DOI:
10.1039/C7SC00499K
(Edge Article)
Chem. Sci., 2017,
8, 4465-4474
Tris[(1-isopropylbenzimidazol-2-yl)dimethylsilyl]methyl metal complexes, [TismPriBenz]M: a new class of metallacarbatranes, isomerization to a tris(N-heterocyclic carbene) derivative, and evidence for an inverted ligand field†
Received
2nd February 2017
, Accepted 27th March 2017
First published on 2nd May 2017
Abstract
The tris[(1-isopropylbenzimidazol-2-yl)dimethylsilyl]methyl ligand, [TismPriBenz], has been employed to form carbatrane compounds of both the main group metals and transition metals, namely [TismPriBenz]Li, [TismPriBenz]MgMe, [TismPriBenz]Cu and [TismPriBenz]NiBr. In addition to the formation of atranes, a zinc compound that exhibits κ3-coordination, namely [κ3-TismPriBenz]ZnMe, has also been obtained. Furthermore, the [TismPriBenz] ligand may undergo a thermally induced rearrangement to afford a novel tripodal tris(N-heterocyclic carbene) variant, as shown by the conversion of [TismPriBenz]Cu to [κ4-C4-TismPriBenz*]Cu. The transannular M–C bond lengths in the atrane compounds are 0.19–0.32 Å longer than the sum of the respective covalent radii, which is consistent with a bonding description that features a formally zwitterionic component. Interestingly, computational studies demonstrate that the Cu–Catrane interactions in [TismPriBenz]Cu and [κ4-C4-TismPriBenz*]Cu are characterized by an “inverted ligand field”, in which the occupied antibonding orbitals are localized more on carbon than on copper.
Introduction
Atranes comprise an interesting class of molecules in which two bridgehead atoms are joined by three three-atom linkers, thereby resulting in a tricyclic motif.1–3 A relatively recent development in this area is concerned with the synthesis of metallacarbatranes that feature transannular M–C interactions.4 Such compounds are of interest because the M–C bond corresponds to an M–X interaction, in contrast to the transannular M←L4,5 or M→Z4,6 dative bonds that are more commonly encountered in atranes (Fig. 1).7,8 For example, we have recently employed tris(2-pyridylthio)methyl ([Tptm])3,9 and tris(1-methylimidazol-2-ylthio)methyl ([TitmMe])10 as ligands for the construction of metallacarbatranes,11 and have demonstrated that the nature of the heterocyclic nitrogen donor has an impact on the structure of the carbatrane.10 Since a common feature of these ligands is the attachment of the heterocycles to the carbon bridgehead via a sulfur atom, we considered it worthwhile to investigate a different type of linker. Therefore, we report here a new class of tetradentate tripodal ligands in which three imidazole donors are attached to a carbon bridgehead via C–Si linkages. In addition, we also describe isomerization of the tris(imidazole) ligand to afford a novel tripodal tris(N-heterocyclic carbene) derivative.
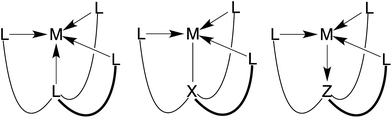 |
| Fig. 1 Three types of atrane molecules classified according to whether the transannular interaction involves an L, X or Z-type binding site. | |
Results and discussion
We considered the [Me2Si] moiety to be an appealing linker for the construction of analogues of the above tetradentate tripodal ligands because (i) C–Si bonds are typically robust,12 (ii) methyl substituents on silicon can provide a protective environment for the bridgehead carbon, and (iii) silyl groups lower the pKa of adjacent C–H groups,13 thereby facilitating protolytic cleavage. Furthermore, tripodal molecules of the type HC(SiMe2X)3 are known, e.g. X = NR,14 PR2,15 CH2PR2,16 S,17 Se,17 and OC2H4OMe,18 and thus provide a precedent for the synthesis of variants that include heterocyclic nitrogen donors.
Indeed, tris[(1-isopropylbenzimidazol-2-yl)dimethylsilyl]-methane, [TismPriBenz]H, and the lithium derivative, [TismPriBenz]Li, may be obtained from 1-isopropylbenzimidazole and HC(SiMe2Cl)3via the sequence illustrated in Scheme 1.19 Specifically, treatment of 1-isopropylbenzimidazole with MeLi, followed by addition of HC(SiMe2Cl)3, affords [TismPriBenz]Li, which is converted to [TismPriBenz]H upon reaction with H2O;20 [TismPriBenz]Li may also be regenerated by treatment of [TismPriBenz]H with BunLi.
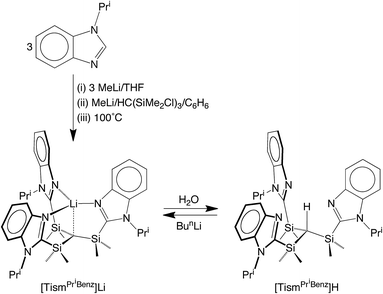 |
| Scheme 1 | |
The molecular structure of [TismPriBenz]Li has been determined by X-ray diffraction (Fig. 2), thereby revealing that the compound possesses an atrane motif21 in which the lithium adopts an approximately trigonal monopyramidal22 coordination environment with N–Li–N and C–Li–N bond angles of 118.61(9)° and 96.8(2)°.23,24 Trigonal monopyramidal coordination is not common for lithium, but a similar coordination environment is observed for tris(2-pyridylthio)methyllithium, [Tptm]Li.3,25 An interesting difference between [TismPriBenz]Li and [Tptm]Li, however, pertains to the geometry at the bridgehead carbon atom. Specifically, the [CSi3] moiety of [TismPriBenz]Li adopts a much greater degree of planarity (Table 1) than does the [CS3] moiety of [Tptm]Li, as indicated by the fact that the sum of the Si–C–Si angles of [TismPriBenz]Li (355.8°)26 is much closer to 360° than is the sum of the S–C–S angles of [Tptm]Li (345.2°). Furthermore, the Li–C–Si angles of [TismPriBenz]Li [96.87(13)°] are closer to 90° than are the Li–C–S angles of [Tptm]Li [103.05(7)°].27,28 Since silyl-substituted carbanions are close to planar,29 as illustrated by [Li(tmen)2][C(SiMe2PPh2)3],30,31,32 the planarity of the [CSi3] moiety of [TismPriBenz]Li suggests that the molecule possesses a significant degree of formally zwitterionic character in which carbon bears a negative charge.4,33–35 In support of this suggestion, while the Li–N bond lengths [2.017(2) Å] are comparable to the sum of the covalent radii (1.99 Å),36 the Li–C bond length [2.273(9) Å] is distinctly longer (by 0.23 Å) than the sum of covalent radii (2.04 Å).36,37 Moreover, the HOMO of [TismPriBenz]Li is largely composed of a p-orbital on carbon, similar to that of the planar [TismPriBenz]− anion with a comparable conformation (Fig. 3).
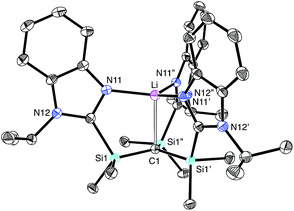 |
| Fig. 2 Molecular structure of [TismPriBenz]Li. | |
Table 1 Metrical data for [TismPriBenz]M derivatives
|
d(M–C)/Å |
d(M–C) − ∑(cov. radii)a |
∑(Si–C–Si)/° |
d(C–[Si3])b/Å |
Ref. 36.
