Mesoporous thin film WO3 photoanode for photoelectrochemical water splitting: a sol–gel dip coating approach†
Received
11th October 2016
, Accepted 20th December 2016
First published on 9th January 2017
Abstract
A facile and cost-efficient method to fabricate a mesoporous structured WO3 photoanode was implemented for use in a tandem dual photosystem water splitting photoelectrochemical cell. Semi-transparent thin films of tungsten trioxide were fabricated by sol–gel process, incorporating a block co-polymer to induce a template-directed mesoporous structure. These thin films are deposited by dip coating onto transparent conducting oxide substrates and crystallized at a low temperature of 400 °C in air. These WO3 photoanodes exhibit a photocurrent of up to 0.6 mA cm−2 in potassium phosphate buffers of pH 2, 4, and 6 at 1.23 V vs. RHE under 300 mW cm−2 visible (400–900 nm) light irradiation with a faradaic efficiency of up to 75%. Furthermore, we have demonstrated that corrosion occurs in electrolytes of pH > 4. The faradaic efficiencies in varying pH solutions suggest that parasitic redox reactions occurs in acidic conditions, limiting the O2 production and demonstrating the need for stable surface co-catalysts to increase faradaic efficiencies. In neutral conditions, protective layers and/or co-catalysts are needed for increasing WO3 photoanode stability.
1. Introduction
Due to the increasing demand for energy, the development of renewable fuels is imperative to account for the decrease of fossil fuels which will become scarce in the near future. Hydrogen is a promising energy carrier which could potentially aid in weaning off fossil fuel dependency. An attractive solution is to directly convert solar energy into hydrogen which can be stored and re-used in the chemical industry (i.e. fertilizer production by the Haber–Bosch process) as well as energy industries, transportation, etc. One of the most promising potential approaches to this issue is the concept of water splitting photoelectrochemical cells (PECs) which combines the concepts of photovoltaics and electrolyzers – two technologies which are presently well developed, mature technologies.1–4
One of the challenges of this technology is to find suitable and robust materials for both light harvesting and catalysis of the oxygen and hydrogen evolution reactions. Transition metal oxides are encouraging materials due to the fact that they possess photovoltaic effect and are stable in aqueous solutions, which enables them to avoid potential competitive redox reactions associated with degradation and corrosion.5 Tandem PECs combine two photoelectrode materials generally an n-type semiconductor photoanode for the oxygen evolution reaction (OER) and p-type semiconductor for the hydrogen evolution reaction (HER). As most large band bap materials are n-type semiconductors, this designates them as appropriate photoanode candidates – providing the valence band position lower than the OER (1.23 V vs. RHE). The first discovery of photocatalytic characteristics in transition metal oxides for water splitting was reported in 1972 by Fujishima and Honda using rutile TiO2.6 Since then, many metal oxides, notably, α-Fe2O3, WO3, anatase TiO2, and BiVO4, have been investigated as n-type photoanode materials for the water oxidation half reaction. These photoanode materials have been additionally coupled with numerous surface co-catalysts to increase their efficiency by decreasing the photocurrent onset/overpotential, increasing faradaic efficiencies, and avoiding parasitic reactions and degradation which may compete with water splitting reactions. Typical co-catalysts used to date for the OER on n-type photoanodes are cobalt phosphate (Co-Pi),7–11 Co3O4,12,13 MnO2,14 FeOOH,15,16 NiOOH,17,18 IrO2,19,20 RuO2,21 RhO2,13 or Pt.22
Tungsten trioxide has been studied extensively as a n-type photoanode material for water oxidation in photocatalytic systems.23 It was the subsequent material selection regarding metal oxide materials for water splitting photoanodes following the discovery of TiO2.24 WO3 typically possesses a band gap of 2.7 eV, slightly smaller than that of anatase TiO2 of 3.2 eV; thus, enabling increased absorption of the solar spectrum (e.g. WO3 can utilize 12% of the solar spectrum; whereas, TiO2 can only harness 4%).25 Despite the narrow range of light absorption towards the ultraviolet range of the light spectrum, WO3 photoanodes typically display larger steady state photocurrents than α-Fe2O3 photoanodes due to the higher incident photon to current efficiency (IPCE).26 The hole diffusion length in WO3 is believed to be 150 nm while α-Fe2O3 has a limited hole diffusion length of only 2–4 nm.23,28 WO3 also possesses inherently good electron transport.27 Therefore, WO3 is considered advantageous compared to both TiO2 and α-Fe2O3 for solar absorption and conductivity.
Metal oxide thin films, specifically WO3, can be fabricated using several techniques: drop-casting,28 anodization,29–33 electrodeposition,9,34–36 chemical vapor deposition,37 spray pyrolysis,38 magnetron sputtering,39 e-beam evaporation,40 doctor blade,41–43 and spin-coating.44,45 Nanostructuration46–49 and doping have been shown to increase photocurrents by augmenting the surface area between the semiconductor and electrolyte, as well as decreasing the distance between the production sites of holes and the interface where they are used to produce oxygen.
This article examines low cost, thin film, semi-transparent, mesoporous, WO3 as an n-type photoanode for water splitting application in a tandem dual photosystem photocatalytic device. These WO3 photoactive thin films are fabricated by an inexpensive, robust, reproductive, sol–gel dip coating technique, which is easily scalable and transferrable to industrial application.
2. Experimental
2.1. Synthesis
Ethanol (99.9% ACS reagent AnalaR NORMAPUR) and tetrahydrofuran (THF, 99.9% ACS reagent AnalaR NORMAPUR) purchased from VWR. WCl6 (99.9% trace metal basis), KH2PO4 (≥99.995% TRACESELECT), K2HPO4 (≥99.9% ACS reagent) as well as H3PO4 were purchased from Sigma Aldrich. Poly(isobutylene-b-ethylene oxide) (#P4793-IbEO or #P4792-IbEO) was purchased from Polymer Source.
