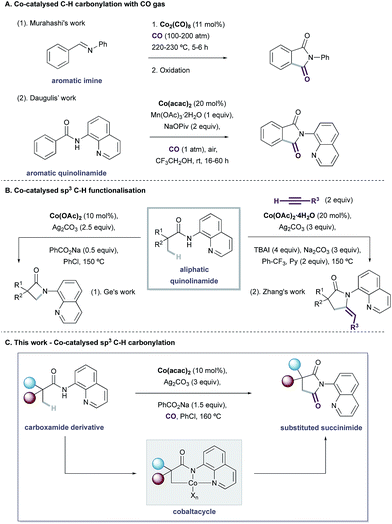 |
| Scheme 1 A Co-catalysed C–H carbonylation strategy. | |
Prompted by our interest in catalytic oxidative C–H carbonylation reactions,10–12 we reasoned that its merger with cobalt catalysis, guided by a pyridyl-derived auxiliary, would provide a distinct platform for C(sp3)–H activation using earth abundant metals. Herein, we report the development of an oxidative Co-catalysed carbonylative cyclisation procedure of aliphatic quinolinamides to yield a range of substituted succinimide products (Scheme 1C). The reaction proceeds under atmospheric pressures of CO using operationally simple conditions and accommodates a variety of versatile functional groups. During the submission of our manuscript, an elegant study was reported by Sundararaju and co workers, which detailed the same Co-catalyzed carbonylation process.13
At the outset of our studies, we prepared a range of pyridyl-derived pivaloylamide substrates (Scheme 2A) and exposed them to reaction conditions based on Ge's C–H amidation process (Co(OAc)2, sodium benzoate and Ag2CO3 in PhCl at 150 °C) under a CO atmosphere.9 We observed the formation of the desired C(sp3)–H carbonylation product in only one of these cases (Scheme 2B); quinolinamide 1a was converted to succinimide 7a with an 86% assay yield. The failure of any of the other substrates to undergo carbonylation highlights an important role of the 8-aminoquinoline group in controlling the C–H activation (Scheme 2B).
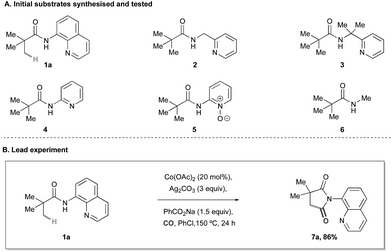 |
| Scheme 2 Investigation of substrate structure and lead reaction. | |
Next, we extensively assessed the reaction parameters of the successful process with the aim of further improving the yield as well as better understanding the role of each of the components. We found that a temperature of at least 150 °C was responsible for an effective reaction, with the yield of the process dramatically reduced upon lowering the temperature by only 15 °C (Table 1, entries 1–4). In the absence of the Co(II) catalyst, C–H carbonylation did not proceed, confirming the role of the metal species in mediating this process (entry 5). Replacement of sodium benzoate base with sodium acetate, sodium carbonate or sodium phosphate proved detrimental to the efficiency of the process, with succinimide 7a being formed in diminished 23%, 3% and 2% yield, respectively (entries 6–8). Surprisingly, we found that silver salts were essential for a successful reaction; only traces of the desired product were observed upon replacing Ag(I) oxidants with Mn(OAc)3·2H2O (entry 9), and complete loss of reactivity was observed when alternative inorganic oxidants were employed (entries 10–11). Although a variety of silver salts were tolerated by the reaction, silver carbonate proved to be the superior oxidant (see ESI†). A range of cobalt(II) salts were found to be successful pre-catalysts for C–H carbonylation. Whilst a drop in efficiency was observed for CoBr2, a modest increase in yield of 7a to 91% was observed upon employing Co(acac)2 (entries 13–14). Lowering the catalyst concentration from 20 mol% to 10 mol% was tolerated by increasing the reaction temperature to 160 °C, allowing succinimide 7a to be observed in 94% assay yield, which could be isolated in 89% yield (entry 15). Reducing the catalyst loading to 5 mol% did not aid the reaction, and lowering the amount of the silver salt in the reaction was deleterious to the yield (entries 16–18).
Table 1 Reaction optimisationa

|
Entrya |
Co, mol% |
Base |
Oxidant |
T °C |
Yieldb (%) |
Starting reaction conditions: substrate 1 (0.2 mmol), Co source (10 mol%), base (1.5 equiv.), oxidant (3.0 equiv.), PhCl (2 mL), CO (1 atm), 21 h.