Distance of bridgehead carbon from the [Si3] plane.
|
[TismPriBenz]Li |
2.273(9) |
0.23 |
355.8 |
0.22 |
[TismPriBenz]MgMe |
2.4925(12) |
0.32 |
347.8 |
0.37 |
[κ3-TismPriBenz]ZnMe |
2.171(3) |
0.19 |
346.3 |
0.40 |
[TismPriBenz]Cu |
2.281(7) |
0.20 |
355.2 |
0.23 |
[κ4-C4-TismPriBenz*]Cu |
2.4283(18) |
0.35 |
357.8 |
0.15 |
[TismPriBenz]NiBr |
2.2197(16) |
0.22 |
347.7 |
0.38 |
[TismPriBenz]H |
— |
— |
342.3 |
0.47 |
 |
| Fig. 3 Comparison of the HOMO of [TismPriBenz]Li (top) and [TismPriBenz]− (bottom). | |
[TismPriBenz]H and [TismPriBenz]Li may be employed to form carbatrane compounds of the main group and transition metals. For example, [TismPriBenz]H reacts with Me2Mg via elimination of methane to afford [TismPriBenz]MgMe (Scheme 2). The molecular structure of [TismPriBenz]MgMe has been determined by X-ray diffraction (Fig. 4), thereby demonstrating that the [TismPriBenz] ligand coordinates in a κ4-manner such that the molecule possesses a carbatrane motif,21 but with a Mg–Catrane distance [2.4925(12) Å] that is significantly longer (by 0.32 Å) than both (i) the Mg–CH3 bond length [2.1781(13) Å] and (ii) the sum of covalent radii (2.17 Å).36,38,39 The long Mg–Catrane distance is, nevertheless, consistent with a zwitterionic description in which the carbon atom bears a formal negative charge. This qualitative view of the bonding is supported by computational studies which demonstrate that the HOMO-1 is effectively a lone pair orbital on carbon, with very little contribution from magnesium (Fig. 5).40 As such, the HOMO-1 of [TismPriBenz]MgMe is similar in nature to the HOMO of [TismPriBenz]Li. Despite the comparable atrane motifs of [TismPriBenz]MgMe and [TismPriBenz]Li, however, a notable difference is that the [CSi3] moiety of [TismPriBenz]MgMe is more pyramidal than that of [TismPriBenz]Li, as indicated by the fact that the sum of the Si–C–Si angles of [TismPriBenz]MgMe (347.8°) is smaller than that for [TismPriBenz]Li (355.8°).
 |
| Scheme 2 | |
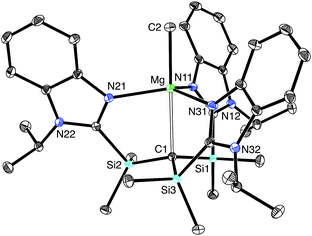 |
| Fig. 4 Molecular structure of [TismPriBenz]MgMe. | |
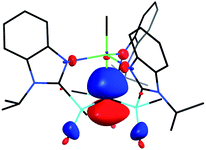 |
| Fig. 5 HOMO-1 of [TismPriBenz]MgMe. | |
[TismPriBenz]H can also be used as a reagent in zinc chemistry. Thus, [TismPriBenz]H reacts with Me2Zn to afford [κ3-TismPriBenz]ZnMe (Scheme 2). Although the reaction is analogous to that between [TismPriBenz]H and Me2Mg, X-ray diffraction demonstrates that the zinc product, [κ3-TismPriBenz]ZnMe, has a notably different structure to that of the magnesium counterpart. Specifically, rather than coordinating to zinc in a κ4-manner to afford a carbatrane motif, the ligand binds to zinc in a hypodentate41 κ3-manner, such that one of the imidazolyl groups remains uncoordinated (Fig. 6).42–44 Also in contrast to the magnesium derivative, [TismPriBenz]MgMe, for which the Mg–CH3 and Mg–Catrane bond lengths are very different, the corresponding bonds for [κ3-TismPriBenz]ZnMe are more similar: d(Zn–CH3) = 1.989(3) Å and d(Zn–Catrane) = 2.171(3)Å, and the former is comparable to the sum of covalent radii (1.98 Å).36,45,46
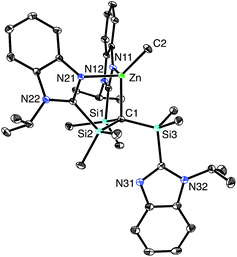 |
| Fig. 6 Molecular structure of [κ3-TismPriBenz]ZnMe. | |
Density functional theory (DFT) calculations on the isomeric forms of [TismPriBenz]MgMe and [TismPriBenz]ZnMe support the experimental observations. Specifically, the DFT calculations demonstrate that the κ4-isomer is 1.94 kcal mol−1 more stable than the κ3-isomer for [TismPriBenz]MgMe, whereas the κ3-isomer is 3.39 kcal mol−1 more stable than the κ4-isomer for [TismPriBenz]ZnMe (Fig. 7).
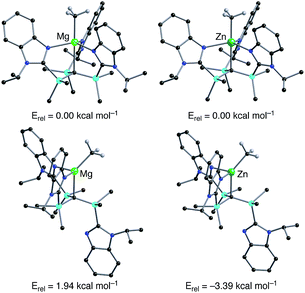 |
| Fig. 7 Relative energies of geometry optimized structures of κ3- and κ4-[TismPriBenz]MMe (M = Mg, Zn). Hydrogen atoms on [TismPriBenz] are omitted for clarity. | |
The lithium compound, [TismPriBenz]Li, has also been used to synthesize metal complexes via metathesis reactions involving metal halides. For example, [TismPriBenz]Li reacts with [(Me3P)CuCl]4 to give [TismPriBenz]Cu (Scheme 3), which has been shown by X-ray diffraction (Fig. 8) to possess a trigonal monopyramidal structure similar to that of [TismPriBenz]Li, with Cu–C and Cu–N distances of 2.281(7) Å and 2.014(3) Å, respectively.47,48 As with the lithium and magnesium carbatranes, the Cu–Catrane bond of [TismPriBenz]Cu is also longer (by 0.20 Å) than the sum of covalent radii (2.08 Å).36,49,50
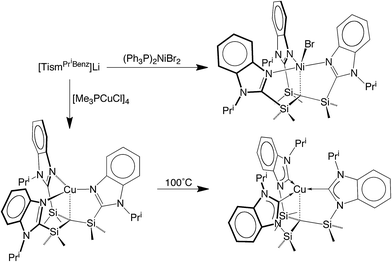 |
| Scheme 3 | |
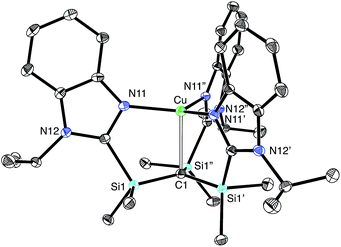 |
| Fig. 8 Molecular structure of [TismPriBenz]Cu. | |
A distinct difference between [TismPriBenz]Cu and the lithium and magnesium complexes, [TismPriBenz]Li and [TismPriBenz]MgMe, however, is the degree of covalent interaction between copper and the atrane carbon atom. Specifically, overlap between the carbon 2pz orbital and the copper 3dz2 orbital gives rise to Cu–C bonding and antibonding combinations, the latter of which is the HOMO (Fig. 9), as illustrated in the qualitative molecular orbital diagram shown in Fig. 10. Interestingly, the bonding combination possesses a significant copper component, while the antibonding combination possesses a significant carbon component; indeed, a natural bond orbital analysis of [TismPriBenz]Cu classifies the HOMO as a carbon lone pair orbital. This arrangement is counter to that observed for most transition metal compounds with σ-donor ligands, for which the bonding combination usually possesses more ligand character because the ligand orbitals are typically lower in energy than the metal orbitals.51,52
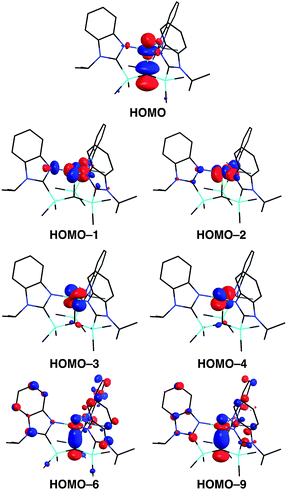 |
| Fig. 9 Frontier orbitals of [TismPriBenz]Cu. Note that the in-phase interaction between the carbon 2pz orbital and the copper 3dz2 orbital is a component of two molecular orbitals with similar energies (HOMO-6, −0.2169 eV; HOMO-9, −0.2192 eV). | |
 |
| Fig. 10 Qualitative molecular orbital diagram for [TismPriBenz]Cu with C3v symmetry, with the ligand arbitrarily represented in its neutral form. | |
However, despite the fact that situations in which the bonding orbital possesses mainly metal character (and the corresponding antibonding orbital possesses mainly ligand character) are not normally encountered in transition metal chemistry, examples of so-called “inverted ligand fields” have recently been discussed.51 Such circumstances may arise when the ligand σ-orbitals are higher in energy than the metal d orbitals, an occurrence that is more likely at the end of the transition series.51 A salient example is provided by [Cu(CF3)4]−, for which the bonding has been investigated both experimentally and computationally.51,53–55
Most interestingly, [TismPriBenz]Cu undergoes a novel isomerization at 100 °C to afford a tris(N-heterocyclic carbene) derivative, [κ4-C4-TismPriBenz*]Cu (Scheme 3),56 which has been structurally characterized by X-ray diffraction (Fig. 11).
