Ultra-small and low crystalline CoMoO4 nanorods for electrochemical capacitors
Received
27th October 2016
, Accepted 6th December 2016
First published on 9th January 2017
Abstract
Molybdenum and cobalt binary oxide (CoMoO4) nanowires, nanorods and micro-rhombohedra were synthesized by facile hydrothermal/solvothermal methods. Of all the structures, the ultra-small nanorods showed pseudocapacitive nature, high coulombic efficiency, and the best overall performance as compared to the other electrodes, with a maximum specific capacitance of 420 F g−1 at 1 A g−1. Besides, the rate capability and galvanostatic cycling test revealed their good rate capability and cycling stability. More importantly, the CoMoO4 nanorods and a pure graphene hydrogel were used to assemble an asymmetric electrochemical capacitor, which exhibited improved power density and energy density when compared to the performances of some transition metal oxide based full cells. CoMoO4 nanorods possess large specific capacitance, rate capability and long-term performance. The enhanced electrochemical behaviors of nanorods show that controlling the nano-architecture and crystallinity could be a simple and effective strategy to boost electrochemical energy capacitive capabilities.
1. Introduction
Electrochemical capacitors (ECs) have small size and low mass, high power density, fast charging performance, excellent cycling stability, thus they can play an intermediate role between batteries and electrostatic capacitors as energy storage devices. Therefore, they have emerged as one of the most important candidates for the new-generation energy storage facilities.1–3 Electrochemical capacitors have already been used in many applications including mobile electronics and transportation because of their aforementioned unique and superior characteristics,4 while such satisfactory results are unachievable in batteries and electrostatic capacitors. In recent years, the contribution of electricity from renewable sources (wind, solar, tidal etc.) has still been minimal as the supply has still been intermittent.5 Hence, under this circumstance, energy storage technology should be well developed to ensure a more stable and continuous energy supply from renewable energy. Electrochemical capacitors, which exhibit very high energy and power density, have proven to be highly efficient energy storage devices. Thus, ECs could contribute to the development of sustainable energy and fuels significantly.
Commercial electrochemical capacitors at the current stage are strongly dominated by high-surface-area carbonaceous materials,6 notwithstanding their potential limitations namely insufficient energy/power densities as well as drastic decrease in specific resistance with high current. Hence, transition metal oxide materials that have drawn tremendous attention because of their high pseudocapacitance due to fast redox reactions on the surface7–10 have been the focus in the recent research fields. Furthermore, their feasibility in the development of high-energy density ECs can be realized due to their remarkable capacitance. Therefore, significant attention has been emphasized on exploiting transition metal oxides as EC electrodes in order to improve their capacitance and performance at high current densities.
Transition-metal oxides are a promising choice of materials for the manufacture of electrodes for electrochemical capacitors because of their multiple oxidation states, which may incur abundant pseudocapacitance.11,12 As a whole, binary metal oxides exhibit higher capacitive performances than single metal oxides because of their multiple oxidation states and better electroconductivity,13,14 thus proving to be a probable candidate material for high performance electrochemical capacitors.15,16 As such, ruthenium oxides have fulfilled the necessary requirement for effective capacitor fabrication, however, scalability is hampered by their scarcity and high material cost.17 As for cobalt oxides, Xu et al. reported a facile synthesis of Co3O4 nanotubes for supercapacitor materials. In this study, a high specific capacitance of 574 F g−1 at a current density of 0.1 A g−1 was shown, nonetheless a loss of capacitance of about 16% at 1 A g−1 had downplayed its competency at high current densities.18 As for molybdenum oxides, MoO2 nanorods showed a low specific capacitance, however MoO2 is suitable in the application of asymmetric hybrid electrochemical capacitors due to its stable capacitive behavior in wide potential windows.19 As exemplified in the two studies, cobalt oxides possess attractive properties including low cost and high redox activity.20–23 On the other hand, molybdenum oxides demonstrate reversible small ion storage and a wide potential window.24,25 Hence, integrating the advantageous properties of cobalt oxides and molybdenum oxides for the development of binary oxides made from molybdenum and cobalt could lead to enhanced capacitive behaviors.
Despite the attractive advantages of molybdenum and cobalt binary oxides as electrode materials, it is important to design their structures and tune their crystallinity rationally in order to enhance the electrochemical performance further. This is because pseudocapacitance originates from redox faradaic reactions that include the reversible transportation of ions and electrons.26 Moreover, this process involves charge transport inside the electrodes as well as at the interface of the electrode and electrolyte. As such, 1-D and 2-D nanostructures can be easily accessed by electrolytes and can shorten the path for ion transport, eventually leading to larger capacitance and good rate capability.27–31
Intrigued by these results, we worked on building cobalt and molybdenum oxide based 1-D and 2-D materials: CoMoO4 nanowires, nanorods and micro-rhombohedra. They were produced by hydrothermal/solvothermal methods and their uniform structure and excellent electrochemical capacitive behavior are demonstrated in detail. Such findings demonstrate the great prospect of cobalt and molybdenum based binary oxides for high-performance energy storage applications by utilizing the advantages such as low cost, easy scale-up and high long-term performance.