4.4 mL of ethanol were mixed with 1.6 mL THF with 100 mg of PIB-b-PEO in a closed vial and then placed in an oven at 70 °C until the block co-polymer was completely dissolved. Finally, 1 g WCl6 was added to the solution (cooled to room temperature) containing the polymer (0.28 M final tungsten concentration). An exothermic reaction occurred upon the addition of tungsten chloride, evolving chlorine gas which was vented, and the solution is left to stir for at least 1 hour resulting in a turquoise-blue solution. The block co-polymer was omitted in the sol for dense WO3 thin films preparation. Fluorine doped tin oxide (FTO) transparent conducting oxide substrates (Solems Asahi-100), consisting of 80 nm FTO (approximately 80 Ω per square) on 1.1 mm of glass were employed and rinsed with ethanol before thin layers were deposited. The hybrid solution was deposited onto FTO substrates by dip coating technique at 2.7 mm s−1 withdrawal speed at low relative humidity (measured with HANNA thermohygrometer HI9564) at room temperature with an annealing treatment at 350 °C for 2–5 minutes in a ceramic plate oven in air between each layer depositions. In total, each layer deposition measured about 70 nm, and several layers were consecutively dipped to increase the thickness in aim to increase the light absorption for better performance. In total, 8 layers (averaging 550 nm) were deposited by dip coating and final thermal treatment/calcination was effectuated between 400–550 °C for 30 minutes in a ceramic oven in air.
2.2. Characterization
UV-visible light absorption and transmission was measured with an Agilent Technologies Cary Series 5000 UV-visible-near infrared spectrometer using a double beam with a background of FTO defined. X-ray diffraction patterns were taken using Bragg–Brentano flat-specimen geometry on all the samples using a high-resolution Bruker D8 diffractometer using CuKα radiation and equipped with a linear position sensitive detector. The diffraction patterns were refined using the Rietveld program Xnd.50 Field emission gun scanning electron microscopy (FEG-SEM) was effectuated using a Hiatchi Oxford x-max beam SU-70 with a 50 mm2 tungsten filament at 10–15 kV. Material elements were also detected using the Hiatchi Oxford microscope for energy-dispersive X-ray spectroscopy (EDX). High resolution transmission electron microscopy (HR-TEM) was done using a JEOL JEM-2010.
2.3. Photoelectrochemical performance
Photoelectrochemical measurements were completed with an AMETEK Solartron Analytical Modulab potentiostat which measured a linear voltage sweep (LVS) of dark and illuminated samples in a 3-electrode setup. The LVS sweeps were effectuated from 0 V to 1.8 V vs. Ag/AgCl KCl saturated reference electrode (ALS Co. LTD RE-1C referenced 0.197 V vs. NHE) with a platinum wire as a counter electrode using a 10 mV s−1 scan rate. All current density curves were referenced to the reversible hydrogen electrode (RHE), according to the equation: E vs. RHE = E vs. Ag/AgCl + E0(Ag/AgCl) (0.197) + 0.059 × pH. The geometric active area of the WO3, which acts as the working electrode, is defined by Hi-Bond polyamide scotch tape (HB830-19) which measured 0.2 cm2. Electrical contacts were made by conductive copper scotch (3M Scotch 1245) which was adhered directly to the FTO exposed portion of the photoelectrode. All solutions used were 1 M potassium phosphate buffers in order to compare different pH values (0, 2, 4, and 6) while maintaining the same ionic force and anion composition. The output power of the xenon lamp was measured using a Newport 1918-R photodiode. The xenon lamp output was 300 mW cm−2 for visible light (400–900 nm) and 350 mW cm−2 for visible and ultraviolet light. J–V curves were performed either under 300 mW cm−2 illumination (when only visible light was considered) or 350 mW cm−2 (when both visible and ultraviolet light were considered) – specified in figure descriptions. Photocurrent density was measured 50 cm away from a 300 W xenon lamp (Newport Xe ozone free lamp model 6258) working at 280 W equipped with an IR water filter Oriel Liquid Filter 61945 (250–950 nm). Visible light measurements were possible using a 400 nm UV filter Oriel UV filter 51272 (cut off 400 nm). A Newport electronic shutter (model 76994) was also used for chopped light measurements with controller (model 76995, combined with a Jeulin very low frequency generator reference 293049).
For separation and catalytic efficiency, LVS measurements of 550 nm of mesoporous WO3 were effectuated under AM1.5 G illumination, back illuminated in 1 M H2SO4 (pH 0) with and without 1 M Na2SO3, 50 cm from the lamp (standard) with a Pt counter, Ag/AgCl (KCl saturated) reference using a LVS from −0.2 to 1.8 V vs. ref with a step of 10 mV and Newport 81088A Air Mass Filter, AM1.5 Global and a Newport (Xe 300 W Ozone free) lamp 67001. The quantum yield or incident photon to current efficiency (IPCE) was measured using a 300 W xenon lamp calibrated with a total power of 100 mW cm−2 output in 1 M H2SO4 (pH 0) between 300–600 nm.
All photoelectrochemical experiments were performed with backside illumination, as tests suggest the photoelectrochemical performance is similar for both back and front side illumination; thus, in the final dual tandem PEC configuration the photoanode will be employed illuminated from the back as to reduce ionic transport distance in the electrolyte relative to the photocathode.
2.4. Stability and faradaic measurements
Stability tests and faradaic efficiency of the 550 nm mesoporous WO3 film were performed under 350 mW cm−2 light (roughly 300–900 nm) 50 cm from the lamp like LVS measurements using an active area of 1 cm2 defined by electrolytic scotch tape. These measurements were tested in a laboratory fabricated hermetic H-cell with a plastic window allowing a 3-electrode setup separated by glass frits. Oxygen in the headspace of the cell was detected with a Perkin Elmer Clarus 580 gas chromatograph TCD at 32 °C with nitrogen carrier gas. Oxygen in solution was measured simultaneously with a Unisense Ox-N 13987 Clark electrode calibrated at 40 °C (due to increase in solution temperature under illumination during testing) with a two point calibration in air saturated solution and deoxygenated solution. 1 M potassium phosphate solutions of pH 2, 4, and 6 were used to compare oxygen evolution and stability over time.