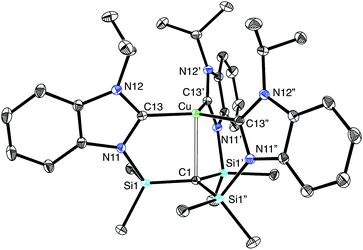 |
| Fig. 11 Molecular structure of [κ4-C4-TismPriBenz*]Cu. | |
Although several tripodal tris(N-heterocyclic carbene) ligands have been reported,57–59 the formation of [κ4-C4-TismPriBenz*]Cu is notable because [κ4-C4-TismPriBenz*] is an example of such a ligand that also features an additional potential X-type7 binding site.60 The isomerization of [TismPriBenz]Cu to [κ4-C4-TismPriBenz*]Cu is, however, accompanied by an increase in the axial Cu–Catrane distance from 2.281(7) Å to 2.4283(18) Å, a value that is 0.35 Å longer than the sum of the covalent radii.36,61 Despite this lengthening, the copper 3dz2 and carbon 2pz orbitals interact, and the derived bonding and antibonding orbitals are illustrated in Fig. 12. As observed for [TismPriBenz]Cu, the antibonding orbital possesses mainly carbon character such that the bonding situation also corresponds to an “inverted ligand field”.
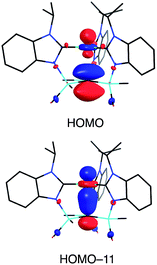 |
| Fig. 12 The HOMO and HOMO-11 of [κ4-C4-TismPriBenz*]Cu. | |
DFT calculations indicate that [κ4-C4-TismPriBenz*]Cu is more stable than [TismPriBenz]Cu by 4.18 kcal mol−1, which is in accord with the experimental observations. In contrast, isomerization of the structurally analogous lithium derivative, [TismPriBenz]Li, to [κ4-C4-TismPriBenz*]Li is predicted to be thermodynamically unfavorable by 35.3 kcal mol−1.62 The different thermodynamic trends reflect, inter alia, (i) the intrinsic stability of [TismPriBenz] versus [TismPriBenz*] and (ii) the relative preferences of copper and lithium to coordinate to a N-heterocyclic carbene versus an imidazole donor.63 With respect to the former, the tris(N-heterocyclic carbene), [TismPriBenz*]H, is calculated to be 41.1 kcal mol−1 higher in energy than the tris(imidazole), [TismPriBenz]H, with the 3
:
0 conformation64 that is used for κ4-coordination.65,66 As such, it is evident that coordination of the copper to the carbon donors provides a driving force for the isomerization.67 While the tautomerization of imidazoles to a C-coordinated ligand at a metal center has been previously observed,68–70 we are unaware of the corresponding transformation involving migration of a silyl group rather than a hydrogen atom.71 Furthermore, the formation of [κ4-C4-TismPriBenz*]Cu is also noteworthy because C-coordination has been predicted to be less favorable than N-coordination of imidazole to CuCl.66a,b
The nickel compound, [TismPriBenz]NiBr, may be obtained via metathesis of [TismPriBenz]Li with (Ph3P)2NiBr2 (Scheme 3). Although the [TismPriBenz] ligand binds in a κ4-manner (Fig. 13), with a Ni–C bond length of 2.2197(16) Å and Ni–N bond lengths in the range 2.0093(14)–2.1230(14) Å,72 the molecule does not adopt a trigonal bipyramidal structure akin to that of [TismPriBenz]MgMe. Specifically, rather than possess three N–Ni–N angles of approximately 120°, the three nitrogen atoms of [TismPriBenz]NiBr adopt a T-shaped arrangement, with N–Ni–N bond angles of 89.36(5)°, 90.34(6)° and 174.72(5)°; despite the different placement of the imidazole donors, however, the three C–Ni–N angles retain values of approximately 90°, namely 87.18(6)°, 87.63(6)° and 96.75(6)°.72 Thus, in addition to coordinating with a local C3 geometry, the [TismPriBenz] ligand is flexible and may also coordinate with an idealized 90° seesaw geometry.23 Although the latter coordination mode of the [TismPriBenz] ligand could support a square pyramidal structure, the location of the bromine is such that the coordination geometry of nickel is intermediate between square pyramidal and trigonal bipyramidal,73 as indicated by a τ5 five coordinate index of 0.44.74–76 As observed for the above carbatrane compounds, the Ni–C bond length [2.2197(16) Å] is also longer (by 0.22 Å) than the sum of covalent radii (2.00 Å).36,77
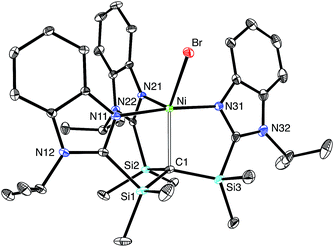 |
| Fig. 13 Molecular structure of [TismPriBenz]NiBr. | |
Conclusions
In summary, [TismPriBenz] is a flexible ligand that can coordinate to a metal center in both κ3 and κ4-manners, with the latter affording a carbatrane motif. Furthermore, when coordinating in a κ4-manner, the [TismPriBenz] ligand can adopt either a trigonal monopyramidal geometry or a seesaw geometry. Interestingly, we have also demonstrated that the [TismPriBenz] ligand may undergo a thermally induced rearrangement to afford a novel tripodal tris(N-heterocyclic carbene) ligand, as demonstrated by the conversion of [TismPriBenz]Cu to [κ4-C4-TismPriBenz*]Cu. A notable feature of the atrane compounds is that the transannular M–C bond lengths are 0.19–0.32 Å longer than the sum of the respective covalent radii, which is consistent with a zwitterionic component for the description of the molecules. Finally, a particularly noteworthy feature of both [TismPriBenz]Cu and [κ4-C4-TismPriBenz*]Cu is that Cu–Catrane interaction is characterized by an “inverted ligand field”, in which the occupied antibonding orbital is more localized on carbon than on copper.
Acknowledgements
We thank the National Science Foundation (CHE-1058987 and CHE-1465095) for support of this research. M. R. acknowledges the National Science Foundation for a Graduate Research Fellowship under Grant No. DGE-16-44869.
Notes and references
-
(a) J. G. Verkade, Coord. Chem. Rev., 1994, 137, 233–295 CrossRef CAS;
(b) J. G. Verkade, Acc. Chem. Res., 1993, 26, 483–489 CrossRef CAS.
-
(a) M. G. Voronkov, Pure Appl. Chem., 1966, 13, 35–59 CrossRef CAS;
(b) M. G. Voronkov and V. P. Baryshok, J. Organomet. Chem., 1982, 239, 199–249 CrossRef CAS;
(c) M. G. Woronkow, G. I. Seltschan, A. Lapsina and W. A. Pestunowitschich, Z. Chem., 1968, 8, 214–217 CrossRef.