The yield was determined by 1H NMR analysis of the crude reaction mixture using 1,1,2,2-tetrachloroethane as the internal standard.
|
1 |
Co(OAc)2 |
PhCO2Na |
Ag2CO3 |
150 |
86 |
2 |
Co(OAc)2 |
PhCO2Na |
Ag2CO3 |
140 |
72 |
3 |
Co(OAc)2 |
PhCO2Na |
Ag2CO3 |
135 |
40 |
4 |
Co(OAc)2 |
PhCO2Na |
Ag2CO3 |
130 |
18 |
5 |
No Co |
PhCO2Na |
Ag2CO3 |
150 |
0 |
6 |
Co(OAc)2 |
NaOAc |
Ag2CO3 |
150 |
23 |
7 |
Co(OAc)2 |
Na2CO3 |
Ag2CO3 |
150 |
3 |
8 |
Co(OAc)2 |
Na3PO4 |
Ag2CO3 |
150 |
2 |
9 |
Co(OAc)2 |
PhCO2Na |
Mn(OAc)3 |
150 |
6 |
10 |
Co(OAc)2 |
PhCO2Na |
NaClO3 |
150 |
3 |
11 |
Co(OAc)2 |
PhCO2Na |
CAN |
150 |
6 |
12 |
Co(OAc)2 |
PhCO2Na |
No Ag |
150 |
0 |
13 |
CoBr2 |
PhCO2Na |
Ag2CO3 |
150 |
10 |
14 |
Co(acac)2 |
PhCO2Na |
Ag2CO3 |
150 |
91 |
15
|
10 mol%
|
PhCO
2
Na
|
Ag
2
CO
3
|
160
|
94
|
16 |
5 mol% |
PhCO2Na |
Ag2CO3 |
160 |
88 |
17 |
5 mol% |
PhCO2Na |
2.0 equiv. |
160 |
78 |
18 |
5 mol% |
PhCO2Na |
1.5 equiv. |
160 |
72 |
With a set of optimal conditions in hand, we next assessed the scope of the Co-catalyzed C–H carbonylation reaction (Table 2). We found that simple alkyl-derived carboxamides were effective substrates for this reaction affording the corresponding succinimide products in high yields. It is interesting to note that on reaction of 1c and 1d, displaying phenyl groups at both the α- and β-position with respect to the carbonyl motif, complete selectivity was observed for C(sp3)–H activation at the methyl groups over traditionally more reactive sp2-hybridised C–H bonds to form 7c and 7d. Although similar selectivities were observed in a related Ni-catalysed C–H carbonylation reaction,14 this represents a rare example of competitive aliphatic over aromatic C–H activation. Spirocyclic quinolinamides comprised of a cyclohexane or substituted piperidine moiety also provided synthetically useful yields of the desired succinimide products 7f and 7g.
Table 2 Reaction scopea,b
80% on 5.00 mmol scale.
20 mol% Co(acac)2 used.
|
|
Substrates containing sensitive ester functionalities, as well as electron-withdrawing trifluoromethyl groups were all accommodated by the reaction, affording the succinimides in 51–80% yield (7g–7i). Succinimide 7j could also be prepared in moderate yield by the C–H carbonylation of a protected α-amino acid derivative. Selective methylene C–H activation onto a cyclopropyl group provided the bicyclic succinimide products in reasonable yields (7k–7n). Interestingly, the butyramide-derived substrate,10 displaying a partially substituted carbon atom between the carbonyl group and the site of C–H activation successfully underwent C–H carbonylation in 66% yield, to succinimide 7o; this substrate was unreactive under Ge's Co-catalysed C–H amidation procedure.9a Alanine derived 7p, containing a protected amine group, was also successfully prepared in 55% yield. We found that the Co-catalyzed carbonylation process performed well on a larger, 5 mmol scale to give an 80% yield of the succinimide product 7a.
Finally, we demonstrated that the succinimide products were compatible with further derivatisation: reductive ring opening of 7c with LiAlH4 generated amino-alcohol 8 in 66% yield; hydrolysis of succinimide 7c with 3 M aqueous hydrochloric acid led to the formation of substituted succinic acid 9 in 88% yield, with release of the 8-aminoquinoline directing group; and treatment of 7c with morpholine afforded the ring-opened bis-amide 10 in 77% yield with complete regioselectivity (Scheme 3).