2. Experimental section
2.1 Materials synthesis
The synthesis of cobalt molybdate nanowires proceeded by a typical hydrothermal process: 0.5 mmol Co(NO3)2·6H2O was dissolved in 9 mL DI water, then 0.5 mmol Na2MoO4·2H2O was dissolved in 9 mL DI water, subsequently the Na2MoO4·2H2O solution was mixed with the Co(NO3)2·6H2O solution and the mixture was stirred thoroughly for 10 min. The mixture was transferred into an 80 mL autoclave and heated at 150 °C for 12 h. The products were centrifuged, and washed using distilled water several times and dried at 80 °C, after the autoclave reached room temperature. The synthesis protocol of ultra-small cobalt molybdate nano-rods proceeded via a similar procedure except that 0.5 mmol Co(NO3)2·6H2O was dissolved in 9 mL methanol. For the case of micro-rhombohedra, 0.25 mmol of Co(NO3)2·6H2O was added to 9 mL DI water and mixed with 0.5 mmol Na2MoO4·2H2O in 9 mL DI water, with the reaction being kept at 150 °C for 12 h.
A graphene oxide (G.O) solution was prepared via the modified Hummers' method.6,19 Subsequently 2.5 mL of graphene oxide solution (5 mg mL−1) and 6 mL of de-ionized (D.I) water were mixed. This mixture was stirred and then sonicated to ensure complete mixing and homogenization. The solution was then subjected to a hydrothermal reaction at 180 °C in a 25 mL autoclave for 12 hours.
2.2 Materials characterization
The as-prepared products were studied using a field emission scanning electron microscope (JEOLJSM-6700F microscope) operated at 5 kV. TEM and high resolution TEM (HRTEM) images were obtained using a JEOL JEM-2010 FEF microscope at an accelerating voltage of 200 kV. X-ray diffraction (XRD) was conducted with a Bruker D8 Advance X-ray diffractometer equipped with Cu Kα radiation (λ = 0.15406 nm). Raman tests were carried out using a Renishaw inVia Raman microscope equipped with a charge coupled device (CCD) detector to analyze the chemical bonding states of the products. X-ray photoelectron microscopy (XPS) characterization was performed using a VG ESCALAB 220I-XL system to study surface chemical composition.
2.3 Electrochemical characterization
A CHI660d electrochemical workstation was used to conduct electrochemical tests in a three-electrode cell configuration in 2 M KOH solution. The working electrodes were fabricated by mixing the as-synthesized product, acetylene black and poly(tetrafluoroethylene) in a ratio of 75 wt%
:
20 wt%
:
5 wt%, this mixture was dispersed in ethanol and subsequently dropped onto nickel foam. The working electrodes were dried at 70 °C in a vacuum oven in order to remove the solvent. The loading mass was kept in the range of 1.0–1.2 mg over an area of 1 cm2 of nickel foam. The reference electrode was a standard Ag/AgCl electrode, and the counter electrode was a platinum plate. Cyclic voltammetry (CV) was performed in the potential range from −0.3 to 0.5 V. Galvanostatic charging/discharging measurement was carried out to quantify the specific capacitance in the potential window of 0–0.5 V, based on the following equations.32
2.4 Calculations
For the 3-electrode configuration (half-cell).
From the charge discharge measurements, specific capacitance (F g−1) is calculated from the following formulae: |  | (1) |
| 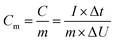 | (2) |
|  | (3) |
|  | (4) |
where C is the specific capacitance (F g−1), I is the current (A), Δt is the discharge time (s), m is the mass of active materials (g), ΔU is the potential window (V), E is the energy density (W h kg−1), and P is the power density (W kg−1).
For the full cell.
For a two-electrode test for the asymmetric supercapacitor (ASC) assembly with a pure graphene negative electrode and the CoMoO4 nanorod positive electrode, the specific capacitance of the entire cell is calculated from eqn (2): | 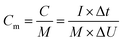 | (5) |
where only M differs from eqn (1) with M = m− + m+, in which m+ and m− are the active masses of the material in the positive and negative electrodes respectively.
The energy density (W h kg−1) and power density (kW kg−1) of the ASC are also calculated using eqn (3) and (4) respectively, which are used for the Ragone plot.
3. Results and discussion
3.1 Characterization of CoMoO4
FESEM images of the obtained CoMoO4 micro/nanostructures are shown in Fig. 1. Fig. 1a and b show pure CoMoO4 nanowires that were synthesized in pure water, the CoMoO4 nanowires have a diameter of 500 nm and length of 10 μm. The bundles of nanowires were loosely composed of slim CoMoO4 filaments, several micrometers in length. Fig. 1c and d display ultra-small CoMoO4 nanorods, which have a diameter of 20 nm and length of 100–200 nm. These nanorods were synthesized in a mixed water–methanol system. It can be observed that some of the nanorods are not smooth. In order to have a closer look at CoMoO4 nanostructures, transmission electron microscopy (TEM) and HRTEM were conducted (Fig. 2). From the image it can be seen that the small nanorods were well dispersed. The HRTEM images (Fig. 2d) show that the nanorods have abundant defects due to vacancies and dislocated atoms, which are more electroactive and may give rise to faster redox reactions; besides, the interlayer distance of CoMoO4 is about 0.335 nm, which coincides with the interplanar distances of CoMoO4.33,34 As a comparison, ultra-small CoMoO4 nanorods can be synthesized in a mixed hydrothermal/solvothermal system. This is probably attributed to the methanol solvent which can coordinate easily to the as-formed particle nuclei, thus allowing stable colloidal suspensions of well dispersed oxide nanoparticles;34,35 therefore, small rod-like structures can be formed. Micro-rhombohedra were fabricated in the pure water system by adjusting the ratio of precursor molecules (cobalt nitrate
:
sodium molybdate) from the molar ratio of 1
:
1 to 1
:
2. The as-synthesized micro-rhombohedron is homogeneous and uniform, with the approximate dimensions (length, width and thickness) of 6 μm, 5 μm and 1 μm, respectively.