- Although the term “atrane” was originally introduced to describe compounds in which the transannular interaction involved a nitrogen atom, the classification has been expanded to include other atoms. See, for example: N. Chakrabarti, W. Sattler and G. Parkin, Polyhedron, 2013, 58, 235–246 CrossRef CAS and references therein.
- I. Kuzu, I. Krummenacher, J. Meyer, F. Armbruster and F. Breher, Dalton Trans., 2008, 5836–5865 RSC.
-
(a) A. G. Blackman, Polyhedron, 2005, 24, 1–39 CrossRef CAS;
(b) A. G. Blackman, Eur. J. Inorg. Chem., 2008, 2633–2647 CrossRef CAS.
-
(a) H. Kameo and H. Nakazawa, Chem.–Asian J., 2013, 8, 1720–1734 CrossRef CAS PubMed;
(b) H. Braunschweig and R. D. Dewhurst, Dalton Trans., 2011, 40, 549–558 RSC;
(c) H. Braunschweig, R. D. Dewhurst and A. Schneider, Chem. Rev., 2010, 110, 3924–3957 CrossRef CAS PubMed;
(d) F. G. Fontaine, J. Boudreau and M. H. Thibault, Eur. J. Inorg. Chem., 2008, 5439–5454 CrossRef CAS;
(e) A. Amgoune and D. Bourissou, Chem. Commun., 2011, 47, 859–871 RSC;
(f) G. Parkin, Organometallics, 2006, 25, 4744–4747 CrossRef CAS;
(g) G. Bouhadir, A. Amgoune and D. Bourissou, Adv. Organomet. Chem., 2010, 58, 1–107 CrossRef CAS.
- For the classification of ligands as L, X or Z, see:
(a) M. L. H. Green, J. Organomet. Chem., 1995, 500, 127–148 CrossRef CAS;
(b)
G. Parkin, Comprehensive Organometallic Chemistry III, ed. R. H. Crabtree and D. M. P. Mingos, Elsevier, Oxford, 2006, ch. 1, vol. 1 Search PubMed;
(c) M. L. H. Green and G. Parkin, J. Chem. Educ., 2014, 91, 807–816 CrossRef CAS.
- While Fig. 1 depicts three equatorial L-type donors, variants with X-type donors are also known. See, for example, ref. 1, 2 and
(a) L. H. Gade, Acc. Chem. Res., 2002, 35, 575–582 CrossRef CAS PubMed;
(b) R. R. Schrock, Pure Appl. Chem., 1997, 69, 2197–2203 CrossRef CAS;
(c) R. R. Schrock, Acc. Chem. Res., 1997, 30, 9–16 CrossRef CAS;
(d) H. Kawaguchi and T. Matsuo, J. Organomet. Chem., 2004, 689, 4228–4243 CrossRef CAS;
(e) B. V. Kelly, E. C. Weintrob, D. Buccella, J. M. Tanski and G. Parkin, Inorg. Chem. Commun., 2007, 10, 699–704 CrossRef CAS;
(f) L. H. Tong, Y. L. Wong, H. K. Lee and J. R. Dilworth, Inorg. Chim. Acta, 2012, 383, 91–97 CrossRef CAS;
(g) S. Worl, D. Hellwinkel, H. Pritzkow, M. Hofmann and R. Kramer, Dalton Trans., 2004, 2750–2757 RSC;
(h) J. Kobayashi, K. Goto, T. Kawashima, M. W. Schmidt and S. Nagase, J. Am. Chem. Soc., 2002, 124, 3703–3712 CrossRef CAS PubMed;
(i) S. Banerjee, P. Halder and T. K. Paine, Z. Anorg. Allg. Chem., 2014, 640, 1168–1176 CrossRef CAS;
(j) Y. Nakanishi, Y. Ishida and H. Kawaguchi, Dalton Trans., 2016, 45, 15879–15885 RSC;
(k) F. Akagi, Y. Ishida, T. Matsuo and H. Kawaguchi, Dalton Trans., 2011, 40, 2375–2382 RSC;
(l) F. Akagi, T. Matsuo and H. Kawaguchi, J. Am. Chem. Soc., 2005, 127, 11936–11937 CrossRef CAS PubMed.
-
(a) W. Sattler and G. Parkin, J. Am. Chem. Soc., 2011, 133, 9708–9711 CrossRef CAS PubMed;
(b) W. Sattler and G. Parkin, J. Am. Chem. Soc., 2012, 134, 17462–17465 CrossRef CAS PubMed;
(c) W. Sattler, S. Ruccolo and G. Parkin, J. Am. Chem. Soc., 2013, 135, 18714–18717 CrossRef CAS PubMed;
(d) W. Sattler, S. Ruccolo, M. R. Chaijan, T. N. Allah and G. Parkin, Organometallics, 2015, 34, 4717–4731 CrossRef CAS;
(e) W. Sattler and G. Parkin, Catal. Sci. Technol., 2014, 4, 1578–1584 RSC;
(f) W. Sattler and G. Parkin, Chem. Sci., 2012, 3, 2015–2019 RSC.
- S. Ruccolo, W. Sattler, Y. Rong and G. Parkin, J. Am. Chem. Soc., 2016, 138, 14542–14545 CrossRef CAS PubMed.
- For other examples of metallacarbatranes, see ref. 8i–l and
(a) Y. Rong and G. Parkin, Aust. J. Chem., 2013, 66, 1306–1310 CAS;
(b) A. Al-Harbi, Y. Rong and G. Parkin, Dalton Trans., 2013, 42, 14053–14057 RSC;
(c) I. Kinoshita, L. J. Wright, S. Kubo, K. Kimura, A. Sakata, T. Yano, R. Miyamoto, T. Nishioka and K. Isobe, Dalton Trans., 2003, 1993–2003 RSC;
(d) R. Miyamoto, R. Santo, T. Matsushita, T. Nishioka, A. Ichimura, Y. Teki and I. Kinoshita, Dalton Trans., 2005, 3179–3186 RSC;
(e) R. Miyamoto, R. T. Hamazawa, M. Hirotsu, T. Nishioka, I. Kinoshita and L. J. Wright, Chem. Commun., 2005, 4047–4049 RSC;
(f) R. Santo, R. Miyamoto, R. Tanaka, T. Nishioka, K. Sato, K. Toyota, M. Obata, S. Yano, I. Kinoshita, A. Ichimura and T. Takui, Angew. Chem., Int. Ed., 2006, 45, 7611–7614 CrossRef CAS PubMed;
(g) Y. Yoshida, R. Miyamoto, T. Nishioka, H. Hashimoto and I. Kinoshita, Chem. Lett., 2009, 38, 366–367 CrossRef CAS;
(h) I. Kinoshita, H. Hashimoto, T. Nishioka, R. Miyamoto, N. Kuwamura and Y. Yoshida, Photosynth. Res., 2008, 95, 363–371 CrossRef CAS PubMed;
(i) Y. Yoshida, R. Miyamoto, A. Nakato, R. Santo, N. Kuwamura, K. Gobo, T. Nishioka, M. Hirotsu, A. Ichimura, H. Hashimoto and I. Kinoshita, Bull. Chem. Soc. Jpn., 2011, 84, 600–611 CrossRef CAS;
(j) K. Kitano, N. Kuwamura, R. Tanaka, R. Santo, T. Nishioka, A. Ichimura and I. Kinoshita, Chem. Commun., 2008, 1314–1316 RSC;
(k) F. T. Vieira, G. M. de Lima, J. L. Wardell, S. M. S. V. Wardell, K. Krambrock and A. F. d. C. Alcântara, J. Organomet. Chem., 2008, 693, 1986–1990 CrossRef CAS;
(l) N. Kuwamura, R. Kato, K. Kitano, M. Hirotsu, T. Nishioka, H. Hashimoto and I. Kinoshita, Dalton Trans., 2010, 39, 9988–9993 RSC;
(m) S. E. Creutz and J. C. Peters, J. Am. Chem. Soc., 2014, 136, 1105–1115 CrossRef CAS PubMed;
(n) J. Rittle and J. C. Peters, Proc. Natl. Acad. Sci. U. S. A., 2013, 110, 15898–15903 CrossRef CAS PubMed;
(o) P. Halder, S. Paria and T. K. Paine, Chem.–Eur. J., 2012, 18, 11778–11787 CrossRef CAS PubMed;
(p) Y. Yuki, M. Riichi, N. Takanori, H. Hideki and K. Isamu, Chem. Lett., 2009, 38, 366–367 CrossRef.