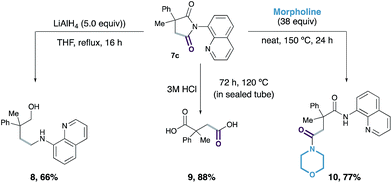 |
| Scheme 3 Product derivatisations. | |
Conclusions
In summary, we have developed a Co-catalysed carbonylative cyclisation procedure of unactivated, aliphatic C–H bonds. Central to the success of this procedure is the stabilising effect of the quinolinamide directing group. The process tolerates a range of functionalised substrates to generate substituted succinimide products. Importantly, the operationally simple reaction conditions are complemented by the ability utilise an atmospheric pressure of carbon monoxide. While the mechanism of this process remains unclear with respect to the catalytic function of the cobalt salts, an elucidation of the role of the essential stoichiometric Ag additives will also be crucial.15 Moreover, to fully realise the full synthetic potential of C–H activation with earth abundant catalysts, an important challenge will be to develop processes that do not rely on precious metal additives and bespoke directing auxiliaries. On going mechanistic studies should reveal further opportunities refine these transformations and lead to the development of new efficient Co-catalyzed C–H activation reactions.
Acknowledgements
We are grateful to the Herchel Smith Foundation for a scholarship (P. W.) and the Royal Society (Wolfson Merit Award, M. J. G) and the EPSRC (EP/100548X/1) and ERC (ERC-StG-259711) for Fellowships (M. J. G). Mass spectrometry data were acquired at the EPSRC UK National Mass Spectrometry Facility at Swansea University.
Notes and references
-
(a) J. Yamaguchi, A. D. Yamaguchi and K. Itami, Angew. Chem., Int. Ed., 2012, 51, 8960–9009 CrossRef CAS PubMed
;
(b) T. W. Lyons and M. S. Sanford, Chem. Rev., 2010, 110, 1147–1169 CrossRef CAS PubMed
;
(c) I. A. I. Mkhalid, J. H. Barnard, T. B. Marder, J. M. Murphy and J. F. Hartwig, Chem. Rev., 2010, 110, 890–931 CrossRef CAS PubMed
;
(d) J. Wencel-Delord, T. Dröge, F. Liu and F. Glorius, Chem. Soc. Rev., 2011, 40, 4740–4761 RSC
;
(e) R. Jazzar, J. Hitce, A. Renaudat, J. Sofack-Kreutzer and O. Baudoin, Chemistry, 2010, 16, 2654–2672 CrossRef CAS PubMed
;
(f) R.-Y. Zhu, M. E. Farmer, Y.-Q. Chen and J. Q. Yu, Angew. Chem., Int. Ed., 2016, 55, 10578–10599 CrossRef CAS PubMed
;
(g) K. Gao and N. Yoshikai, Acc. Chem. Res., 2014, 47, 1208 CrossRef CAS PubMed
;
(h) D. A. Colby, R. G. Bergmann and J. A. Ellman, Chem. Rev., 2010, 110, 624–655 CrossRef CAS PubMed
.
- Selected recent reviews on first row transition metal catalysed C-H bond functionalization see:
(a) D. Wei, X. Zhu, J.-L. Niu and M.-P. Song, ChemCatChem, 2016, 8, 1242–1263 CrossRef CAS
;
(b) N. Moselage, J. Li and L. Ackermann, ACS Catal., 2016, 6, 498–525 CrossRef
;
(c) N. Yoshikai, ChemCatChem, 2015, 7, 732–734 CrossRef CAS
;
(d) T. Hyster, Catal. Lett., 2015, 145, 458–467 CrossRef CAS
;
(e) I. Bauer and H. Knölker, Chem. Rev., 2015, 115, 3170–3387 CrossRef CAS PubMed
;
(f) S. Z. Tasker, E. A. Standley and T. F. Jamison, Nature, 2014, 509, 299–309 CrossRef CAS PubMed
;
(g) A. A. Kulkarni and O. Daugulis, Synthesis, 2009, 4087–4109 CAS
.
- S. Murahashi, J. Am. Chem. Soc., 1955, 77, 6403–6404 CrossRef CAS
.