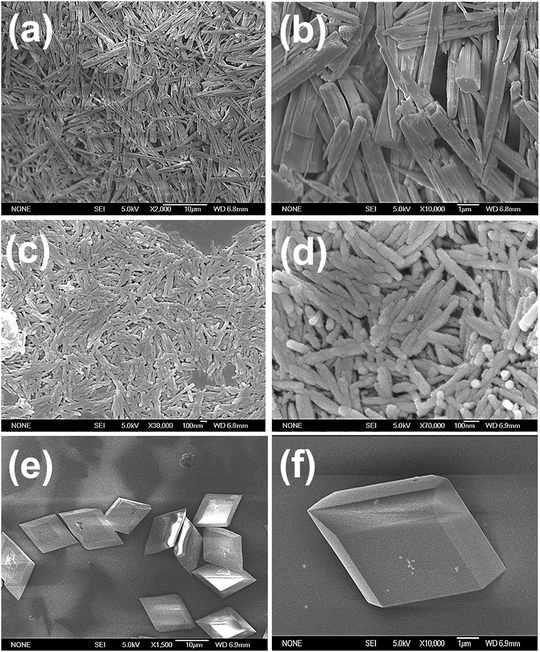 |
| Fig. 1 FESEM images of (a and b) CoMoO4 nanowires, (c and d) CoMoO4 nanorods, (e and f) CoMoO4 micro-rhombohedra. | |
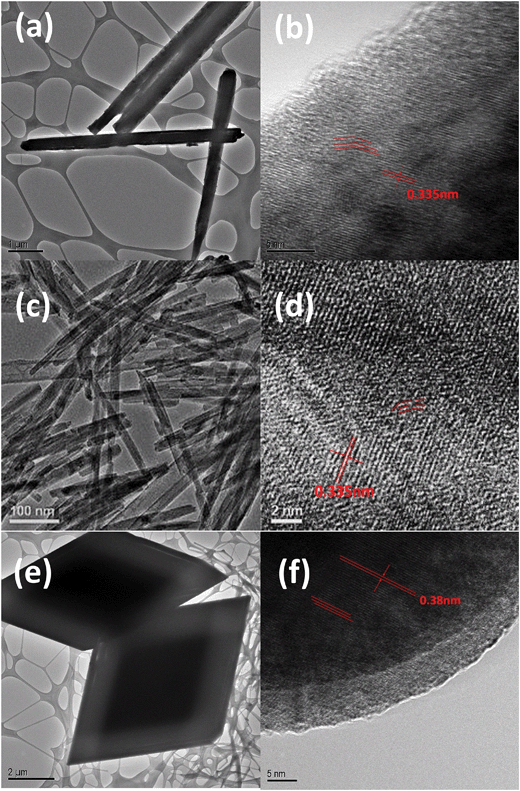 |
| Fig. 2 TEM and HRTEM images of (a and b) CoMoO4 nanowires, (c and d) CoMoO4 nanorods, (e and f) CoMoO4 micro-rhombohedra. | |
X-ray diffraction (XRD) measurements were conducted to determine the crystal structures and phases of the products, which are shown in Fig. 3a. The CoMoO4 nanowires display stronger crystallinity than the nanorods. Micro-rhombohedra on the other hand showed a distinct diffraction pattern which indicated high crystallinity. Their patterns were consistent with the standard pattern for CoMoO4 (JCPDS no. 21-0868), as typical diffraction peaks of CoMoO4 were observed. Thus, based on the measurement, it can be expected that nanorods will outperform the other nanostructures due to their low crystallinity, which was proven from previous study,35,36 as the lower crystallinity of CoMoO4 favors improvement in its electrochemical capacitive properties.
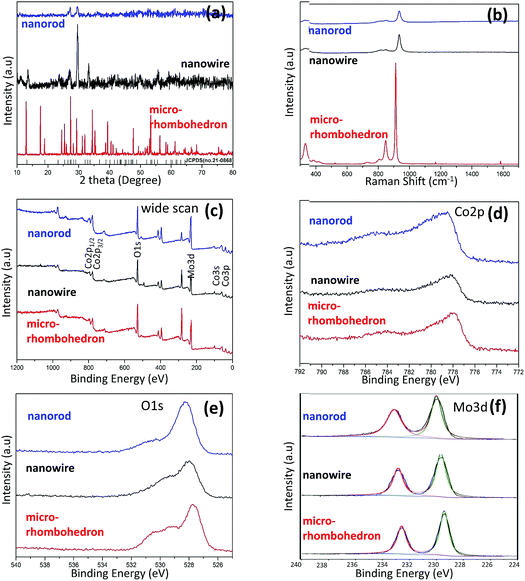 |
| Fig. 3 (a) XRD patterns of CoMoO4 nanowires, nanorods, and micro-rhombohedra; (b) Raman spectra of CoMoO4 nanowires, nanorods, and micro-rhombohedra; (c) XPS survey scan showing the elements Co, O and Mo; (d) XPS of Co 2p; (e) XPS of O 1s; (f) XPS of Mo 3d. | |
Raman spectroscopy was carried out, which is displayed in Fig. 3b. All Raman spectra exhibit peaks at around 332, 849, 879, and 935 cm−1, which were similar to previous reports,36,37 where a dominant band at 940 cm−1, and minor bands at 813 and 880 cm−1 can be identified. The strong bands at 879 and 935 cm−1 and a discernible band at 332 cm−1 correspond to Mo–O–Co stretching vibrations in CoMoO4. Therefore, the main bands of CoMoO4 which have appeared in the Raman spectra indicate the successful synthesis of CoMoO4.
