DOI:
10.1039/C7SC03903D
(Edge Article)
Chem. Sci., 2017,
8, 8285-8290
Bioinspired synthesis of pentacyclic onocerane triterpenoids†
Received
6th September 2017
, Accepted 14th October 2017
First published on 16th October 2017
Abstract
The first chemical synthesis of pentacyclic onocerane triterpenoids has been achieved. A putative biomimetic tricyclization cascade is employed to forge a fused decalin-/oxepane ring system. The synthetic route proceeds to (+)-cupacinoxepin in seven steps and to (+)-onoceranoxide in eight steps in the longest linear sequence, when starting from geranyl chloride and (+)-sclareolide. The bioinspired epoxypolyene cyclization is supported by computational and enzymatic studies.
Triterpenoids constitute an important family of diverse natural products with unique biological activities.1 Their structural complexity is generated by cyclase enzymes that convert simple acyclic isoprenoid precursors into polycyclic molecules.2,3 For example, onocerane triterpenes were shown to be biosynthesized from squalene (1) or its oxidized derivatives (2, 3) by cyclizations initiated at both termini.4 The intermediates and products can be distinguished by their oxidation level (OL), (carbo-)cyclization level (CL), and the hydration level (HL), i.e. the number of incorporated water molecules. Following core assembly, functional group modifications (FGMs) or C–H-oxidations5 may occur (Scheme 1). In a bioassay-guided screening for anti-malarial compounds, Schuehly and coworkers reported the isolation of a novel triterpenoid from the bark of Cupania cinerea.6 Cupacinoxepin (4) showed moderate activity against the Plasmodium falciparum K1 strain (8.7 μM) and features a novel fused pentacyclic onocerane scaffold composed of three six-membered carbocycles and two oxepanes. Although data from a single crystal suitable for X-ray crystallography was obtained, the absolute configuration could not be determined.6 Previous synthetic work on the onceranes7–10 was focused on the tetracyclic C2-symmetrical congeners onocerandiol (5)11–14 and α-onocerin (6).15–22 The biosynthesis of onoceranoxide (7)23 and α-onocerin (6) via824,25 was hypothesized to proceed via cyclization of squalene and diepoxysqualene, respectively (Scheme 1). This process was elegantly mimicked by Corey's double allylsilane epoxypolyene cyclization.16 However, the highly substituted oxepane in cupacinoxepin (4) and onoceranoxide (7)26,27 required a novel synthetic strategy.
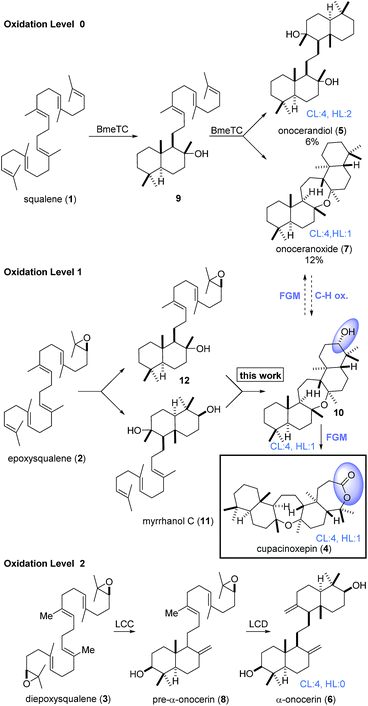 |
| Scheme 1 Core assembly of onocerane triterpenes with regard to the oxidation level (OL), cyclization level (CL) and the hydration level (HL). LCC and LCD = Lycopodium clavatum C and D respectively. | |
Chemical synthesis of ditertiary ethers is a daunting task.28–38 Unfortunately, the seemingly obvious approach to form the oxepane from two tertiary alcohols is outpaced by competing cationic pathways.13,14 Inspired by the cyclization of squalene to 7via9 catalyzed by Bacillus megaterium tetraprenyl-β-curcumene cyclase (BmeTC),23 we identified myrrhanol C (11)39 or epoxy dienol 12 as potential precursors for the synthesis of 10. While the actual biosynthetic pathway is unknown, the realization of an epoxydiene tricyclization appeared more feasible in a laboratory setting. Finally, oxidation of the secondary alcohol 10 to cupacinoxepin (4) (via the intermediacy of a ketone) completes the putative biosynthesis. Only a few examples of polyene cyclizations40–45 using tertiary alcohols as nucleophiles are known,46–48 and to the best of our knowledge polyene tricyclizations using tertiary alcohols to form oxepanes have only been achieved enzymatically so far.23,49 In order to probe this key cyclization (12 → 10) in the laboratory, we selected epoxy dienol 12 as our retrosynthetic target (Scheme 2).