- For selected references on high valent cobalt catalyzed C(sp3)–H bond functionalization see:
(a) S. Prakash, K. Muralirajan and C.-H. Cheng, Angew. Chem., Int. Ed., 2016, 55, 1844–1848 CrossRef CAS PubMed
;
(b) C. Du, P.-X. Li, X. Zhu, J.-F. Suo, J.-L. Niu and M.-P. Song, Angew. Chem., Int. Ed., 2016, 55, 13571–13575 CrossRef CAS PubMed
;
(c) S. Maity, R. Kancherla, U. Dhawa, E. Hoque, S. Pimparkar and D. Maiti, ACS Catal., 2016, 6, 5493–5499 CrossRef
;
(d) A. Lerchen, S. Vásquez-Céspedes and F. Glorius, Angew. Chem., Int. Ed., 2016, 55, 3208–3211 CrossRef CAS PubMed
;
(e) G. Tan, S. He, X. Huang, X. Liao, Y. Cheng and J. You, Angew. Chem., Int. Ed., 2016, 55, 10414–10418 CrossRef CAS PubMed
;
(f) R. Manoharan, G. Sivakumar and M. Jeganmohan, Chem. Commun., 2016, 52, 10533–10536 RSC
;
(g) T. Yamaguchi, Y. Kommagalla, Y. Aihara and N. Chatani, Chem. Commun., 2016, 52, 10129–10132 RSC
;
(h) L. Kong, S. Yu, X. Zhou and X. Li, Org. Lett., 2016, 18, 588–591 CrossRef CAS PubMed
;
(i) J. R. Hummel and J. A. Ellman, J. Am. Chem. Soc., 2015, 137, 490–498 CrossRef CAS PubMed
;
(j) P. Patel and S. Chang, ACS Catal., 2015, 5, 853–858 CrossRef CAS
;
(k) H. Wang, J. Koeller, W. Liu and L. Ackermann, Chem.–Eur. J., 2015, 21, 15525–15528 CrossRef CAS PubMed
;
(l) Z.-Z. Zhang, L. Bin, C.-Y. Wang and B.-F. Shi, Org. Lett., 2015, 17, 4094–4097 CrossRef CAS PubMed
;
(m) T. Yoshino, H. Ikemoto, S. Matsunaga and M. Kanai, Angew. Chem., Int. Ed., 2013, 52, 2207–2211 CrossRef CAS PubMed
;
(n) L. Grigorjeva and O. Daugulis, Org. Lett., 2014, 16, 4688–4690 CrossRef CAS PubMed
.
- V. G. Zaitsev, D. Shabashov and O. Daugulis, J. Am. Chem. Soc., 2005, 127, 13154–13155 CrossRef CAS PubMed
.
- L. Grigorjeva and O. Daugulis, Angew. Chem., Int. Ed., 2014, 53, 10209–10212 CrossRef CAS PubMed
.
-
(a) Y. Aihara and N. Chatani, J. Am. Chem. Soc., 2014, 136, 898–901 CrossRef CAS PubMed
;
(b) Y. Aihara, M. Tobisu, Y. Fukumoto and N. Chatani, J. Am. Chem. Soc., 2014, 136, 15509–15512 CrossRef CAS PubMed
;
(c) T. Kubo, Y. Aihara and N. Chatani, Chem. Lett., 2015, 44, 1365–1367 CrossRef CAS
.
- Selected recent reviews on the use of 8-aminoquinoline as a directing group for 1st row metals, see:
(a) L. C. Misal Castro and N. Chatani, Chem. Lett., 2015, 44, 410–421 CrossRef
;
(b) G. Rouquet and N. Chatani, Angew. Chem., Int. Ed., 2013, 52, 11726–11743 CrossRef CAS PubMed
;
(c) M. Corbet and F. Decampo, Angew. Chem., Int. Ed., 2013, 52, 9896–9898 CrossRef CAS PubMed
; see also
(d) T. Matsubara, S. Asako, E. IIies and E. Nakamura, J. Am. Chem. Soc., 2014, 136, 646–649 CrossRef CAS PubMed
.
-
(a) X. Wu, K. Yang, Y. Zhao, H. Sun, G. Li and H. Ge, Nat. Commun., 2015, 6, 6462–6471 CrossRef CAS PubMed
;
(b) J. Zhang, H. Chen, C. Lin, Z. Liu, C. Wang and Y. Zhang, J. Am. Chem. Soc., 2015, 137, 12990–12996 CrossRef CAS PubMed
.