In order to study the surface chemical composition of the CoMoO4 nanostructures, X-ray photoelectron spectroscopy (XPS) characterization was conducted in the binding energy ranging from 0 to 1200 eV (Fig. 3c). The XPS survey spectrum of CoMoO4 (Fig. 3d) exhibited three major elements: Co, Mo, and O. The Co 2p3/2 core-level spectrum is centered at 778.5 eV (Fig. 3d), which corresponds to Co2+.38 The O 1s spectrum (Fig. 3e) has one main peak at 528.3 eV which results from the lattice oxygen.39,40Fig. 2c shows the XPS data in the range of 392–404 eV, where only one peak at 397.7 eV is observed, which is assigned to Mo 3p3/2.41 In the region of 224–240 eV, a 3d5/2–3d3/2 doublet at 233.2 and 230 eV can be identified. The binding energy and the splitting width (ΔMo 3d = 3.2 eV) well fit with those reported for Mo6+.42,43
3.2 Electrochemical performances
Cyclic voltammetry (CV) was conducted to study the charge transport mechanism in the as-prepared electrodes. Fig. 4 shows redox peaks in the CV curves of CoMoO4 in a 2 M KOH solution, showing the pseudo-capacitance behaviors of the faradaic redox reaction of CoMoO4. The faradaic redox reactions proceeded via the following steps:36
3[Co(OH)3]− ↔ Co3O4 + 4H2O + OH− + 2e− |
Co3O4 + H2O + OH− ↔ 3CoOOH + e− |
CoOOH + OH− ↔ CoO2 + H2O + e− |
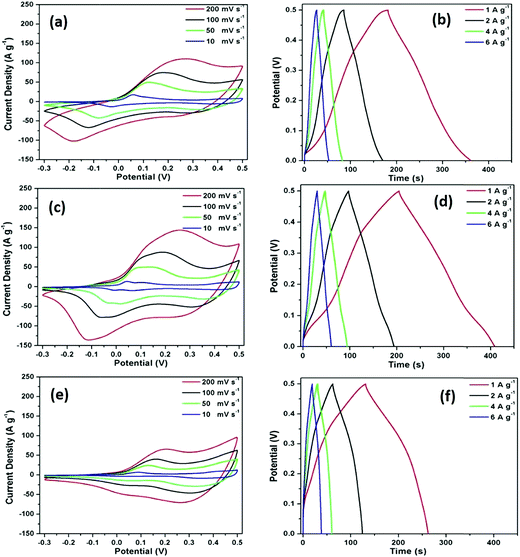 |
| Fig. 4 (a, c and e) Cyclic voltammograms of CoMoO4 nanowires, nanorods, and micro-rhombohedra in 2 M KOH; (b, d and f) galvanostatic charging/discharging curves of CoMoO4 nanowires, nanorods, and micro-rhombohedra in 2 M KOH. | |
Moreover, the scan rates of CV were increased in order to probe their corresponding current response. The scan rates were set as 10 mV s−1, 50 mV s−1, 100 mV s−1 and 200 mV s−1, and the redox current increased accordingly. Besides, the anodic peak moved to a positive potential and the cathodic peak moved to a negative potential as the scan rate increased. The proportional increase of the peak current with scan rate can also demonstrate the pseudocapacitive behavior of the as-prepared electrodes. Furthermore, the corresponding increase of current at the high scan rate confirms that the rates of redox faradaic reactions and charge transport are sufficient to work at high current densities, which further indicates the superiority of the as-prepared electrodes.
The galvanostatic charging/discharging (GCD) curves of CoMoO4 electrodes were recorded in 2 M KOH at room temperature (Fig. 4b, d and f). The specific capacitances of the three electrodes were calculated based on the discharging curves, as shown in Fig. 5c. The specific capacitance of CoMoO4 nanorods, nanowires and micro-rhombohedra electrodes were calculated as: 420, 379, 365, 360 F g−1; 362, 340, 322, 312 F g−1; and 263, 250, 248, 238 F g−1 at 1, 2, 4, and 6 A g−1. It is noteworthy that the specific capacitance of the CoMoO4 nanorods is much higher than that of the other two electrodes.