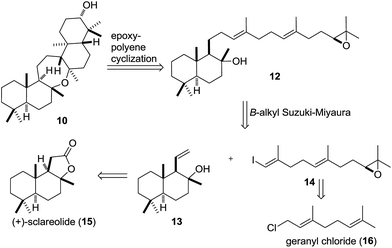 |
| Scheme 2 Retrosynthetic analysis of 10 based on an epoxypolyene cyclization of precursor 12. | |
We envisioned the cyclization precursor 12 to be generated in a B-alkyl Suzuki–Miyaura coupling between an alkylborane derived from 13 and vinyl iodide 14.50 The two fragments were traced back to the readily available starting materials (+)-sclareolide (15) and geranyl chloride (16), respectively.51–53
The synthesis of fragment 1354 started with the conversion of 15 into the corresponding benzyl ether 17 using a one-pot55,56 reduction/alkylation sequence (Scheme 3). To this end, reduction of (+)-sclareolide with LiAlH4 in THF at 0 °C
57 was followed by treatment with Rochelle salt, DMF, KOH and 2-Me-C6H4CH2Br to afford benzyl ether 17 in excellent yield. A [2,3]-Wittig-type fragmentation mediated by n-BuLi directly yielded fragment 13 in an acceptable yield of 44% over two steps.58,59 The subtle deviation of the ether group from the literature-known benzyl ether to its 2-Me-benzyl derivative increased the yield from 21% to 44%. A screening of different ether derivatives showed alkyl substituents with benzylic hydrogen atoms in the 2-position of the aromatic ring to give higher yields of 13. Moreover, we observed a temperature dependence of the fragmentation yield, maximizing at −13 °C.46 The configuration of 13 was confirmed by X-ray crystallography.60
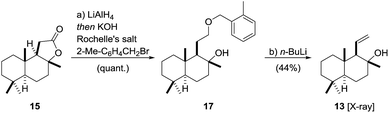 |
| Scheme 3 Synthesis of fragment 13. Reagents and conditions: (a) LiAlH4 (0.7 eq.), THF, 0 °C, 40 min then 2-Me-C6H4CH2Br (2.1 eq.), KOH (4.0 eq.), Rochelle's salt (1.2 eq.), DMF, 45 °C, 27 h, quant.; (b) n-BuLi (4.0 eq.), THF, −78 °C, 10 min to −13 °C, 90 min, 44% (over 2 steps). | |
Vinyl iodide 14 was prepared from geranyl chloride via nucleophilic substitution with lithiated 1861 followed by desilylation with TBAF (Scheme 4). Negishi's zirconium-catalyzed carboalumination62,63 of 19 with AlMe3 and subsequent trapping of the vinyl aluminium intermediate with iodine afforded vinyl iodide 20.64,65 Dihydroxylation of the dimethyl-substituted alkene with the (DHQD)2PHAL ligand66 (33% yield, 97% ee) proceeded with low position-selectivity which is reflected by the small amount of recovered starting material (25%). Using the Corey–Noe–Lin (CNL) ligand67 increased the position-selectivity to give the terminal diol in 36% yield (94% ee) with 49% of recovered alkene.68 Mesylation of the secondary alcohol and subsequent treatment with K2CO3 afforded epoxide 14 in 81% yield.69 With alkene 13 and vinyl iodide 14 in hand, the crucial coupling was investigated (Scheme 5). Treatment of alkene 13 (neat) with 9-BBN dimer at 85 °C for 4 h led to the expected borane, which was directly used in the B-alkyl Suzuki–Miyaura reaction69–71 to afford epoxy dienol 12 in 77% yield on gram scale. The stage was then set to examine the putative biomimetic tricyclization.72 We anticipated the formation of the oxepane to be unfavorable both for entropic and enthalpic reasons.73 A variety of Brønsted and Lewis acids46 failed to give the desired product, but gratifyingly, treatment of 12 with EtAlCl2 at −78 °C under high dilution (CH2Cl2, 1 mM) afforded a separable mixture of target compound 10 (20%) along with another pentacyclic product 21 (12%).74–76 Spirocyclic motifs similar to 21 have been found in several bioactive natural products.77–80 Further conditions for the tricyclization of 9 were investigated but no product formation could be observed.46