- For reviews of oxidative carbonylation reactions, see
(a)
M. Beller, Catalytic carbonylation reactions, Topic in organometallic Chemistry, Springer, 2006, pp. 1–283 Search PubMed
;
(b) X.-F. Wu, H. Neumann and M. Beller, ChemSusChem, 2013, 6, 229–241 CrossRef CAS PubMed
;
(c) X.-F. Wu, H. Neumann and M. Beller, Chem. Rev., 2013, 113, 1–35 CrossRef CAS PubMed
;
(d) S. T. Gadge, P. Gautam and B. M. Bhanage, Chem. Rec., 2016, 16, 835–856 CrossRef CAS PubMed
.
- For selected examples of aliphatic catalytic C–H carbonylation processes, see
(a) T. Sakakura, T. Sodeyama, K. Sasaki, K. Wada and M. Tanaka, J. Am. Chem. Soc., 1990, 112, 7221–7229 CrossRef CAS
;
(b) N. Chatani, T. Asaumi, T. Ikeda, S. Yorimitsu, Y. Ishii, F. Kakiuchi and S. Murai, J. Am. Chem. Soc., 2000, 122, 12882–12883 CrossRef CAS
;
(c) E. J. Yoo, M. Wasa and J.-Q. Yu, J. Am. Chem. Soc., 2010, 132, 17378–17380 CrossRef CAS PubMed
;
(d) N. Hasegawa, V. Charra, S. Inoue, Y. Fukumoto and N. Chatani, J. Am. Chem. Soc., 2011, 133, 8070–8073 CrossRef CAS PubMed
;
(e) N. Hasegawa, K. Shibata, V. Charra, S. Inoue, Y. Fukumoto and N. Chatani, Tetrahedron, 2013, 69, 4466–4472 CrossRef CAS
;
(f) P.-L. Wang, Y. Li, Y. Wu, C. Li, Q. Lan and X.-S. Wang, Org. Lett., 2015, 17, 3698–3701 CrossRef CAS PubMed
;
(g) C. Wang, L. Zhang, C. Chen, J. Han, Y. Yao and Y. Zhao, Chem. Sci., 2015, 6, 4610–4614 RSC
;
(h) P. Xie, Y. Xie, B. Qian, H. Zhou, C. Xia and H. Huang, J. Am. Chem. Soc., 2012, 134, 9902–9905 CrossRef CAS PubMed
.
- For examples of catalytic aliphatic C–H carbonylation from our group, see:
(a) A. McNally, B. Haffemayer, B. S. L. Collins and M. J. Gaunt, Nature, 2014, 510, 129–133 CrossRef CAS PubMed
;
(b) J. Calleja, D. Pla, T. Gorman, V. Dominguez, B. Haffameyer and M. J. Gaunt, Nat. Chem., 2015, 7, 1009 CrossRef CAS PubMed
;
(c) D. Willcox, B. G. N. Chappell, K. F. Hogg, J. Calleja, A. P. Smalley and M. J. Gaunt, Science, 2016, 354, 851 CrossRef CAS PubMed
.
- Concurrent with the submission of our manuscript, a similar paper on Co-catalyzed C–H carbonylation was reported; see N. Barsu, S. K. Bolli and B. Sundararaju, Chem. Sci., 2017, 8 10.1039/C6SC05026C
.
-
(a) X. Wu, J. Miao, Y. Li, G. Li and H. Ge, Chem. Sci., 2016, 7, 5260–5264 RSC
;
(b) X. Wu, Y. Zhao and H. Ge, J. Am. Chem. Soc., 2015, 137, 4924–4927 CrossRef CAS PubMed
.
- For the role of Ag additives in C–H activation; see
(a) M. D. Lotz, N. M. Camasso, A. J. Canty and M. S. Sanford, Organometallics, 2017, 36, 165–171 CrossRef CAS
;
(b) D. Whitaker, J. Bures and I. Larrosa, J. Am. Chem. Soc., 2016, 138, 8384 CrossRef CAS PubMed
.
Footnotes |
† Electronic supplementary information (ESI) available. See DOI: 10.1039/c6sc05581h |
‡ Equal contribution of authors. |
|
This journal is © The Royal Society of Chemistry 2017 |
Click here to see how this site uses Cookies. View our privacy policy here.