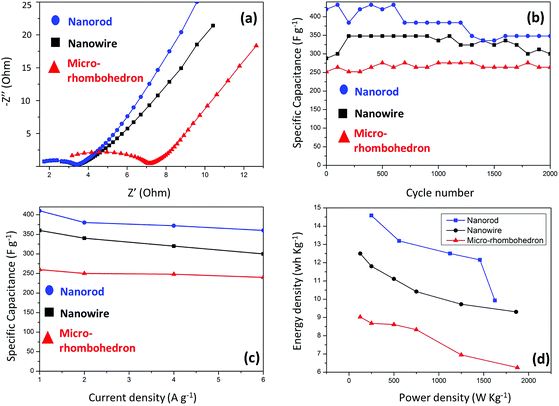 |
| Fig. 5 (a) Complex-plane impedance plots of CoMoO4 nanowires, nanorods, and micro-rhombohedra; (b) cycle life of CoMoO4 nanowires, nanorods, and micro-rhombohedra at a current density of 6 A g−1; (c) specific capacitance of CoMoO4 nanowires, nanorods, and micro-rhombohedra at various current densities; (d) energy density vs. power density of CoMoO4 nanowires, nanorods, and micro-rhombohedra. | |
However, high current densities of electrochemical capacitors are strongly desired in industrial applications. As such, measurements of the specific capacitance of Co–MoO4 electrodes at higher current densities are needed, which was emphasized in this work. Normally at high current densities, the inner active sites of the electrode cannot be fully accessed because of the high drop in internal resistance, correspondingly specific capacitance values may drop largely.44 Our result reveals that the observed specific capacitance value of 360 F g−1 at 6 A g−1 for the CoMoO4 electrodes is higher than those of previous reports, such as for MoO2 (ref. 45) and Co3O4.46 For CoMoO4 nanorods, at a current density of 6 A g−1 nearly 85% of the specific capacitance remains compared to that at 1 A g−1.
A comparison of the specific capacitance and rate capability of the CoMoO4 nanowires, nanorods, and micro-rhombohedra is summarized in Fig. 5c. The GCD curves are symmetrical, which shows their high coulombic efficiency and good reversibility in redox faradaic reactions. Although the specific capacitance inevitably drops when the current density increases, the CoMoO4 nanorod electrode possesses the highest capacitance and good rate capability all the way. This is possibly due to the superiority of the one-dimensional structure and ultra-small size of the Co–MoO4 nanorods, which allows electrolyte ions to move freely inside the electrode materials, the rate capability of the nanorods thus being enhanced significantly. The GCD cycle test was conducted for 2000 cycles in order to compare the long term performance of the CoMoO4 electrodes (Fig. 5b). It was observed that after 2000 cycles, the CoMoO4 nanorods still have high specific capacitance among the three electrodes. Compared with our CoMoO4 electrochemical capacitors, Portet et al. reported an activated carbon/carbon nanotube capacitor with a specific capacitance of 93 F g−1, and the activated carbon based capacitor showed a drastic decrease in capacitance at high current densities.47 On the other hand, Fe3O4/activated carbon reported by Du et al. delivered a specific capacitance of 37.9 F g−1, and lost 18% of the initial capacity after 500 cycles.48 Therefore, these results show that our CoMoO4 based electrochemical capacitor has demonstrated great improvement in its capacitance and stability performance.
Electrochemical performance depends on the particle size, crystal structure and surface area. Thus in our work the energy capacitive capabilities may be related to the nanoscale architecture of CoMoO4 electrodes. As the nanorods were homogeneous and well-dispersed, this morphology may largely alleviate self-aggregation of the CoMoO4 nanorods, which guarantees effective and sufficient contact of the CoMoO4 nanorod surface with electrolytes.49,50 Besides, smaller and shorter rod-like CoMoO4 that displays low crystallinity provides sufficient surface active sites for the redox reaction.35 Therefore, the facile reactivity of OH− is an additional factor contributing to the improved charge storage. In total, these factors may lead to the improvement of capacitive behaviors as electrochemical capacitors. As for comparisons in the nanoscale architecture of CoMoO4, Liu et al. prepared CoMoO4 nanorod electrodes for electrochemical capacitors; the nanorods were highly crystalline with a diameter of about 100 nm, delivering a specific capacitance of 286 F g−1.51 Geng et al. prepared CoMoO4 microplates@CoMoO4 microprisms on Ni foam. They displayed superior cycling stability, but showed significant drops at high current densities.52 From these comparisons it is obvious that our morphology-controlled ultra-small nanorods have displayed significant enhancement in their capacitive behaviors.
Electrochemical impedance spectroscopy (EIS) was conducted to test electrode resistance and electrolyte diffusion (Fig. 5a). As demonstrated by previous impedance studies on cobalt oxide nanostructures, a typical EIS curve is composed of two main parts: (1) the semicircular loop at high frequencies, which stands for the resistance of charge transfer; such a property is closely related to the surface atomic arrangements of the electrodes, with the lower value of the resistance that indicates good conductive nature; (2) straight lines at lower frequencies that represent electrolyte diffusion properties. As a whole, fast ion transport from the electrolyte to the electrode surface improves its capacitive performance.53,54 The diameters of the semicircular loops of the EIS curves for the nanowire, nanorod and microrhombohedron electrodes are about 1.8, 1.7, and 4.0 Ω, respectively. This clearly illustrates that the nanorod structures have a faster redox faradaic reaction and the best charge transport property among all the structures, which may benefit from the low crystalline nanostructures, as evidenced by the aforementioned results of TEM, HRTEM and XRD. Moreover, the slope of the straight segment of the micro-rhombohedron electrode is less than 45°, indicating that the material may not store electrochemical energy efficiently. In contrast, the slope of nanorods in the lower frequency region is the highest among the three electrodes, implying that ultra-small rod-like structures can result in better electrolyte diffusion, which may be another factor contributing to the improvement in the capacitive behavior.
Fig. 5d shows the plot of energy density vs. power density of these electrodes. Compared with the present work's nanowires and micro-rhombohedra, the nanorods have better specific energy and power densities. Furthermore, the decrease of energy density for the Co–MoO4 nanorods with the increase in power density is much smaller than that of the CoMoO4 nanowires, micro-rhombohedra and the electrodes of previous studies. The highest energy density 14.6 W h kg−1 at a power density of 250 W kg−1 was recorded for the case of nanorods.