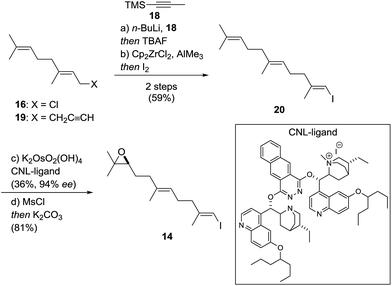 |
| Scheme 4 Synthesis of fragment 14. Reagents and conditions: (a) n-BuLi (1.2 eq.), 18 (1.2 eq.), THF, −78 °C, 2.5 h then TBAF (1.3 eq.), −78 °C to 23 °C, 24 h, 82%; (b) Cp2ZrCl2 (0.25 eq.), AlMe3 (3.0 eq.), H2O (1.0 eq.), CH2Cl2, −23 °C, 1 h then I2 (1.2 eq.), THF, −23 °C to 23 °C, 16 h, 72%; (c) K2OsO2(OH)4 (0.3 mol%), CNL-ligand (0.2 mol%), K3Fe(CN)6 (3.0 eq.), MeSO2NH2 (1.0 eq.), K2CO3 (3.0 eq.), t-BuOH, H2O, 1 °C, 53 h, 36%, 94% ee, and 49% of 20; (d) MsCl (1.1 eq.), pyridine (15 eq.), CH2Cl2, 0 °C to 23 °C, 18 h then K2CO3 (10 eq.), MeOH, 2.5 h, 81%. | |
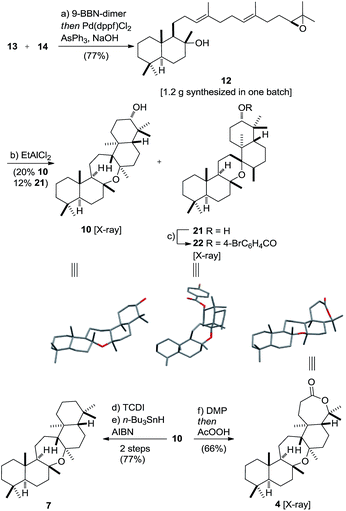 |
| Scheme 5 Synthesis of cupacinoxepin 4 and onoceranoxide 7. Reagents and conditions: (a) 13 (1.4 eq.), 9-BBN dimer (2.8 eq.), 85 °C, 4 h then 14 (1.0 eq.), Pd(dppf)Cl2 (0.1 eq.), AsPh3 (0.4 eq.), NaOH (6.0 eq.), 1 °C, 17 h, 77%; (b) EtAlCl2 (3.0 eq.), CH2Cl2 (1 mM), −78 °C, 1.5 h, 20% 10, 12% 21; (c) 4-BrC6H4COCl (5.0 eq.), 4-DMAP (20 eq.), 50 °C, CH2Cl2, 3 d, 73%; (d) TCDI (20 eq.), 4-DMAP (20 eq.), CH2Cl2, 70 °C 13.5 h, 83%; (e) n-Bu3SnH (3.0 eq.), AIBN (cat.), toluene, 160 °C, 10 min to 120 °C, 20 min then n-Bu3SnH (3.0 eq.), AIBN (cat.), 160 °C, 10 min to 120 °C, 20 min, 93%; (f) DMP (2.0 eq.), CH2Cl2, 23 °C, 4 h then AcOOH (10 eq.), NaOAc (20 eq.), 17 h then AcOOH (10 eq.), NaOAc (20 eq.), 5 h, 66%. DMP = Dess–Martin periodinane, TCDI = 1,1′-thiocarbonyldiimidazole, AIBN = azobisisobutyronitrile. | |
The structures of oxepane 10 and p-Br-benzoate derivative 22 were confirmed by X-ray crystallography. Despite its rather low yield, the cyclization generated four stereogenic centers and the remaining carbon skeleton in a single transformation. Additionally, the secondary alcohol in 10 might serve as a handle in future SAR studies and enable the evaluation of this class of pentacyclic onocerane triterpenoids as potential antimalarial drug leads.81,82 In addition, recent work in the field of C–H functionalization has demonstrated the sclareolide scaffold to be amenable for the selective introduction of other functional groups.83–90 Next, the major cyclization product 10 was subjected to a one-pot Dess–Martin/Baeyer–Villiger oxidation91–94 to afford cupacinoxepin in 66% yield (1.7% overall yield starting from 16). The spectroscopic data, including the optical rotation, matched those reported in the literature, thereby determining the absolute configuration of (+)-cupacinoxepin.6 In addition, we were able to obtain a crystal suitable for the direct determination of the absolute configuration of 4 by X-ray crystallography. Onoceranoxide 7 was formed from 10via formation of the thiocarbamate and subsequent reduction with tributyltin hydride in 77% yield (2 steps) (2.0% overall yield starting from 16).95 The spectroscopic data matched those reported in the literature.26