- For example, the C–Si bond in CH3SiH3 (89.6 kcal mol−1) is stronger than the C–S bond in CH3SH (75.0 kcal mol−1). See: Y.-R. Luo, in Comprehensive Handbook of Chemical Bond Energies, CRC Press, 2007, ch. 8 and 9 Search PubMed.
-
(a) A. Streitwieser, L. Xie, P. Wang and S. M. Bachrach, J. Org. Chem., 1993, 58, 1778–1784 CrossRef CAS;
(b) S. Durmaz, J. Organomet. Chem., 1975, 96, 331–334 CrossRef CAS;
(c) B. Romer, G. G. Gatev, M. L. Zhong and J. I. Brauman, J. Am. Chem. Soc., 1998, 120, 2919–2924 CrossRef;
(d) E. A. Brinkman, S. Berger and J. I. Brauman, J. Am. Chem. Soc., 1994, 116, 8304–8310 CrossRef CAS;
(e) D. M. Wetzel and J. I. Brauman, J. Am. Chem. Soc., 1988, 110, 8333–8336 CrossRef CAS;
(f) P. v. R. Schleyer, T. Clark, A. J. Kos, G. W. Spitznagel, C. Rohde, D. Arad, K. N. Houk and N. G. Rondan, J. Am. Chem. Soc., 1984, 106, 6467–6475 CrossRef CAS.
- See, for example, ref. 8a.
-
(a) D. M. Friesen, R. McDonald and L. Rosenberg, Can. J. Chem., 1999, 77, 1931–1940 CrossRef CAS;
(b) A. Avent, D. Bonafoux, C. Eaborn, S. K. Gupta, P. B. Hitchcock and J. D. Smith, J. Chem. Soc., Dalton Trans., 1999, 831–834 RSC;
(c) D. M. Friesen, O. J. Bowles, R. McDonald and L. Rosenberg, Dalton Trans., 2006, 2671–2682 RSC.
-
Ref. 11n and A. G. Avent, D. Bonafoux, C. Eaborn, M. S. Hill, P. B. Hitchcock and J. D. Smith, J. Chem. Soc., Dalton Trans., 2000, 2183–2190 RSC.
- U. Herzog, G. Rheinwald and H. Borrmann, J. Organomet. Chem., 2002, 660, 27–35 CrossRef CAS.
-
(a) H. Li, A. J. A. Aquino, D. B. Cordes, F. Hung-Low, W. L. Hase and C. Krempner, J. Am. Chem. Soc., 2013, 135, 16066–16069 CrossRef CAS PubMed;
(b) H. Li, A. J. A. Aquino, D. B. Cordes, W. L. Hase and C. Krempner, Chem. Sci., 2017, 8, 1316–1328 RSC.
- Unless otherwise specified, the abbreviation [TismPriBenz] refers to coordination to a metal via a nominal κ4-N3C manner.
- For related synthetic approaches, see ref. 16 and
(a) J. Ohshita, Y. Hatanaka, S. Matsui, T. Mizumo, Y. Kunugi, Y. Honsho, A. Saeki, S. Seki, J. Tibbelin, H. Ottosson and T. Takeuchi, Dalton Trans., 2010, 39, 9314–9320 RSC;
(b) C. L. Lund, O. Stanga, J. W. Quail and J. Muller, Can. J. Chem., 2007, 85, 483–490 CrossRef CAS;
(c) P. Jutzi and W. Sakriss, Chem. Ber., 1973, 106, 2815–2824 CrossRef CAS.
- To the extent that M–Catrane distances are larger than the sum of the covalent radii, the compounds may be better described as quasi-atranes (see ref. 1).
- Note that “trigonal pyramidal” is not synonymous with “trigonal monopyramidal” since the former refers to three-coordinate geometries, while the latter refers to four-coordinate geometries. See, for example, ref. 3 and:
(a) P. A. W. Dean, J. J. Vittal and N. C. Payne, Inorg. Chem., 1984, 23, 4232–4236 CrossRef CAS;
(b) K. Izod, J. Stewart, E. R. Clark, W. McFarlane, B. Allen, W. Clegg and R. W. Harrington, Organometallics, 2009, 28, 3327–3337 CrossRef CAS;
(c) S. Yamana, J. Chem. Educ., 1988, 65, 1074–1074 CrossRef CAS;
(d) C. C. Cummins, J. Lee, R. R. Schrock and W. D. Davis, Angew. Chem., Int. Ed. Engl., 1992, 31, 1501–1503 CrossRef;
(e) M. Ray, B. S. Hammes, G. P. A. Yap, A. L. Rheingold and A. S. Borovik, Inorg. Chem., 1998, 37, 1527–1532 CrossRef CAS;
(f) C. E. Searls, S. T. Kleespies, M. L. Eppright, S. C. Schwartz, G. P. A. Yap and R. C. Scarrow, Inorg. Chem., 2010, 49, 11261–11263 CrossRef CAS PubMed;
(g) D. S. Kuiper, R. E. Douthwaite, A. R. Mayol, P. T. Wolczanski, E. B. Lobkovsky, T. R. Cundari, O. P. Lam and K. Meyer, Inorg. Chem., 2008, 47, 7139–7153 CrossRef CAS PubMed;
(h) S. Suzuki, T. Sakurai, A. Nakahara, M. Masuko and H. Iwasaki, Biochim. Biophys. Acta, 1985, 827, 190–192 CrossRef CAS;
(i) H. Schumann, U. Hartmann, A. Dietrich and J. Pickardt, Angew. Chem., Int. Ed. Engl., 1988, 27, 1077–1078 CrossRef.
- Correspondingly, the four-coordinate τ4 (0.87) and τ4δ (0.87) geometry indices are close to the idealized value for a trigonal monopyramid (0.85). τ4 = [360 − (α + β)]/141, where α and β are the two largest angles, and τ4δ = τ4(β/α), where α > β. See:
(a) L. Yang, D. R. Powell and R. P. Houser, Dalton Trans., 2007, 955–964 RSC;
(b) M. H. Reineke, M. D. Sampson, A. L. Rheingold and C. P. Kubiak, Inorg. Chem., 2015, 54, 3211–3217 CrossRef CAS PubMed.
- Note that the lithium is displaced by 0.24 Å from the trigonal plane of the three nitrogen atoms towards the carbon.
- [Tptm]Li is characterized respectively by (i) N–Li–N and C–Li–N bond angles of 119.86(2)° and 92.21(1)° and (ii) Li–C and Li–N bond lengths of 2.210(5) Å and 2.002(1) Å.
- For reference, the average value of the sum of the Si–C–Si angles in molecules with the C(SiMe2)3 moiety listed in the Cambridge Structural Database is 333.9°. Searches of the Cambridge Structural Database were performed with version 5.37. See: C. R. Groom, I. J. Bruno, M. P. Lightfoot and S. C. Ward, Acta Crystallogr., 2016, B72, 171–179 Search PubMed.
- It is also worth noting that [CSi3] moiety of the related phosphine derivative, [κ4-C(SiMe2CH2PPh2)3]Li, with Li–C–Si angles in the range 103.4–104.9°, and ∑(Si–C–Si) = 343.2°, is much less planar than that of [TismPriBenz]Li. See ref. 16.