2.5. Electrocatalyst deposition
Cobalt phosphate was photo-electrodeposited onto the WO3 in pH 6 using a 0.5 mM cobalt concentration in 0.1 M potassium phosphate solution. The deposition was completed for 1, 5, or 10 minutes with 300 mW cm−2 illumination with platinum mesh counter electrode (2 cm2 active area exposed to catalyst deposition – back illuminated). Samples were rinsed with deionized water and dried with compressed air after deposition. 5 minute depositions were found to perform the best of the varied deposition times and resulting in dispersed cobalt over the WO3 surface.
3. Results and discussion
3.1. Structure
Calcination treatment of the thin films at 400 °C resulted in a distorted β-orthorhombic/γ-monoclinic WO3 structure with crystal orientation preferential to the (1 0 0) plane modeled from refined diffraction patterns using the orthorhombic β polymorph.50,51
X-ray diffraction patterns (XRD) suggest the WO3 crystal system orients orthorhombic along the a-plane and monoclinic in b/c plane shown in Fig. 1. Lattice parameters are calculated to be 7.34 Å, 6.89 Å, and 7.65 Å for a, b, and c planes, respectively.
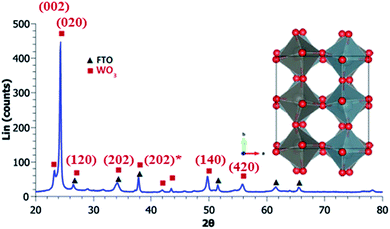 |
| Fig. 1 XRD CuKα pattern of mesoporous WO3 (indicated by red squares and indexed peaks are labeled in red for the orthorhombic phase, only one peak corresponds to orthorhombic phase, noted*) on glass FTO substrate (triangles). | |
The WO3 film exhibits a mesoporous nanostructuration, with an average thickness of 550 nm from 8 consecutive depositions by dip coating (Fig. 2). The pores have an average of 20 nm diameter size verified by HR-TEM (Fig. 2e and f). The film nanostructure is columnar, consisting of needle-shaped crystallites (see Fig. 2b and d). The needle cross-sections normal to their axis are randomly oriented in the plane perpendicular to the sample surface, while their a-axis is always well aligned along the direction normal to the sample surface (see Fig. 2b and d).
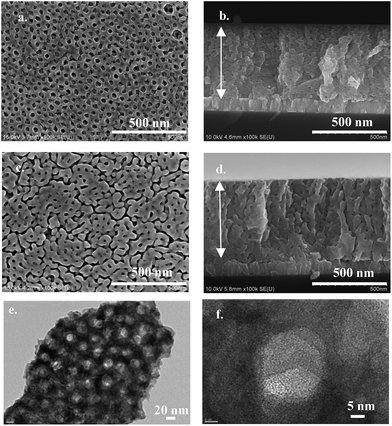 |
| Fig. 2 FEG-SEM images of WO3 thermally treated at (a) 400 °C, (b) corresponding cross section at 400 °C, (c) WO3 thermally treated at 500 °C and (d) cross section at 500 °C, (e and f) HR-TEM images of mesoporous WO3 flakes calcinated at 400 °C dispersed in ethanol at 50k (e) and 400k magnification (f). | |
The absorption and transmission spectra of WO3 are seen in the UV-visible transmission spectrum in Fig. 3a. The absorption edge of WO3, according to UV-visible absorption spectra, is found around 430 nm confirming a large band gap. The band gap of sol gel processed WO3 was estimated from absorption and transmission data, using Tauc plot representation. A linear fit suggested a value of 2.89 eV (inserted in Fig. 3a).52 Fringes are seen in the visible and near-infrared portion of the transmittance spectrum and are most likely due to the refraction of WO3 pores and dip coated layers. The WO3 thin film photoanode is visually transparent with a slight yellow tint. This slight yellow tint indicates a large band gap, allowing shorter wavelengths to 430 nm to pass to the photocathode to be absorbed in an envisioned tandem dual photosystem photocatalytic cell configuration.
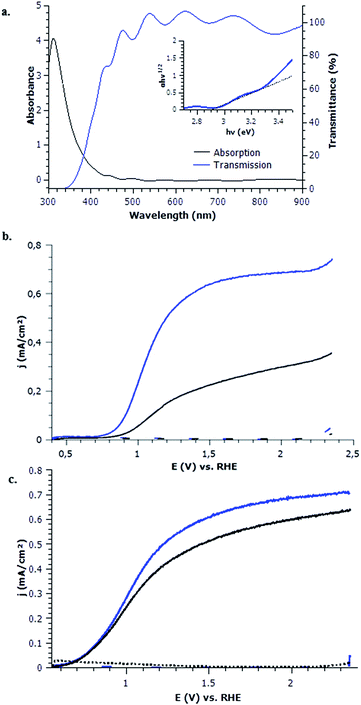 |
| Fig. 3 (a) UV-visible absorption (black) and transmission (blue) spectra of mesoporous WO3 with interlaced Tauc plot suggesting band gap of 2.89 eV, (b) linear voltage sweep of mesoporous (blue) and dense (black) WO3 electrodes in 1 M potassium phosphate solution under visible light with corresponding FEG-SEM, (c) linear voltage sweep of mesoporous WO3 thermally treated at 400 °C (blue) and 500 °C (black) upon visible illumination. | |
3.2. Photoelectrochemical performance
The photoelectrochemical performance of mesoporous WO3 photoanode has been evaluated in a three electrode cell upon linear sweep voltage in pH 2–6 potassium phosphate solutions.