In order to investigate the mechanism of the epoxypolyene cyclization in more detail and to get insight into the low selectivity for the desired trans–anti–trans pathway, well established density functional theory (DFT) methods were applied (mPW1PW91/6-31+G(d,p)//B3LYP/6-31+G(d)).46 Two epimeric trans-decalin-type structures (S-epimer 23 and R-epimer 24, Scheme 6) were predicted, based on inherent reactivity preferences (i.e., in the absence of solvent or enzyme),96 to result from epoxydiene cyclization, consistent with previous work by Corey and Shenvi on related systems.75,97 The predicted major intermediate 23 is derived from a chair–chair conformation, while 24 is derived from a chair–boat conformation.75,80
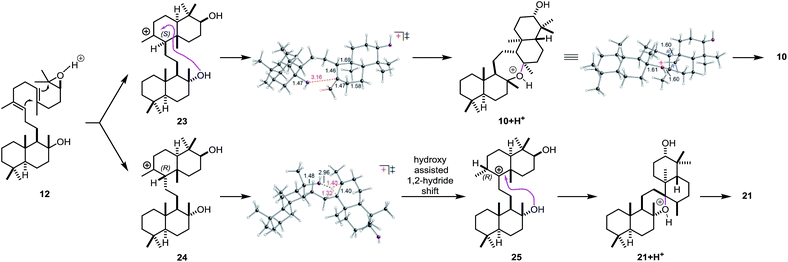 |
| Scheme 6 Carbocation rearrangements leading to 10 and 21, modeled with H+ in place of Lewis acid (LA). | |
For 23, 7-membered ring formation was predicted to be preferred over other pathways by several kcal mol−1. For 24, 1,2-hydride shift to form 25/6-membered ring formation was predicted to be preferred over formation of a 7-membered ring by several kcal mol−1. As shown in the transition state structure for formation of 25, the hydride shift appears to be assisted by the tertiary hydroxyl group (via a favorable electrostatic interaction between the partially negatively charged oxygen and the partially positively charged migrating hydrogen; related interactions have been described previously).98,99 In addition, the biosynthetic relevance of the epoxypolyene cyclization was probed by incubation of 12 with BmeTC. GC-MS analysis revealed the formation of 10 along with an elimination product.46 The absence of 21 indicates the influence of BmeTC in overriding inherent reactivity and enforcing the chair–chair conformation leading to 10.
In conclusion, we have provided access to a new class of pentacyclic onocerane triterpenoids. Additionally, we have completed the first asymmetric synthesis of antiprotozoal agent (+)-cupacinoxepin and (+)-onoceranoxide and determined their absolute configuration. By using an epoxypolyene cascade tricyclization as the key step, we were able to rapidly assemble the fused pentacyclic structure in a single synthetic operation. The putative biosynthetic precursor was assembled from two terpene derived fragments using a B-alkyl Suzuki–Miyaura reaction. Our synthetic route to cupacinoxepin consists of seven steps from geranyl chloride, four of which are C–C bond formations.
Conflicts of interest
There are no conflicts to declare.
Acknowledgements
We thank the Studienstiftung des deutschen Volkes for a doctoral fellowship (F. B.), Buchler GmbH (Braunschweig) for a generous donation of dihydrochinidin-hydrochloride, Joanna Najdek, Anna Timofeeva, Luciné V. Gabriel, Ryan Allen for experimental assistance with the [2,3]-Wittig-type fragmentation, Tobias Olbrisch for the isolation of α-onocerin and Dr Jens Schmidt and Prof. Dr Christian B. W. Stark (Universität Hamburg) for experimental data and a generous donation of the Corey–Noe–Lin catalyst. The computational work was supported by the US National Science Foundation (CHE-1565933 and CHE-030089 [via XSEDE] to D. J. T.). This work was supported by JSPS KAKENHI Grant Numbers 25450149 and 16K14911 (to T. S.).