- In contrast to [TismPriBenz]Li, the protonated derivative, [TismPriBenz]H, possesses H–C–Si [102.2(15)°, 102.6(15)°, and 108.0(15)°] angles and Si–C–Si [112.32(9)°, 114.34(9)°, and 115.60(9)°] angles which are in accord with a pyramidal geometry for the [CSi3] moiety.
- α-Silyl groups are also known to stabilize carbanions via negative hyperconjugation involving delocalization of electron density from the carbon p-orbital into low energy σ* Si–C bonds. See, for example, ref. 13b–f.
- A. G. Avent, D. Bonafoux, C. Eaborn, S. K. Gupta, P. B. Hitchcock and J. D. Smith, J. Chem. Soc., Dalton Trans., 1999, 831–834 RSC.
-
(a) C. Eaborn, K. Izod and J. D. Smith, J. Organomet. Chem., 1995, 500, 89–99 CrossRef CAS;
(b) S. S. Al-Juaid, C. Eaborn, P. B. Hitchcock, K. Izod, M. Mallien and J. D. Smith, Angew. Chem., Int. Ed. Engl., 1994, 33, 1268–1270 CrossRef;
(c) C. Eaborn, P. B. Hitchcock, K. Izod, A. J. Jaggar and J. D. Smith, Organometallics, 1994, 13, 753–754 CrossRef CAS.
- For examples of formally zwitterionic compounds with a planar [C(SiMe2X)3] moiety, see ref. 18b and:
(a) C. Eaborn, A. Farook, P. B. Hitchcock and J. D. Smith, Organometallics, 1998, 17, 3135–3137 CrossRef CAS;
(b) F. Adam, C. Eaborn, P. B. Hitchcock and J. D. Smith, Chem. Commun., 1996, 741–742 RSC;
(c) C. Eaborn, A. Farook, P. B. Hitchcock and J. D. Smith, Organometallics, 1997, 16, 503–504 CrossRef CAS.
- R. Chauvin, Eur. J. Inorg. Chem., 2000, 577–591 CrossRef CAS.
- For other carbatranes with formally zwitterionic character, see ref. 10.
- For examples of metal compounds with formally three-coordinate anionic carbon centers in which the lone pair on carbon is directed away from the metal center, see:
(a) S. O. Grim, P. H. Smith, S. Nittolo, H. L. Ammon, L. C. Satek, S. A. Sangokoya, R. K. Khanna, I. J. Colquhoun, W. McFarlane and J. R. Holden, Inorg. Chem., 1985, 24, 2889–2895 CrossRef CAS;
(b) S. O. Grim, S. A. Sangokoya, A. L. Rheingold, W. McFarlane, I. J. Colquhoun and R. D. Gilardi, Inorg. Chem., 1991, 30, 2519–2522 CrossRef CAS;
(c) M. Valderrama, R. Contreras, V. Arancibia, P. Muñoz, D. Boys, M. P. Lamata, F. Viguri, D. Carmona, F. J. Lahoz, J. A. López and L. A. Oro, J. Organomet. Chem., 1997, 545, 507–517 CrossRef;
(d) M. C. Gimeno, P. G. Jones, A. Laguna and M. D. Villacampa, Chem. Ber., 1996, 129, 585–588 CrossRef CAS;
(e) F. Breher, J. Grunenberg, S. C. Lawrence, P. Mountford and H. Rüegger, Angew. Chem., Int. Ed., 2004, 43, 2521–2524 CrossRef CAS PubMed;
(f) R. Lalrempuia, A. Stasch and C. Jones, Chem.–Asian J., 2015, 10, 447–454 CrossRef CAS PubMed;
(g) C. Müller, A. Koch, H. Görls, S. Krieck and M. Westerhausen, Inorg. Chem., 2015, 54, 635–645 CrossRef PubMed;
(h) I. Kuzu, I. Krummenacher, I. J. Hewitt, Y. Lan, V. Mereacre, A. K. Powell, P. Höfer, J. Harmer and F. Breher, Chem.–Eur. J., 2009, 15, 4350–4365 CrossRef CAS PubMed;
(i) S. González-Gallardo, I. Kuzu, P. Oña-Burgos, T. Wolfer, C. Wang, K. W. Klinkhammer, W. Klopper, S. Bräåse and F. Breher, Organometallics, 2014, 33, 941–951 CrossRef;
(j) J. Meyer, I. Kuzu, S. González-Gallardo and F. Breher, Z. Anorg. Allg. Chem., 2013, 639, 301–307 CrossRef CAS;
(k) D. Kratzert, D. Leusser, D. Stern, J. Meyer, F. Breher and D. Stalke, Chem. Commun., 2011, 47, 2931–2933 RSC.
- B. Cordero, V. Gómez, A. E. Platero-Prats, M. Revés, J. Echeverría, E. Cremades, F. Barragán and S. Alvarez, Dalton Trans., 2008, 2832–2838 RSC.
- For examples of lithium alkyl compounds with Li–C bond lengths comparable to the sum of the covalent radii, see:
(a) M. Hülsmann, A. Mix, B. Neumann, H.-G. Stammler and N. W. Mitzel, Eur. J. Inorg. Chem., 2014, 2014, 46–50 CrossRef;
(b) C. Strohmann, V. H. Gessner and A. Damme, Chem. Commun., 2008, 3381–3383 RSC.
- For examples of magnesium alkyl compounds with Mg–C bond lengths comparable to the sum of the covalent radii, see:
(a) P. J. Bailey, D. Lorono-Gonzalez and S. Parsons, Chem. Commun., 2003, 1426–1427 RSC;
(b) V. Balasanthiran, M. H. Chisholm, K. Choojun, C. B. Durr and P. M. Wambua, Polyhedron, 2016, 103, 235–240 CrossRef CAS;
(c) R. Han, A. Looney and G. Parkin, J. Am. Chem. Soc., 1989, 111, 7276–7278 CrossRef CAS;
(d) R. Han and G. Parkin, Organometallics, 1991, 10, 1010–1020 CrossRef CAS.
- In contrast, the Mg–CH3 [2.1781(13) Å] and Mg–N [2.1890(11) Å, 2.2156(11) Å, 2.2238(11) Å] bonds correspond closely to the respective sums of covalent radii (2.17 Å and 2.12 Å).
- The HOMO is highly localized on the carbon atom of the Mg–CH3 moiety.
- E. C. Constable, Prog. Inorg. Chem., 1994, 42, 67–138 CrossRef CAS.
- Despite this different coordination mode, the sum of the three Si–C–Si angles of [κ3-TismPriBenz]ZnMe (346.30°) is similar to that for [TismPriBenz]MgMe (347.84°).
- The compound is, however, fluxional in solution, such that the imidazolyl groups are rendered chemically equivalent at room temperature. Decoalescence occurs upon lowering the temperature, such that broad signals indicative of a κ3 coordination mode are observed at −70 °C.
- The κ3-coordination mode is also observed for tris(2-pyridylthio)methyl and tris(1-methylimidazol-2-ylthio)methyl compounds, [κ3-Tptm]ZnMe9a and [κ3-TitmMe]ZnMe.10.
- For examples of zinc alkyl compounds with Zn–C bond lengths comparable to the sum of the covalent radii, see:
(a) C. Romain, V. Rosa, C. Fliedel, F. Bier, F. Hild, R. Welter, S. Dagorne and T. Aviles, Dalton Trans., 2012, 41, 3377–3379 RSC;
(b) A. Stasch, Chem.–Eur. J., 2012, 18, 15105–15112 CrossRef CAS PubMed;
(c) J. G. Melnick, A. Docrat and G. Parkin, Chem. Commun., 2004, 2870–2871 RSC;
(d) I. B. Gorrell, A. Looney and G. Parkin, J. Chem. Soc., Chem. Commun., 1990, 220–222 RSC;
(e) A. Looney, R. Han, I. B. Gorrell, M. Cornebise, K. Yoon, G. Parkin and A. L. Rheingold, Organometallics, 1995, 14, 274–288 CrossRef CAS.