3.2.1. Impact of the nanostructuration.
The impact of the nanostructuration on the photocurrent tested by linear voltage sweeps was measured on 550 nm thick mesoporous and dense thin films of WO3 heat-treated at 400 °C (Fig. 3b). Upon illumination, the onset potential decreases to 0.6 V vs. RHE – a major cathodic shift of 1.7 V comparable to other literature values.49 Visible light response (400 nm–950 nm) of WO3 results in a photocurrent of up to 0.55 mA cm−2 at 1.23 V vs. RHE with saturated photocurrent of 0.7 mA cm−2 reached at 1.5 V vs. RHE under 300 mW cm−2 xenon lamp output power density. The mesoporous WO3 thin film had a larger photocurrent response than the dense WO3 thin film (Fig. 3b). The photocurrent differences indicate that mesostructuration of thin films creates a larger surface area exposure to the electrolyte, increasing the photoelectrochemical performance for water splitting applications. After correction for film thickness, the 0.55 mA cm−2 photocurrent value measured at 1.23 V vs. RHE under 100 mW cm−2 visible-light illumination (see Fig. S1, ESI†) is comparable to that reported for another mesoporous WO3 film reported by Yagi and coworkers.28
3.2.2. Impact of the heat-treatment.
Thermal treatment of 400 °C and 500 °C in air was applied to WO3 thin films to understand the impact of temperature on the photoelectrochemical performance. The layers treated at 500 °C exhibited a slightly lesser photocurrent in acidic to neutral conditions than when treated at 400 °C (Fig. 3c). This modification is attributed to the change of the porosity network upon thermal treatment at 500 °C, as seen on FEG-SEM images in Fig. 2 (Fig. 2bvs.2d). The electrode/electrolyte interface is probably less defined in 500 °C calcinated thin films, decreasing the overall performance of the electrode.
3.2.3. Understanding physical limitations of WO3 mesoporous films.
Impact of UV light.
Upon removal of the ultraviolet optical filter (cut off 400 nm), the photocurrent of WO3 is greatly increased due to the absorption of additional UV wavelengths as seen in Fig. 4a. This UV light (360–400 nm) addition increases photocurrent from 0.55 mA cm−2 to 6.5 mA cm−2 at 1.23 V vs. RHE under 350 mW cm−2 illumination. This increase upon addition of UV light is in line with IPCE measurements (Fig. 4c). Under AM1.5 G 100 mW cm−2 illumination, the photocurrent value is 0.4 mA cm−2 at 1.23 V vs. RHE Fig. 4a. Compared to other state of the art WO3 photoanodes for the OER in a tandem dual photosystem, the dip coated FTO/WO3 photoanode performance found in literature reports 2.7 mA cm−2 for 6 μm of WO3 under 100 mW cm−2 illumination in pH 0 H2SO4 at 1.4 V vs. RHE. Seeing as our WO3 photoelectrodes are 10× less thick, this suggests that the performances is comparable. IPCE measurements exhibit a maximum of 95% at 310 nm for mesoporous WO3 treated at 400 °C as shown in Fig. 4b. The UV-visible absorption spectra as well as WO3 IPCE responses resemble very much those obtained by other groups.40 Upon calculation of the light penetration depth from UV-visible absorbance measurements and thickness (shown in Fig. S2, ESI†), it seems that all incident light of wavelengths up to 370 nm are absorbed. Absorption losses start to occur between 370–430 nm and efforts should be taken to increase absorption towards this range of the solar spectrum to increase future performance.
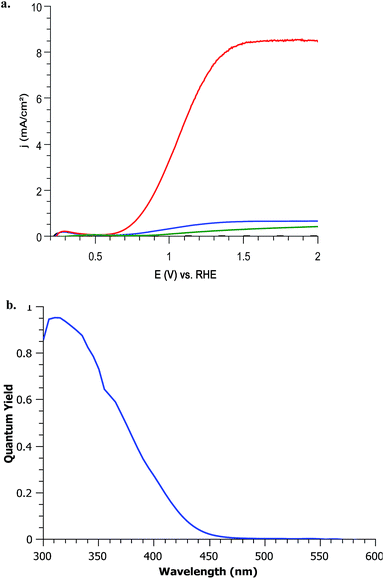 |
| Fig. 4 (a) WO3 linear voltage scan in 1 M (pH 6) potassium phosphate buffer under 300 mW cm−2 visible light illumination (blue) and with addition of UV light 350 mW cm−2 (red), and under AM1.5 (in green) (b) quantum yield of mesoporous WO3 at 1.23 V vs. RHE in 1 M H2SO4 (pH 0). | |
To further understand the physical limitations and characteristics of the WO3 mesoporous films, electrochemical impedance measurements were conducted in 1 M potassium phosphate to measure the flat band potential (EFB) by Mott–Schottky technique (Fig. S3, ESI†). Measurements taken at 500–1000 Hz with 10 mV amplitude suggest that the EFB of WO3 is at 0.37 V vs. RHE (shown by dotted line in Fig. S3, ESI†). This measured flat band value compared well with thin film WO3 photoanodes.24,53 This value, compared to the illuminated onset potential (0.60 vs. RHE) in Fig. 4, indicates an OER overpotential requirement of >0.2 V for this WO3 photoanode. The difference between the flat band potential and the onset value of photocurrent is related to fast recombination of photogenerated carriers together with slow OER kinetics at the material–electrolyte interface.
The impact of light intensity was then studied to determine whether the reaction is limited by surface kinetic or by photocarrier generation. Upon increasing the light intensity from 100 mW cm−2 to 300 mW cm−2 a linear trend is observed for the WO3 mesoporous thin films regarding the current density and light intensity (shown in Fig. S2, ESI†). Thus, charge carrier generation and/or recombination in the bulk material can be considered a potential rate limiting step in WO3 for the OER. These efficiency limiting processes are generally observed with large band gap semiconductors.24 The linear response of photocurrent with light intensity suggest that surface oxidation kinetics do not participate in limiting overall photoelectrochemical performance of mesoporous sol–gel processed thin film WO3 photoanodes under these conditions.
Surface limitation.