Notes and references
- J. Gershenzon and N. Dudareva, Nat. Chem. Biol., 2007, 3, 408–414 CrossRef CAS PubMed.
- R. Xu, G. C. Fazio and S. P. T. Matsuda, Phytochemistry, 2004, 65, 261–291 CrossRef CAS PubMed.
- R. Thimmappa, K. Geisler, T. Louveau, P. O'Maille and A. Osbourn, Annu. Rev. Plant Biol., 2014, 65, 225–257 CrossRef CAS PubMed.
- V. Domingo, J. F. Arteaga, J. F. Quilez del Moral and A. F. Barrero, Nat. Prod. Rep., 2009, 26, 115–134 RSC.
- B. Meunier, S. P. de Visser and S. Shaik, Chem. Rev., 2004, 104, 3947–3980 CrossRef CAS PubMed.
- M. S. Gachet, O. Kunert, M. Kaiser, R. Brun, M. Zehl, W. Keller, R. A. Muñoz, R. Bauer and W. Schuehly, J. Nat. Prod., 2011, 74, 559–566 CrossRef CAS PubMed.
- A. F. Barrero, M. M. Herrador, J. F. Quílez del Moral, P. Arteaga, J. F. Arteaga, M. Piedra and E. M. Sánchez, Org. Lett., 2005, 7, 2301–2304 CrossRef CAS PubMed.
- M. Nishizawa, H. Nishide and Y. Hayashi, Tetrahedron Lett., 1984, 25, 5071–5074 CrossRef CAS.
- R. Carman and H. Deeth, Aust. J. Chem., 1971, 24, 1099–1102 CrossRef CAS.
- E. E. van Tamelen, M. A. Schwartz, E. J. Hessler and A. Storni, Chem. Commun., 1966, 409–411 RSC.
- P. F. Vlad, K. I. Kuchkova, A. N. Aryku and K. Deleanu, Russ. Chem. Bull., 2005, 54, 2656–2658 CrossRef CAS.
- E. Romann, A. J. Frey, P. A. Stadler and A. Eschenmoser, Helv. Chim. Acta, 1957, 40, 1900–1917 CrossRef CAS.
- E. J. Corey and R. R. Sauers, J. Am. Chem. Soc., 1957, 79, 3925–3926 CrossRef CAS.
- E. J. Corey and R. R. Sauers, J. Am. Chem. Soc., 1959, 81, 1739–1743 CrossRef CAS.
- D. H. R. Barton and K. H. Overton, J. Chem. Soc., 1955, 2639–2652 RSC.
- Y. Mi, J. V. Schreiber and E. J. Corey, J. Am. Chem. Soc., 2002, 124, 11290–11291 CrossRef CAS PubMed.
- Y. Tsuda, N. Kashiwaba, M. Kajitani and J. Yasui, Chem. Pharm. Bull., 1981, 29, 3424–3426 CrossRef CAS.
- G. Stork, A. Meisels and J. E. Davies, J. Am. Chem. Soc., 1963, 85, 3419–3425 CrossRef CAS.
- N. Danieli, Y. Mazur and F. Sondheimer, Tetrahedron, 1967, 23, 509–514 CrossRef CAS.
- J. Kokosi and C. Schmidt, Synth. Commun., 1985, 15, 341–354 CrossRef CAS.
- R. F. Church, R. E. Ireland and J. A. Marshall, Tetrahedron Lett., 1961, 2, 34–38 CrossRef.
-
S. Berger and D. Sicker, Classics in Spectroscopy: Isolation and Structure Elucidation of Natural Products, Wiley-VCH, Weinheim, 2009 Search PubMed.
- D. Ueda, T. Hoshino and T. Sato, J. Am. Chem. Soc., 2013, 135, 18335–18338 CrossRef CAS PubMed.
- M. G. Rowan, P. D. G. Dean and T. W. Goodwin, FEBS Lett., 1971, 12, 229–232 CrossRef CAS PubMed.