As CoMoO4 nanorods show excellent electrochemical performance, their capacitive behaviors were characterized by studying the voltammetric response at various scan rates (Fig. 6). In the cyclic voltammetry studies, the relationship between current response and scan rate can be expressed using the power law i = aνb (ν is the scan rate, a and b are coefficient parameters),55 The b value can be calculated from the slope of the linear plot of log
i vs. log
ν (Fig. 6a). Generally, when b = 1, the electrochemical reaction is related to a nondiffusion-controlled surface redox reaction; when b = 1/2, the electrochemical reaction is related to the ideal diffusion-controlled faradaic process.56 The calculated b values of the CoMoO4 electrode at different potentials are shown in Fig. 6b. At the potential of 0.05–0.15 V, the b value is in the range of 0.6–0.8, indicating that the current arises from the contribution of both capacitive effects and diffusion-controlled behavior. As verified from the HRTEM image, the interplanar distance of the nanorod is 0.335 nm, which allows the diffusion paths for OH− (0.274 nm) to intercalate.
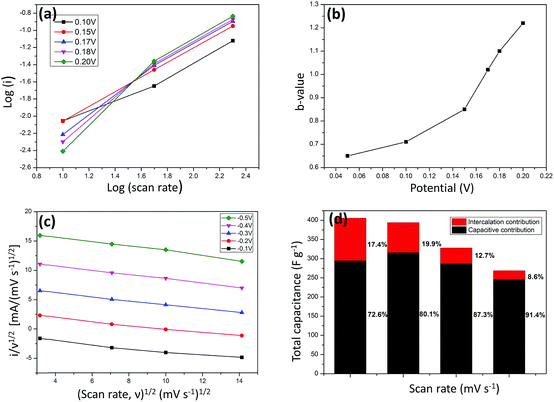 |
| Fig. 6 (a) Power law dependence of current on the scan rate; (b) b values at various potentials for the anodic scan; (c) plots of ν1/2versus i(V)/ν1/2 used for calculating k1 and k2 at different potentials of the cathodic scan; (d) bar chart of total capacitance with the percentage of capacitive contribution and intercalation contribution at various scan rates. | |
Moreover, we further quantify the contribution fraction of capacitive and intercalation effects, respectively. Because the current response (mA) at a specified potential (V) is the sum of two current fractions incurred by the capacitive effects and intercalation behavior as mentioned above, the following equation was adopted:
where
a1ν and
a2ν1/2 denote the current responses from the capacitive and intercalation processes, respectively. Thus, it is possible to quantify the current contribution fraction originating from capacitive and intercalation processes at a given potential by calculating
a1 and
a2 by plotting
ν1/2versus i(
V)/
ν1/2 as
Fig. 6c shows. It was found that an increase in scan rate has a minor effect on the capacitive contributions, while the intercalation contribution decreases markedly with the increase of scan rate (
Fig. 6d). Since the intercalation process is diffusion-controlled, the time for electrolyte diffusion into the host lattices will decrease upon increasing the scan rate. Hence less electrolyte will be involved and its contribution will decrease accordingly.
56 Therefore, the redox pseudocapacitive behavior will dominate the charge storage at high scan rates.
To measure the performances of CoMoO4 nanorods in practical applications, a simple asymmetric electrochemical capacitor was assembled by using CoMoO4 nanorods as the positive electrode and pure graphene hydrogel as the negative electrode in a two-electrode configuration in a 2 M KOH solution. The pure graphene electrode is known to act as a good negative electrode in aqueous alkaline medium and was also tested for both GCD and CV measurements within a potential window of 0 to −1 V in 2 M KOH as shown in Fig. 8a and b.32,57 Before assembling the full cell, it was probed that the graphene hydrogel can work under the potential window from −1 V to 0 V (Fig. 7a and b), while the operation window of CoMoO4 nanorods was 0–0.5 V as shown in Fig. 4f. It was assumed that the full cell can be cycled reversibly in the potential window of 0–1.5 V. Therefore, the full cell was assembled and tested in the potential window from 0 V to 1.5 V. Our pure graphene hydrogel gave a high specific capacitance of 315 F g−1 at 1 A g−1 and showed good rate capability by retaining a capacitance of 230 F g−1 even at 10 A g−1 as seen in Fig. 7c. The pure graphene hydrogel thus proves to be far more superior as a negative electrode, compared to the conventional activated carbon. The possibility of using it directly without any binders, its high capacitance, chemical stability and durability, all make it suitable for use as a negative electrode in the full cell.
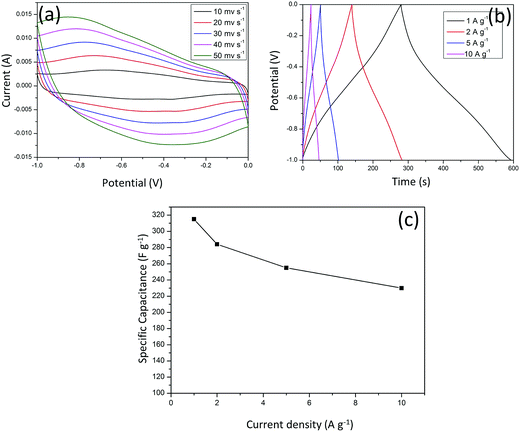 |
| Fig. 7 (a) CV graphs; (b) GCD plot; (c) specific capacitance vs. current density; all of pure graphene hydrogel (negative electrode) in 2 M KOH electrolyte. | |
To balance the charge storage in the full cell, the masses of the positive electrode and the negative electrode with different specific capacitances and working voltage were adjusted according to eqn (6):57
|  | (6) |
where
m is the mass,
C is the specific capacitance at a current density of 1 A g
−1, and Δ
V is the voltage range.