To confirm that surface oxidation kinetics do not participate in limiting overall photoelectrochemical performance in these mesoporous WO3 photoanodes, the photocurrent response was tested with chopped light under chronoamperometric conditions at 1.23 V vs. RHE (Fig. 5a).
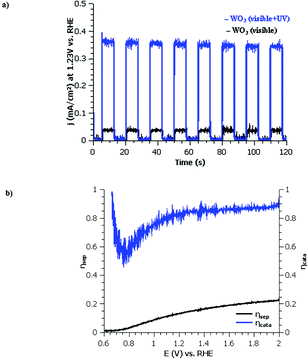 |
| Fig. 5 (a) Light response of WO3 under 100 mW cm−2 visible irradiation (black) and upon addition of UV light (blue), (b) charge separation efficiency (black) and surface catalytic efficiency of WO3 in 1 M potassium phosphate buffer (pH 6) under 100 mW cm−2 illumination using a sacrificial reagent 1 M H2O2. | |
The photocurrent current displays a square response, suggesting the photoresponse to light is rapid, exhibiting no transient photocurrent. This behavior suggests that the surface extraction of holes is more rapid than the arrival charge carriers at the semiconductor liquid junction (SCLJ). Therefore, overall efficiency is limited by material bulk properties.
To better quantify the surface reactivity, a sacrificial reagent (1 M H2O2 or Na2SO3) was added to the electrolyte (Fig. 5b). Under these conditions, 100% surface catalytic efficiency can be assumed due to the kinetically favoured reactions with the reagent anions compared to the kinetically restrained OER. The method of Dotan, et al. was used to quantify absorption losses under AM1.5 G irradiation conditions.54 The mesoporous WO3 thin films absorb only 55% of the light considering a band gap of 2.89 eV according to UV-visible transmission data. When applied to the solar spectrum, this limits the theoretical maximum photocurrent to 2.13 mA cm−2 compared to the theoretical maximum of 3.88 mA cm−2 (obtained by integration of the AM1.5 G spectra assuming no optical or quantum losses for a band gap of 3.89 eV). The photoelectrochemical performance by linear voltage sweeps under AM1.5 G illumination using an Oriel AM1.5 G filter and a xenon lamp with a power output of 100 mW cm−2 display a photocurrent of only 0.34 mA cm−2. Upon the addition of the sacrificial reagent, this photocurrent only slightly increased to 0.40 mA cm−2 at 1.23 V vs. RHE. Assuming 100% surface catalytic efficiency for the photocurrent with sacrificial reagent, this corresponds to a charge separation efficiency of only 18% using the method of Dotan et al. Using this assumption, a surface catalytic efficiency of 85% at water splitting potentials in potassium phosphate buffer was calculated (displayed in Fig. 6b).
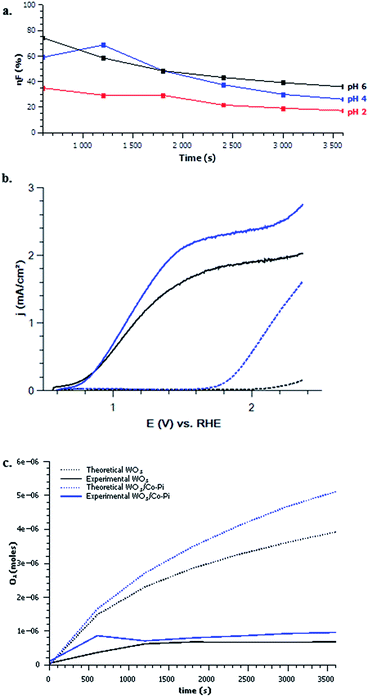 |
| Fig. 6 (a) Faradaic efficiencies measured by charge, gas chromatograph, and Clark electrode data from WO3 in 1 M potassium phosphate of varying pH at 1.5 V vs. RHE under 350 mW cm−2 irradiation, (b) linear voltage sweep of WO3 (black, plain line: light, dash line: dark) and WO3/Co-Pi (blue, plain line: light; dash line: dark) in 1 M potassium phosphate buffer (pH 6) under visible light (350 mW cm−2) illumination, (c) experimental moles of O2 measured (solid line) and theoretical moles (dotted line) of O2 calculated for WO3 (black) and WO3/Co-Pi (blue) thin films in 1 M potassium phosphate buffer (pH 6) under 350 mW cm−2. | |
To confirm that conductivity of WO3 is the limiting factor in these mesoporous WO3 films, time resolved microwave conductivity (TRMC) measurements were performed to obtain charge carrier mobility (μ) and lifetime (τ) from the change in the photoconductance of the WO3 mesoporous thin film (shown in Table S1, ESI and Fig. S4, ESI†).55 The charge carrier diffusion lengths were calculated using the following relationship,
, where μ is the mobility (m2 V−1 s−1), k is the Boltzmann constant, and T is the temperature (K), e is the electronic charge (C), and τ is the charge carrier lifetime (s). These measurements propose a maximum diffusion length of 130 nm in mesoporous WO3, slightly smaller to that reported in literature (150 nm).23 For 550 nm thin films, these diffusion lengths are enough to extract holes at the SCLJ for the OER. This diffusion length could potentially be increased by doping WO3 thin films or further optimization of the mesoporous network. Nevertheless, metal oxides are known to be poor absorbers of light and possess poor absorption coefficients.56 WO3 is an indirect band gap semiconductor (necessitating phonons in addition to photons in order to photogenerate charge carriers). Indirect semiconductors generally require thicker layers for increased light absorption for effective photogenerated charge carrier generation. This is reflected in literature studies concerning WO3 as a photoanode, using thin film thicknesses of at least 1 μm, which is twice as thick as the mesoporous thin film in this study.30,32,57–60 The absorption spectra, IPCE measurements, and addition of a sacrificial reagent all suggest that there are absorption losses at lower wavelength energies which contribute to the performance limitation of WO3 as well as the poor separation efficiency in the bulk material. Thus, optical absorption and internal bulk material conductivity seem to be the main limitations in photoelectrochemical activity in these mesoporous WO3 thin films used for the OER in water splitting applications.