- The Zn–N bond lengths [2.088(2) Å and 2.091(2) Å] are also close to the sum of covalent radii (1.93 Å).
- For an example of trigonal monopyramidal Cu(I) compound with three imidazole donors, see: J. K. Voo, K. C. Lam, A. L. Rheingold and C. G. Riordan, J. Chem. Soc., Dalton Trans., 2001, 1803–1805 RSC.
- For an example of a structurally related anionic boratrane derivative, see: M. E. Moret, L. M. Zhang and J. C. Peters, J. Am. Chem. Soc., 2013, 135, 3792–3795 CrossRef CAS PubMed.
- For examples of copper alkyl compounds with Cu–C bond lengths comparable to the sum of the covalent radii, see:
(a) F. Schaper, S. R. Foley and R. F. Jordan, J. Am. Chem. Soc., 2004, 126, 2114–2124 CrossRef CAS PubMed;
(b) G. Boche, F. Bosold, M. Marsch and K. Harms, Angew. Chem., Int. Ed., 1998, 37, 1684–1686 CrossRef CAS.
- It is also worth noting that copper carbatrane compounds of the type [Tptm]CuX possess Cu–C bond lengths that are comparable to the sum of the covalent radii. See, for example, ref. 11c–h.
- R. Hoffmann, S. Alvarez, C. Mealli, A. Falceto, T. J. Cahill, T. Zeng and G. Manca, Chem. Rev., 2016, 116, 8173–8192 CrossRef CAS PubMed.
- In contrast, the opposite situation is observed for Lewis acidic ligands in which the unoccupied acceptor orbitals are higher in energy than the occupied metal orbitals. See, for example, ref. 4 and 6.
- R. C. Walroth, J. T. Lukens, S. N. MacMillan, K. D. Finkelstein and K. M. Lancaster, J. Am. Chem. Soc., 2016, 138, 1922–1931 CrossRef CAS PubMed.
-
(a) J. P. Snyder, Angew. Chem., Int. Ed. Engl., 1995, 34, 80–81 CrossRef CAS;
(b) M. Kaupp and H. G. Vonschnering, Angew. Chem., Int. Ed. Engl., 1995, 34, 986–986 CrossRef CAS;
(c) S. Alvarez, R. Hoffmann and C. Mealli, Chem.–Eur. J., 2009, 15, 8358–8373 CrossRef CAS PubMed;
(d) G. Aullón and S. Alvarez, Theor. Chem. Acc., 2009, 123, 67–73 CrossRef.
- It is worth noting that inverted ligand field patterns can also be obtained due to molecular distortions. For example, the axial distortion of a tetrahedron can result in a “two-above-three” pattern of d orbitals, rather than the “three-above-two” pattern that is associated with tetrahedral symmetry. See, for example:
(a) D. M. Jenkins, A. J. Di Bilio, M. J. Allen, T. A. Betley and J. C. Peters, J. Am. Chem. Soc., 2002, 124, 15336–15350 CrossRef CAS PubMed;
(b) D. M. Jenkins and J. C. Peters, J. Am. Chem. Soc., 2005, 127, 7148–7165 CrossRef CAS PubMed;
(c) S. D. Brown and J. C. Peters, J. Am. Chem. Soc., 2005, 127, 1913–1923 CrossRef CAS PubMed.
- For related isomerization reactions of tripodal compounds, see:
(a) P. Halder and T. K. Paine, Inorg. Chem., 2011, 50, 708–710 CrossRef CAS PubMed;
(b) N. Kuwamura, K. Kitano, M. Hirotsu, T. Nishioka, Y. Teki, R. Santo, A. Ichimura, H. Hashimoto, L. J. Wright and I. Kinoshita, Chem.–Eur. J., 2011, 17, 10708–10715 CrossRef CAS PubMed;
(c) C. Gwengo, R. M. Silva, M. D. Smith, S. V. Lindeman and J. R. Gardinier, Inorg. Chim. Acta, 2009, 362, 4127–4136 CrossRef CAS;
(d) D. L. Reger, T. C. Grattan, K. J. Brown, C. A. Little, J. J. S. Lamba, A. L. Rheingold and R. D. Sommer, J. Organomet. Chem., 2000, 607, 120–128 CrossRef CAS;
(e) A. Al-Harbi, Y. Rong and G. Parkin, Dalton Trans., 2013, 42, 14053–14057 RSC;
(f) J. M. White, V. W. L. Ng, D. C. Clarke, P. D. Smith, M. K. Taylor and C. G. Young, Inorg. Chim. Acta, 2009, 362, 4570–4577 CrossRef CAS and references therein.
-
(a) C. Santini, M. Marinelli and M. Pellei, Eur. J. Inorg. Chem., 2016, 2016, 2312–2331 CrossRef CAS;
(b) X. Hu and K. Meyer, J. Organomet. Chem., 2005, 690, 5474–5484 CrossRef CAS;
(c) K. Meyer and S. C. Bart, Adv. Inorg. Chem., 2008, 60, 1–30 CrossRef CAS;
(d) J. Smith, Comments Inorg. Chem., 2008, 29, 189–233 CrossRef CAS;
(e) J. A. Mata, M. Poyatos and E. Peris, Coord. Chem. Rev., 2007, 251, 841–859 CrossRef CAS.
-
(a) H. V. R. Dias and W. C. Jin, Tetrahedron Lett., 1994, 35, 1365–1366 CrossRef CAS;
(b) H. Nakai, Y. J. Tang, P. Gantzel and K. Meyer, Chem. Commun., 2003, 24–25 RSC;
(c) X. Hu, Y. Tang, P. Gantzel and K. Meyer, Organometallics, 2003, 22, 612–614 CrossRef CAS;
(d) H. Kropp, A. Scheurer, F. W. Heinemann, J. Bendix and K. Meyer, Inorg. Chem., 2015, 54, 3562–3572 CrossRef CAS PubMed;
(e) S. B. Muñoz, W. K. Foster, H.-J. Lin, C. G. Margarit, D. A. Dickie and J. M. Smith, Inorg. Chem., 2012, 51, 12660–12668 CrossRef PubMed.
- For some reviews pertaining to the coordination chemistry of N-heterocyclic carbenes, see:
(a) D. Bourissou, O. Guerret, F. P. Gabbai and G. Bertrand, Chem. Rev., 2000, 100, 39–91 CrossRef CAS PubMed;
(b) D. J. Nelson and S. P. Nolan, Chem. Soc. Rev., 2013, 42, 6723–6753 RSC.
- For an example of a tripodal tris(N-heterocyclic carbene) coordinated to Cu(I), see: X. Hu, I. Castro-Rodriguez and K. Meyer, J. Am. Chem. Soc., 2003, 125, 12237–12245 CrossRef CAS PubMed.
- This variation is also reproduced by DFT calculations, although the magnitude of the increase (0.32 Å) is greater than that observed experimentally (0.15 Å).
- For examples of N-heterocyclic carbene complexes of the main group metals, see:
(a) S. Bellemin-Laponnaz and S. Dagorne, Chem. Rev., 2014, 114, 8747–8774 CrossRef CAS PubMed;
(b) C. E. Williams, Organomet. Chem., 2010, 36, 1–28 Search PubMed.
- In addition, energy changes associated with the different Cu⋯Catrane interactions also play a role in dictating the relative stabilities of the two isomers.
- For a discussion of the conformational classifications of tripodal ligands, see: A. Kreider-Mueller, Y. Rong, J. S. Owen and G. Parkin, Dalton Trans., 2014, 43, 10852–10865 RSC.