The ASC was then assembled accordingly and tested for GCD and CV as seen in Fig. 8a and b. All the charge/discharge curves are symmetric, which exhibit their superior reversibility and significance for use in future practical applications. The specific capacitances were calculated from the GCD curves and gave a high value of 57 F g−1 at 1 A g−1. The cycle test showed that the full cell reached the high specific capacitance and maintained a stable charge storage behavior without much decay in the testing period as shown in Fig. 8c.
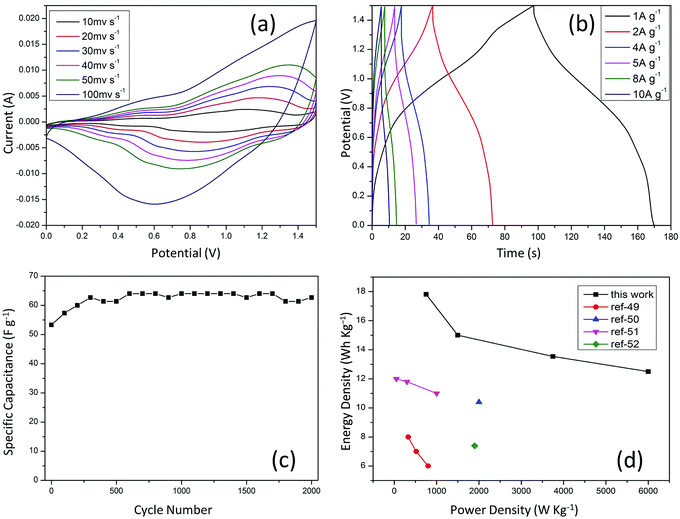 |
| Fig. 8 (a) CV plot of the ASC CoMoO4//pure G; (b) GCD graph of the ASC CoMoO4//pure G; (c) cyclic stability of the ASC CoMoO4//pure G, all in 2 M KOH electrolyte; (d) Ragone plot of our CoMoO4 nanorods//pure G and some devices from previous reports. | |
The Ragone plot is depicted in Fig. 8d including the energy density and power density obtained from GCD and a comparison is drawn between our material and some of the existing materials. Our ASC shows marked improvements in energy and power densities. Even a simple assembly of our CoMoO4 nanorods//pure graphene gave a specific energy density of 12.5 W h kg−1 at a power density of 6000 W kg−1. This opens new doors for assembling practical asymmetric capacitor systems with our material as a positive electrode and with a 3-D well dispersed and macroscopic graphene hydrogel as the negative electrode to improve the overall performance. A few comparisons have been made to some other transition metal oxides based electrochemical full cells. The graphene–MnO2//graphene asymmetric device could give an energy density of 10.03 W h kg−1 at an average power density of 2.53 kW kg−1.58 The MnO2-functional CNT (FCNT)//FCNT asymmetric device showed energy density of 10.4 W h kg−1 at 2000 W kg−1.59 For the full-cell made with RuO2//RuO2, when its power density is larger than 1000 W kg−1, the energy density decreases fast and becomes 1.3 W h kg−1 at 5000 W kg−1.7 As a counterpart of a CoMoO4 based asymmetric electrochemical capacitor, the Ni–Co oxide//AC asymmetric device displayed the energy density of 7.4 W h kg−1 at 1900 W kg−1.60 The rGO//CoMoO4 ASC showed a specific capacitance of 26.16 F g−1, with an energy density of 8.17 W h kg−1.61 To the best of our knowledge, this is the first time that CoMoO4 based materials were assembled in a full cell and showed much improved performance, compared with other intensively studied transition metal oxides based full cells. These comparisons demonstrate the improvements that we have achieved in the electrochemical performance and may pave a new way to broaden the application of transition metal oxides based electrochemical capacitors.
4. Conclusion
In summary, a mixed hydrothermal/solvothermal method was developed to synthesize one-dimensional Co–MoO4 nanorods possessing a specific capacitance of 420 F g−1 at the current density of 1 A g−1, with a good rate capability with capacitance retention of 85% at a high current density of 6 A g−1. The superior supercapacitive behavior could be attributed to the unique one dimensional structure of CoMoO4 nanorods that have a large electroactive surface and low crystal degree, which facilitates electrolyte diffusion, charge transport and fast faradaic reaction. Moreover, an asymmetric electrochemical capacitor was assembled by using the CoMoO4 nanorods and graphene hydrogel. It exhibited high power density of 6000 W kg−1 with energy density of 12.5 W h kg−1. This work demonstrates that constructing one-dimensional CoMoO4 nanorods by controlling the morphology and crystallinity is a simple and effective way to enhance electrochemical capacitive performances. The synthesis strategy developed in this work may open a new avenue to produce novel materials for energy storage devices.
Conflict of interest
The authors declare no competing financial interest.
Acknowledgements
This work is supported by the AcRF Tier 1 grant (RGT27/13), Ministry of Education, Singapore.