- T. Araki, Y. Saga, M. Marugami, J. Otaka, H. Araya, K. Saito, M. Yamazaki, H. Suzuki and T. Kushiro, ChemBioChem, 2016, 17, 288–290 CrossRef CAS PubMed.
- H. Ageta, K. Shiojima and K. Masuda, Chem. Pharm. Bull., 1982, 30, 2272–2274 CrossRef CAS.
- Y. Arai, M. Yamaide, S. Yamazaki and H. Ageta, Phytochemistry, 1991, 30, 3369–3377 CrossRef CAS.
- A. S. Kleinke, D. Webb and T. F. Jamison, Tetrahedron, 2012, 68, 6999–7018 CrossRef CAS.
- H. Bouanou, J. A. Gil, R. Alvarez-Manzaneda, R. Chahboun and E. Alvarez-Manzaneda, J. Org. Chem., 2016, 81, 10002–10008 CrossRef CAS PubMed.
- M. T. Corbett and J. S. Johnson, Chem. Sci., 2013, 4, 2828–2832 RSC.
- E. J. Alvarez-Manzaneda, R. Chaboun, E. Alvarez, E. Cabrera, R. Alvarez-Manzaneda, A. Haidour and J. M. Ramos, Synlett, 2006, 2006, 1829–1834 CrossRef.
- M. Maemoto, A. Kimishima and T. Nakata, Org. Lett., 2009, 11, 4814–4817 CrossRef CAS PubMed.
- H. Shigehisa, M. Hayashi, H. Ohkawa, T. Suzuki, H. Okayasu, M. Mukai, A. Yamazaki, R. Kawai, H. Kikuchi, Y. Satoh, A. Fukuyama and K. Hiroya, J. Am. Chem. Soc., 2016, 138, 10597–10604 CrossRef CAS PubMed.
- S. Pan, J. Xuan, B. Gao, A. Zhu and H. Ding, Angew. Chem., Int. Ed., 2015, 54, 6905–6908 CrossRef CAS PubMed.
- L. Catti and K. Tiefenbacher, Chem. Commun., 2015, 51, 892–894 RSC.
- G. A. Olah, A. P. Fung and R. Malhotra, Synthesis, 1981, 1981, 474–476 CrossRef.
- L. Fang, Y. Chen, J. Huang, L. Liu, J. Quan, C.-c. Li and Z. Yang, J. Org. Chem., 2011, 76, 2479–2487 CrossRef CAS PubMed.
-
O. Piva, in Synthesis of Saturated Oxygenated Heterocycles II: 7- to 16-Membered Rings, ed. J. Cossy, Springer Berlin Heidelberg, Berlin, Heidelberg, 2014, pp. 283–320, DOI:10.1007/978-3-642-41470-1_1.
- R. B. Boar, L. A. Couchman, A. J. Jaques and M. J. Perkins, J. Am. Chem. Soc., 1984, 106, 2476–2477 CrossRef CAS.
- R. A. Yoder and J. N. Johnston, Chem. Rev., 2005, 105, 4730–4756 CrossRef CAS PubMed.
-
L. F. Tietze, G. Brasche and K. M. Gericke, Domino Reactions in Organic Synthesis, Wiley-VCH, Weinheim, 2006 Search PubMed.
-
S. A. Snyder and A. M. Levinson, in Comprehensive Organic Synthesis II, ed. P. Knochel and G. A. Molander, Elsevier, Amsterdam, 2nd edn, 2014, p. 268, DOI:10.1016/B978-0-08-097742-3.00309-8.
- M. Baunach, J. Franke and C. Hertweck, Angew. Chem., Int. Ed., 2015, 54, 2604–2626 CrossRef CAS PubMed.
- V. K. Aggarwal, P. A. Bethel and R. Giles, J. Chem. Soc., Perkin Trans. 1, 1999, 3315–3321 RSC.
- T. N. Barrett and A. G. M. Barrett, J. Am. Chem. Soc., 2014, 136, 17013–17015 CrossRef CAS PubMed.
- See ESI† for details.
- S. Nakamura, K. Ishihara and H. Yamamoto, J. Am. Chem. Soc., 2000, 122, 8131–8140 CrossRef CAS.
- M. Uyanik, H. Ishibashi, K. Ishihara and H. Yamamoto, Org. Lett., 2005, 7, 1601–1604 CrossRef CAS PubMed.