- A similar difference in energy (40.9 kcal mol−1) is also calculated for [TismPriBenz*]H and [TismPriBenz]H with a 1
:
2 conformation akin to that of the solid state structure of the latter. In addition, the [TismPriBenz*]− anion is 25.6 kcal mol−1 higher in energy than the tris(imidazole) counterpart, [TismPriBenz]−, with the 3
:
0 conformation that is used for κ4-coordination.
- Indeed, calculations on the parent imidazole demonstrate that the tautomer with one NH moiety is more stable than the tautomer with two NH groups. See:
(a) G. Sini, O. Eisenstein and R. H. Crabtree, Inorg. Chem., 2002, 41, 602–604 CrossRef CAS PubMed;
(b) R. Tonner, G. Heydenrych and G. Frenking, Chem.–Asian J., 2007, 2, 1555–1567 CrossRef CAS PubMed;
(c) G. A. McGibbon, C. Heinemann, D. J. Lavorato and H. Schwarz, Angew. Chem., Int. Ed. Engl., 1997, 36, 1478–1481 CrossRef CAS;
(d) C. Heinemann and W. Thiel, Chem. Phys. Lett., 1994, 217, 11–16 CrossRef CAS;
(e) H. Basch, M. Krauss and W. J. Stevens, Int. J. Quantum Chem., 1987, 31, 405–415 CrossRef CAS.
- In this regard, it is pertinent to note that, whereas the geometry optimized Cu–CHet bond length (2.005 Å) of [κ4-C4-TismPriBenz*]Cu is shorter than the Cu–N bond lengths (2.073 Å) of [TismPriBenz]Cu, the opposite is observed for the lithium system, with the Li–CHet distance (2.103 Å) being longer than the Li–N interaction (2.024 Å).
-
(a) R. J. Sundberg, R. F. Bryan, I. F. Taylor and H. Taube, J. Am. Chem. Soc., 1974, 96, 381–392 CrossRef CAS;
(b) M. Brill, J. Diaz, M. A. Huertos, R. Lopez, J. Perez and L. Riera, Chem.–Eur. J., 2011, 17, 8584–8595 CrossRef CAS PubMed;
(c) B. Eguillor, M. A. Esteruelas, J. Garcia-Raboso, M. Olivan, E. Onate, I. M. Pastor, I. Penafiel and M. Yus, Organometallics, 2011, 30, 1658–1667 CrossRef CAS;
(d) M. H. Yu, H. H. Yang, A. R. Naziruddin, S. Kanne, B. H. Wang, F. C. Liu, I. J. B. Lin and G. H. Lee, Eur. J. Inorg. Chem., 2016, 4829–4834 CrossRef CAS;
(e) M. A. Huertos, J. Perez, L. Riera, J. Diaz and R. Lopez, Chem.–Eur. J., 2010, 16, 8495–8507 CrossRef CAS PubMed;
(f) M. A. Huertos, J. Perez, L. Riera and A. Menedez-Veldazquez, J. Am. Chem. Soc., 2008, 130, 13530–13531 CrossRef CAS PubMed;
(g) M. A. Huertos, J. Perez, L. Riera, J. Diaz and R. Lopez, Angew. Chem., Int. Ed., 2010, 49, 6409–6412 CrossRef CAS PubMed;
(h) J. Ruiz and B. F. Perandones, J. Am. Chem. Soc., 2007, 129, 9298–9299 CrossRef CAS PubMed.
- Examples of the transformation of a C-coordinated ligand to a N-coordinated imidazole ligand have also been observed. See:
(a) S. Burling, M. F. Mahon, R. E. Powell, M. K. Whittlesey and J. M. J. Williams, J. Am. Chem. Soc., 2006, 128, 13702–13703 CrossRef CAS PubMed;
(b) L. J. L. Haller and S. A. Macgregor, Eur. J. Inorg. Chem., 2009, 2000–2006 CrossRef.
- The isomerization of 2-substituted pyridines to afford N-heterocyclic carbene derivatives is also known. See, for example:
(a) S. Conejero, J. Lopez-Serrano, M. Paneque, A. Petronilho, M. L. Poveda, F. Vattier, E. Alvarez and E. Carmona, Chem.–Eur. J., 2012, 18, 4644–4664 CrossRef CAS PubMed;
(b) F. Vattier, V. Salazar, M. Paneque, M. L. Poveda and E. Alvarez, Organometallics, 2014, 33, 498–510 CrossRef CAS.
- For the formal 1,2-migration of silyl groups from nitrogen to carbon in N-heterocyclic carbenes, see: S. Sole, H. Gornitzka, O. Guerret and G. Bertrand, J. Am. Chem. Soc., 1998, 120, 9100–9101 CrossRef CAS.
- These data are for crystals of [TismPriBenz]NiBr·THF obtained from a solution in THF. A different crystalline form, [TismPriBenz]NiBr·C6H6, with similar bond lengths and angles, has also been obtained.
- Note that the idealized trigonal bipyramidal structure of [TismPriBenz]NiBr is one in which the Br is in an equatorial site, which contrasts with the structure of [TismPriBenz]MgMe in which the methyl group is in an axial site.
-
τ
5 = (β − α)/60, where β − α is the difference between the two largest angles. Idealized trigonal bipyramidal and square pyramidal geometries are characterized by values of 1.00 and 0.00, respectively. See: A. W. Addison, T. N. Rao, J. Reedijk, J. van Rijn and G. C. Verschoor, J. Chem. Soc., Dalton Trans., 1984, 1349–1356 RSC.
- Distortions of this type are not uncommon for 5-coordinate d8 nickel centers. See, for example:
(a) C. Janiak, L. Uehlin, H. P. Wu, P. Klufers, H. Piotrowski and T. G. Scharmann, J. Chem. Soc., Dalton Trans., 1999, 3121–3131 RSC;
(b) M. D. Santana, G. Garcia, A. A. Lozano, G. Lopez, J. Tudela, J. Perez, L. Garcia, L. Lezama and T. Roj, Chem.–Eur. J., 2004, 10, 1738–1746 CrossRef CAS PubMed;
(c) S. Zai, H. Gao, Z. Huang, H. Hu, H. Wu and Q. Wu, ACS Catal., 2012, 2, 433–440 CrossRef CAS;
(d) P. Chavez, I. G. Rios, A. Kermagoret, R. Pattacini, A. Meli, C. Bianchini, G. Giambastiani and P. Braunstein, Organometallics, 2009, 28, 1776–1784 CrossRef CAS;
(e) F. Speiser, P. Braunstein, L. Saussine and R. Welter, Organometallics, 2004, 23, 2613–2624 CrossRef CAS;
(f) R. Gao, M. Zhang, T. L. Liang, F. S. Wang and W. H. Sun, Organometallics, 2008, 27, 5641–5648 CrossRef CAS;
(g) X. Hou, T. L. Liang, W.-H. Sun, C. Redshaw and X. Chen, J. Organomet. Chem., 2012, 708, 98–105 CrossRef;
(h) J. Hou, W.-H. Sun, S. Zhang, H. Ma, Y. Deng and X. Lu, Organometallics, 2006, 25, 236–244 CrossRef CAS.
- For a review of the structures of 5-coordinate nickel(II) compounds, see: D. M. Roddick and D. Zargarian, Inorg. Chim. Acta, 2014, 422, 251–264 CrossRef CAS.
- For examples of nickel alkyl compounds with Ni–C bond lengths comparable to the sum of the covalent radii, see:
(a) J. Cámpora, I. Matas, P. Palma, C. Graiff and A. Tiripicchio, Organometallics, 2005, 24, 2827–2830 CrossRef;
(b) C. Yoo, S. Oh, J. Kim and Y. Lee, Chem. Sci., 2014, 5, 3853–3858 RSC.
Footnote |
† Electronic supplementary information (ESI) available: Experimental details. CCDC 1529724–1529731. For ESI and crystallographic data in CIF or other electronic format see DOI: 10.1039/c7sc00499k |
|
This journal is © The Royal Society of Chemistry 2017 |
Click here to see how this site uses Cookies. View our privacy policy here.