Stability.
WO3 mesoporous films were tested under constant illumination in potassium phosphate buffer in acidic to neutral conditions to further understand aspects which affect OER kinetics as well as stability and faradaic efficiency. To test stability under continuous operation, the photocurrent and the faradaic efficiency of WO3 photoanodes were measured in potassium phosphate buffer at pH 2, 4, and 6 at 1.5 V vs. RHE under 350 mW cm−2 irradiation for 1 hour with UV-visible light (Fig. 6a and S5, ESI†). The corresponding photocurrents are shown in Fig. S5a, ESI.† A decrease in photocurrent and faradaic efficiency were observed especially at near neutral pH. The WO3 photocurrents decreased slowly overtime in pH 2, 4, and considerably in pH 6. This decrease in photocurrent and faradaic efficiency is directly related to the photo dissolution of WO3 (formation of WO42− anions) in neutral to basic solutions, as indicated by the Pourbaix diagram for tungsten.35 This corrosion is confirmed by post-mortem FEG-SEM analyses of the photoanodes after 1 hour of measurements (Fig. S6, ESI†). Further, Fig. 6a indicates that the corrosion of WO3 only occurs for pH values >4 under oxidizing conditions. The low faradaic efficiency observed may also be attributed to the formation of peroxo species such as S2O82− at the surface of WO3 photoanode as it has been suggested.61 Additionally, the slight decrease in photocurrent for WO3 photoanodes in acidic conditions may be due to blocking of the WO3 surface by peroxo species typically associated with incomplete O2 production or O2 bubble trapping at the surface of the electrode modifying the interface exposed to the electrolyte. It is thought that formation of O2 is preferred over peroxo species at higher pH.21 Therefore, stability in neutral to basic conditions and O2 formation in acidic conditions limit the performance of WO3 for the OER.
Impact of the addition of electrocatalyst.
Addition of a co-catalyst on photoelectrodes can potentially ameliorate the water splitting surface kinetics and reduce surface recombination. The impact of the addition of electrocatalyst on the surface of these mesoporous WO3 thin films was investigated (Fig. 6b). A reported OER electrocatalyst, cobalt phosphate (Co-Pi), was photo-electrodeposited onto the WO3 at pH 6, as it has been reported to increase the performance of α-Fe2O3.35,62 According to the deposition conditions described in the Experimental section, the quantity of electrocatalyst deposited was about 3% atomic cobalt constitution compared to tungsten onto the mesoporous WO3 thin film surface after 5 minutes. This was verified by EDX measurements (see Fig. S7, ESI†). The linear voltage sweep photoresponse of a successful 5 minute Co-Pi deposition on mesoporous WO3 compared to bare mesoporous WO3 is shown in Fig. 6b. The addition of Co-Pi failed to exert a cathodic shift of the onset potential; however, the photocurrent slightly increased compared to bare WO3 near the OER equilibrium potential.9
Faradaic testing in 1 M potassium phosphate (pH 6) has been performed for bare mesoporous and for WO3/Co-Pi. Initially, the WO3/Co-Pi film had a larger O2 production than bare WO3 (Fig. 6c), but after time it exhibit faradaic efficiency similar to that of the bare mesoporous WO3. With time, the mesoporous WO3 thin films decorated with Co-Pi still corroded overtime. This observation may suggest that Co-Pi detaches from the surface and dissolves in the solution as it has been previously demonstrated in various reports.10,10,63–69
4. Conclusion
Thin films of WO3 obtained by sol–gel dip coating appear to be a promising lead as low cost n-photoanode materials for use in acidic water splitting technologies. WO3 photoanodes for the OER were indeed successfully fabricated by the energy-efficient sol–gel dip coating method, furthermore allowing for a control of the microstructure of the films. Nanostructuration increases the performance. We have shown that the mesoporous films exhibit larger photocurrents than dense layers of WO3, which is attributed to their larger specific surface area. The performance of our sol–gel dip coated WO3 compared with other reported work.42,70,71 At AM1.5 G conditions, a photocurrent of 0.4 mA cm−2 has been measured for roughly 550 nm mesoporous WO3 thin films. The surface, though struggling with peroxo species in acidic conditions and stability in neutral conditions, is not the rate limiting step for WO3. Photo-electrochemical measurements performed under various experimental conditions (e.g. pH, cell configuration, co-catalyst addition and stability, etc.) demonstrate that there is a compromise between stability and light-driven OER efficiency. Under acidic conditions, WO3 thin film photoanodes suffer from poor OER kinetics and an appropriate surface co-catalyst is required to increase the surface kinetics and favour the production of O2 over parasitic reactions. Though there are not many known OER co-catalysts stable in acidic conditions, further research is needed to lower the overpotential and increase photocurrent values at WO3 photoanodes under such conditions.
Acknowledgements
Imaging and microanalysis was performed on a SU-70 Hitachi FEG-SEM and a X-Max Oxford EDX detector, instruments facilitated by the IMPC (Institut des Matériaux de Paris Centre FR2482) financially supported by the C'Nano projects of the Region Ile-de-France. Time resolved microwave conductivity measurements were completed at the Helmholtz Zentrum solar fuels department with Dennis Friedrich and Roel Van de Krol. This work was financed by Total Energies Nouvelles and supported by the French National Research Agency (Labex program, ARCANE, ANR-11-LABX-0003-01).
References
- G. Peharz, F. Dimroth and U. Wittstadt, Int. J. Hydrogen Energy, 2007, 32, 3248–3252 CrossRef CAS.
- S. Licht, B. Wang, S. Mukerji, T. Soga, M. Umeno and H. Tributsch, Int. J. Hydrogen Energy, 2001, 26, 653–659 CrossRef CAS.
- S. A. Bonke, M. Wiechen, D. R. MacFarlane, L. Spiccia and N. R. d. Tacconi, Energy Environ. Sci., 2015, 8, 2791–2796 CAS.