- T. Abe and T. Hoshino, Org. Biomol. Chem., 2005, 3, 3127–3139 CAS.
- D. Urabe, T. Asaba and M. Inoue, Chem. Rev., 2015, 115, 9207–9231 CrossRef CAS PubMed.
- J. Mulzer, Nat. Prod. Rep., 2014, 31, 595–603 RSC.
- Z. G. Brill, M. L. Condakes, C. P. Ting and T. J. Maimone, Chem. Rev., 2017, 117, 11753–11795 CrossRef CAS PubMed.
- T. Newhouse, P. S. Baran and R. W. Hoffmann, Chem. Soc. Rev., 2009, 38, 3010–3021 RSC.
- For an attempted synthesis, see: J. H. George, M. McArdle, J. E. Baldwin and R. M. Adlington, Tetrahedron, 2010, 66, 6321–6330 CrossRef CAS.
- C. Vaxelaire, P. Winter and M. Christmann, Angew. Chem., Int. Ed., 2011, 50, 3605–3607 CrossRef CAS PubMed.
- Y. Hayashi, Chem. Sci., 2016, 7, 866–880 RSC.
- K. K. W. Kuan, H. P. Pepper, W. M. Bloch and J. H. George, Org. Lett., 2012, 14, 4710–4713 CrossRef CAS PubMed.
- M. Matsushita, Y. Nagaoka, H. Hioki, Y. Fukuyama and M. Kodama, Chem. Lett., 1996, 25, 1039–1040 CrossRef.
- Direct conversion of the primary alcohol into a leaving group and subsequent elimination was outcompeted by intramolecular substitution forming ambroxide.
- CCDC 1529115 (13), 1529116 (4), 1529117 (22), 1529118 (10) contain the supplementary crystallographic data for this paper.†.
- E. J. Corey and H. A. Kirst, Tetrahedron Lett., 1968, 9, 5041–5043 CrossRef.
- T. Yoshida and E. Negishi, J. Am. Chem. Soc., 1981, 103, 4985–4987 CrossRef CAS.
- P. Wipf and S. Lim, Angew. Chem., Int. Ed. Engl., 1993, 32, 1068–1071 CrossRef.
- D. J. Clausen, S. Wan and P. E. Floreancig, Angew. Chem., Int. Ed., 2011, 50, 5178–5181 CrossRef CAS PubMed.
- V. Domingo, L. Lorenzo, J. F. Quilez del Moral and A. F. Barrero, Org. Biomol. Chem., 2013, 11, 559–562 CAS.
- H. C. Kolb, M. S. VanNieuwenhze and K. B. Sharpless, Chem. Rev., 1994, 94, 2483–2547 CrossRef CAS.
-
(a) E. J. Corey, M. C. Noe and S. Lin, Tetrahedron Lett., 1995, 36, 8741–8744 CrossRef CAS;
(b) J. Schmidt and C. B. W. Stark, Org. Lett., 2012, 14, 4042–4045 CrossRef CAS PubMed.
- Determination of the absolute configuration by Mosher ester analysis.
- K. Surendra and E. J. Corey, J. Am. Chem. Soc., 2008, 130, 8865–8869 CrossRef CAS PubMed.
- V. Domingo, L. Silva, H. R. Diéguez, J. F. Arteaga, J. F. Quílez del Moral and A. F. Barrero, J. Org. Chem., 2009, 74, 6151–6156 CrossRef CAS PubMed.
- D. T. Hog, F. M. E. Huber, G. Jiménez-Osés, P. Mayer, K. N. Houk and D. Trauner, Chem.–Eur. J., 2015, 21, 13646–13665 CrossRef CAS PubMed.
- M. Razzak and J. K. De Brabander, Nat. Chem. Biol., 2011, 7, 865–875 CrossRef CAS PubMed.
- C. Galli and L. Mandolini, Eur. J. Org. Chem., 2000, 3117–3125 CrossRef CAS.
- S. V. Pronin and R. A. Shenvi, Nat. Chem., 2012, 4, 915–920 CrossRef CAS PubMed.
- R. A. Shenvi and E. J. Corey, Org. Lett., 2010, 12, 3548–3551 CrossRef CAS PubMed.
- E. J. Corey and M. Sodeoka, Tetrahedron Lett., 1991, 32, 7005–7008 CrossRef CAS.
- W. H. Gerwick and W. Fenical, J. Org. Chem., 1981, 46, 22–27 CrossRef CAS.
- A. Abad, C. Agulló, M. Arnó, A. C. Cuñat, B. Meseguer and R. J. Zaragozá, J. Org. Chem., 1998, 63, 5100–5106 CrossRef CAS.
- A. Grube, M. Assmann, E. Lichte, F. Sasse, J. R. Pawlik and M. Köck, J. Nat. Prod., 2007, 70, 504–509 CrossRef CAS PubMed.
- A. W. Markwell-Heys and J. H. George, Org. Biomol. Chem., 2016, 14, 5546–5549 CAS.
- B. T. Grimberg and R. K. Mehlotra, Pharmaceuticals, 2011, 4, 681 CrossRef PubMed.
- E. L. Flannery, A. K. Chatterjee and E. A. Winzeler, Nat. Rev. Microbiol., 2013, 11, 849–862 CrossRef CAS PubMed.
- C. R. Shugrue and S. J. Miller, Chem. Rev., 2017, 117, 11894–11951 CrossRef CAS PubMed.
- R. K. Quinn, Z. A. Könst, S. E. Michalak, Y. Schmidt, A. R. Szklarski, A. R. Flores, S. Nam, D. A. Horne, C. D. Vanderwal and E. J. Alexanian, J. Am. Chem. Soc., 2016, 138, 696–702 CrossRef CAS PubMed.
- M. S. Chen and M. C. White, Science, 2010, 327, 566–571 CrossRef CAS PubMed.
- Y. Kawamata, M. Yan, Z. Liu, D.-H. Bao, J. Chen, J. T. Starr and P. S. Baran, J. Am. Chem. Soc., 2017, 139, 7448–7451 CrossRef CAS PubMed.
- W. Liu, X. Huang, M.-J. Cheng, R. J. Nielsen, W. A. Goddard and J. T. Groves, Science, 2012, 337, 1322–1325 CrossRef CAS PubMed.
- K. Chen, A. Eschenmoser and P. S. Baran, Angew. Chem., Int. Ed., 2009, 48, 9705–9708 CrossRef CAS PubMed.
- V. A. Schmidt, R. K. Quinn, A. T. Brusoe and E. J. Alexanian, J. Am. Chem. Soc., 2014, 136, 14389–14392 CrossRef CAS PubMed.
- O. F. Jeker, A. G. Kravina and E. M. Carreira, Angew. Chem., Int. Ed., 2013, 52, 12166–12169 CrossRef CAS PubMed.
- D. B. Dess and J. C. Martin, J. Org. Chem., 1983, 48, 4155–4156 CrossRef CAS.
- A. Baeyer and V. Villiger, Ber. Dtsch. Chem. Ges., 1899, 32, 3625–3633 CrossRef.
- R. R. Sauers, J. Am. Chem. Soc., 1959, 81, 925–927 CrossRef CAS.
- J. Meinwald and E. Frauenglass, J. Am. Chem. Soc., 1960, 82, 5235–5239 CrossRef CAS.
- M. Göhl and K. Seifert, Eur. J. Org. Chem., 2014, 6975–6982 CrossRef.
- D. J. Tantillo, Angew. Chem., Int. Ed., 2017, 56, 10040–10045 CrossRef CAS PubMed.
- E. J. Corey and B. E. Roberts, Tetrahedron Lett., 1997, 38, 8921–8924 CrossRef CAS.
- M. W. Lodewyk, D. Willenbring and D. J. Tantillo, Org. Biomol. Chem., 2014, 12, 887–894 CAS.
- S. R. Hare, R. P. Pemberton and D. J. Tantillo, J. Am. Chem. Soc., 2017, 139, 7485–7493 CrossRef CAS PubMed.
Footnote |
† Electronic supplementary information (ESI) available: Detailed experimental procedures, spectral data, DFT calculation, X-ray crystallographic data for 4 (CIF), 13 (CIF), 10 (CIF), 22 (CIF). CCDC 1529115–1529118. For ESI and crystallographic data in CIF or other electronic format see DOI: 10.1039/c7sc03903d |
|
This journal is © The Royal Society of Chemistry 2017 |
Click here to see how this site uses Cookies. View our privacy policy here.