- J. W. Ager, M. R. Shaner, K. A. Walczak, I. D. Sharp and S. Ardo, Energy Environ. Sci., 2015, 8, 2811–2824 CAS.
- M. Grätzel, Nature, 2001, 414, 338–344 CrossRef PubMed.
- A. Fujishima and K. Honda, Nature, 1972, 238, 37–38 CrossRef CAS PubMed.
- M. W. Kanan, Y. Surendranath and D. G. Nocera, Chem. Soc. Rev., 2009, 38, 109–114 RSC.
- S. K. Pilli, T. E. Furtak, L. D. Brown, T. G. Deutsch, J. A. Turner and A. M. Herring, Energy Environ. Sci., 2011, 4, 5028–5034 CAS.
- J. A. Seabold and K.-S. Choi, Chem. Mater., 2011, 23, 1105–1112 CrossRef CAS.
- A. Irshad and N. Munichandraiah, J. Phys. Chem. C, 2013, 117, 8001–8008 CAS.
- D. A. Lutterman, Y. Surendranath and D. G. Nocera, J. Am. Chem. Soc., 2009, 131, 3838–3839 CrossRef CAS PubMed.
- M. Grzelczak, J. Zhang, J. Pfrommer, J. Hartmann, M. Driess, M. Antonietti and X. Wang, ACS Catal., 2013, 3, 383–388 CrossRef CAS.
- Y. Park, K. J. McDonald and K.-S. Choi, Chem. Soc. Rev., 2013, 42, 2321–2337 RSC.
- M. Huynh, D. K. Bediako, Y. Liu and D. G. Nocera, J. Phys. Chem. C, 2014, 118, 17142–17152 CAS.
- J. A. Seabold, K. Zhu and N. R. Neale, Phys. Chem. Chem. Phys., 2014, 16, 1121–1131 RSC.
- J. A. Seabold and K.-S. Choi, J. Am. Chem. Soc., 2012, 134, 2186–2192 CrossRef CAS PubMed.
- L. Trotochaud and S. W. Boettcher, Scr. Mater., 2014, 74, 25–32 CrossRef CAS.
- F. Lin and S. W. Boettcher, Nat. Mater., 2013, 13, 81–86 CrossRef PubMed.
- Y. Lee, J. Suntivich, K. J. May, E. E. Perry and Y. Shao-Horn, J. Phys. Chem. Lett., 2012, 3, 399–404 CrossRef CAS PubMed.
- M. Yagi, E. Tomita, S. Sakita, T. Kuwabara and K. Nagai, J. Phys. Chem. B, 2005, 109, 21489–21491 CrossRef CAS PubMed.
- R. Kötz and S. Stucki, Electrochim. Acta, 1986, 31, 1311–1316 CrossRef.
- S. Trasatti, Electrochim. Acta, 1984, 29, 1503–1512 CrossRef CAS.
- X. Liu, F. Wang and Q. Wang, Phys. Chem. Chem. Phys., 2012, 14, 7894–7911 RSC.
- M. A. Butler, J. Appl. Phys., 1977, 48, 1914–1920 CrossRef CAS.
- M. S. Prévot and K. Sivula, J. Phys. Chem. C, 2013, 117, 17879–17893 Search PubMed.
- B. D. Alexander, P. J. Kulesza, I. Rutkowska, R. Solarska and J. Augustynski, J. Mater. Chem., 2008, 18, 2298–2303 RSC.
- M. A. Butler, R. D. Nasby and R. K. Quinn, Solid State Commun., 1976, 19, 1011–1014 CrossRef CAS.
- D. Chandra, K. Saito, T. Yui and M. Yagi, Angew. Chem., 2013, 52, 1–5 CrossRef.
- S. Caramori, V. Cristino, L. Meda, A. Tacca, R. Argazzi and C. A. Bignozzi, Energy Procedia, 2012, 22, 127–136 CrossRef CAS.
- V. Cristino, S. Caramori, R. Argazzi, L. Meda, G. L. Marra and C. A. Bignozzi, Langmuir, 2011, 27, 7276–7284 CrossRef CAS PubMed.
- K. R. Reyes-Gil, C. Wiggenhorn, B. S. Brunschwig and N. S. Lewis, J. Phys. Chem. C, 2013, 117, 14947–14957 CAS.
- A. Tacca, L. Meda, G. Marra, A. Savoini, S. Caramori, V. Cristino, C. A. Bignozzi, V. G. Pedro, P. P. Boix, S. Gimenez and J. Bisquert, ChemPhysChem, 2012, 13, 3025–3034 CrossRef CAS PubMed.
- C. A. Bignozzi, S. Caramori, V. Cristino, R. Argazzi, L. Meda and A. Tacca, Chem. Soc. Rev., 2013, 42, 2228–2246 RSC.
- Q. Mi, A. Zhanaidarova, B. S. Brunschwig, H. B. Gray and N. S. Lewis, Energy Environ. Sci., 2012, 5, 5694–5699 CAS.
- Q. Mi, R. H. Coridan, B. S. Brunschwig, H. B. Gray and N. S. Lewis, Energy Environ. Sci., 2013, 6(9), 2646–2653 CAS.
- J. C. Hill and K.-S. Choi, J. Phys. Chem. C, 2013, 116, 7612–7620 Search PubMed.
- K. Sivula, F. Le-Formal and M. Grätzel, Chem. Mater., 2009, 21, 2862–2867 CrossRef CAS.
- A. Enesca, A. Duta and J. Schoonman, Thin Solid Films, 2007, 515, 6371–6374 CrossRef CAS.
- A. Stepanovich, K. Sliozberg, W. Schuhmann and A. Ludwig, Int. J. Hydrogen Energy, 2012, 37, 11618–11624 CrossRef CAS.
- X. Shi, I. Y. Choi, K. Zhang, J. Kwon, D. Y. Kim, J. K. Lee, S. H. Oh, J. K. Kim and J. H. Park, Nat. Commun., 2016, 7, 11943 CrossRef CAS PubMed.
- S. Han, J. Li, X. Chen, Y. Huang, C. Liu, Y. Yang and W. Li, Int. J. Hydrogen Energy, 2012, 37, 16810–16816 CrossRef CAS.
- M. Yagi, S. Maruyama, K. Sone, K. Nagai and T. Norimatsu, J. Solid State Chem., 2008, 181, 175–182 CrossRef CAS.
- P. J. Barczuk, A. Krolikowska, A. Lewera, K. Miecznikowski, R. Solarska and J. Augustynski, Electrochim. Acta, 2013, 104, 282–288 CrossRef CAS.
- I. Fujimoto, N. Wang, R. Saito, Y. Miseki, T. Gunji and K. Sayama, Int. J. Hydrogen Energy, 2013, 39, 2454–2461 CrossRef.
- H. W. Jeong, T. H. Jeon, J. S. Jang, W. Choi and H. Park, J. Phys. Chem. C, 2013, 117, 9104–9112 CAS.
- S. Shin, J. S. Kim, I. J. Park, M. H. Lee, K. S. Honga and I. S. Cho, J. Mater. Chem. A, 2015, 3, 2920–2926 Search PubMed.
- T. Brezesinski, S. Sallard, M. Antonietti and B. M. Smarsly, Small, 2006, 2, 1203–1211 CrossRef CAS PubMed.
- K. R. Reyes-Gil, B. S. Brunschwig and N. S. Lewis, J. Phys. Chem. C, 2013, 117, 14947–14957 CAS.
- B. Yang, E. Drabarek, P. R. F. Barnes and V. Luca, Chem. Mater., 2007, 19, 5664–5672 CrossRef CAS.
- B. O. Loopstra and H. M. Rietveld, Acta Crystallogr., Sect. B: Struct. Crystallogr. Cryst. Chem., 1969, 25, 1420–1421 CrossRef CAS.
- E. Salje, Acta Crystallogr., Sect. B: Struct. Crystallogr. Cryst. Chem., 1977, 33, 574–577 CrossRef.
-
J. I. Pankove, Optical Processes in Semiconductors, Dover Publications, New York, 1971 Search PubMed.
- S. J. Hong, S. Lee, J. S. Jang and J. S. Lee, Energy Environ. Sci., 2011, 4, 1781–1787 CAS.
- H. Dotan, K. Sivula, M. Grätzel, A. Rothschild and S. C. Warren, Energy Environ. Sci., 2011, 4, 958–964 CAS.
- F. F. Abdi, T. J. Savenije, M. M. May, B. Dam and R. van de Krol, J. Phys. Chem. Lett., 2013, 4, 2752–2757 CrossRef CAS.
- M. Woodhouse and B. A. Parkinson, Chem. Soc. Rev., 2009, 38, 197–210 RSC.
- B. Marsen, E. L. Miller, D. Paluselli and R. E. Rocheleau, Int. J. Hydrogen Energy, 2007, 32, 3110–3115 CrossRef CAS.
- W. Li, J. Li, X. Wang, J. Ma and Q. Chen, Int. J. Hydrogen Energy, 2010, 35, 13137–13145 CrossRef CAS.
- J. K. Kim, K. Shin, S. M. Cho, T.-W. Lee and J. H. Park, Energy Environ. Sci., 2011, 4, 1465–1470 CAS.
- F. Amano, M. Tian, G. Wu, B. Ohtani and A. Chen, ACS Appl. Mater. Interfaces, 2011, 3, 4047–4052 CAS.
- K. Fuku, Y. Miseki, T. Funaki and K. Sayama, ChemSusChem, 2015, 8, 1593–1600 CrossRef CAS PubMed.
- W. Hamd, S. Cobo, J. Fize, G. Baldinozzi, W. Schwartz, M. Reymermier, A. Pereira, M. Fontecave, V. Artero, C. Laberty-Robert and S. Clement, Phys. Chem. Chem. Phys., 2012, 14, 13224–13232 RSC.
- P. Bornoz, F. F. Abdi, S. D. Tilley, B. Dam, R. van de Krol, M. Graetzel and K. Sivula, J. Phys. Chem. C, 2014, 118, 16959–16966 CAS.
- D. R. Gamelin, Nature Chem., 2012, 4, 965–967 CrossRef CAS PubMed.
- L. G. Bloor, P. I. Molina, M. D. Symes and L. Cronin, J. Am. Chem. Soc., 2014, 136, 3304–3311 CrossRef CAS PubMed.
- S. G. B. Klahr, F. Fabregat-Santiago, T. Hamann and J. Bisquert, J. Am. Chem. Soc., 2012, 134, 4294–4302 CrossRef PubMed.
- D. K. Zhong, M. Cornuz, K. Sivula, M. Grätzel and D. R. Gamelin, Energy Environ. Sci., 2011, 4, 1759–1764 CAS.
- M. R. Nellist, F. Lin, T. J. Mills and S. W. Boettcher, Acc. Chem. Res., 2016, 49, 733–740 CrossRef CAS PubMed.
- D. H. W. Li, S. W. Sheehan, Y. He, J. E. Thorne, X. Yao, G. W. Brudvig and D. Wang, Energy Environ. Sci., 2016, 9, 1794–1802 Search PubMed.
- D.-D. Qin, C.-L. Tao, S. A. Friesen, T.-H. Wang, O. K. Varghese, N.-Z. Bao, Z.-Y. Yang, T. E. Mallouk and C. A. Grimes, Chem. Commun., 2012, 48, 729–731 RSC.
- J. K. Kim, J. H. Moon, T.-W. Leec and J. H. Park, Chem. Commun., 2012, 48, 735–737 RSC.
Footnote |
† Electronic supplementary information (ESI) available. See DOI: 10.1039/c6se00001k |
|
This journal is © The Royal Society of Chemistry 2017 |
Click here to see how this site uses Cookies. View our privacy policy here.