References
- J. R. Miller, R. A. Outlaw and B. C. Holloway, Graphene Double-Layer Capacitor with AC Line-Filtering Performance, Science, 2010, 329, 1637–1639 CrossRef CAS PubMed.
- P. C. Chen, G. Z. Shen, Y. Shi, H. T. Chen and C. W. Zhou, Preparation and Characterization of Flexible Asymmetric Supercapacitors Based on Transition-Metal-Oxide Nanowire/Single-Walled Carbon Nano-tube Hybrid Thin-Film Electrodes, ACS Nano, 2010, 4, 4403–4411 CrossRef CAS PubMed.
- T. Brezesinski, J. Wang, S. H. Tolbert and B. Dunn, Ordered mesoporous alpha-MoO3 with iso-oriented nanocrystalline walls for thin-film pseudocapacitors, Nat. Mater., 2010, 9, 146–151 CrossRef CAS PubMed.
- P. Simon and Y. Gogotsi, Materials for electrochemical capacitors, Nat. Mater., 2008, 7, 845–854 CrossRef CAS PubMed.
- P. J. Hall and E. J. Bain, Energy-storage technologies and electricity generation, Energy Policy, 2008, 36, 4352–4355 CrossRef.
- P. Simon and Y. Gogotsi, Capacitive Energy Storage in Nanostructured Carbon–Electrolyte Systems, Acc. Chem. Res., 2013, 46, 1094–1103 CrossRef CAS PubMed.
- Z. S. Wu, D. W. Wang, W. Ren, J. Zhao, G. Zhou, F. Li and H. M. Cheng, Anchoring hydrous RuO2 on graphene sheets for high-performance electrochemical capacitors, Adv. Funct. Mater., 2010, 20, 3595–3602 CrossRef CAS.
- Z. N. Yu, B. Duong, D. Abbitt and J. Thomas, Highly Ordered MnO2 Nanopillars for Enhanced Supercapacitor Performance, Adv. Mater., 2013, 25, 3302–3306 CrossRef CAS PubMed.
- B. Wang, T. Zhu, H. B. Wu, R. Xu, J. S. Chen and X. W. D. Lou, Porous Co3O4 nanowires derived from long Co(CO3)0.5(OH)·0.11H2O nanowires with improved supercapacitive properties, Nanoscale, 2012, 4, 2145–2149 RSC.
- T. Y. Wei, C. H. Chen, H. C. Chien, S. Y. Lu and C. C. Hu, A cost-effective supercapacitor material of ultrahigh specific capacitances: spinel nickel cobaltite aerogels from an epoxide-driven sol–gel process, Adv. Mater., 2010, 22, 347–351 CrossRef CAS PubMed.
- J. W. Lang, L. B. Kong, W. J. Wu, Y. C. Luo and L. Kang, Facile approach to prepare loose-packed NiO nano-flakes materials for supercapacitors, Chem. Commun., 2008, 4213–4215 RSC.
- J. W. Lang, L. B. Kong, M. Liu, Y. C. Luo and L. Kang, Co0.56Ni0.44 Oxide Nanoflake Materials and Activated Carbon for Asymmetric Supercapacitor, J. Electrochem. Soc., 2010, 157, A1341–A1346 CrossRef CAS.
- Y. Q. Wu, X. Y. Chen, P. T. Ji and Q. Q. Zhou, Sol–gel approach for controllable synthesis and electrochemical properties of NiCo2O4 crystals as electrode materials for application in supercapacitors, Electrochim. Acta, 2011, 56, 7517–7522 CrossRef CAS.
- G. Gao, H. B. Wu, S. Ding, L. M. Liu and X. W. D. Lou, Hierarchical NiCo2O4 Nanosheets Grown on Ni Nanofoam as High-Performance Electrodes for Supercapacitors, Small, 2015, 11, 804–808 CrossRef CAS PubMed.
- J. K. Chang, M. T. Lee, C. H. Huang and W. T. Tsai, Physicochemical properties and electrochemical behavior of binary manganese–cobalt oxide electrodes for supercapacitor applications, Mater. Chem. Phys., 2008, 108, 124–131 CrossRef CAS.
- M. Jayalakshmi and K. Balasubramanian, Simple Capacitors to Supercapacitors – An Overview, Int. J. Electrochem. Sci., 2008, 3, 1196–1217 CAS.
- C. C. Hu and C. C. Wang, Improving the utilization of ruthenium oxide within thick carbon–ruthenium oxide composites by annealing and anodizing for electrochemical supercapacitors, Electrochem. Commun., 2002, 4, 554–559 CrossRef CAS.
- J. A. Xu, L. Gao, J. Y. Cao, W. C. Wang and Z. D. Chen, Preparation and electrochemical capacitance of cobalt oxide (Co3O4) nanotubes as supercapacitor material, Electrochim. Acta, 2010, 56, 732–736 CrossRef CAS.
- L. Zheng, Y. Xu, D. Jin and Y. Xie, Well-aligned molybdenum oxide nanorods on metal substrates: solution-based synthesis and their electrochemical capacitor application, J. Mater. Chem., 2010, 20, 7135–7143 RSC.
- X. H. Xia, J. P. Tu, Y. J. Mai, X. L. Wang, C. D. Gu and X. B. Zhao, Self-supported hydrothermal synthesized hollow Co3O4 nanowire arrays with high supercapacitor capacitance, J. Mater. Chem., 2011, 21, 9319–9325 RSC.
- Y. Fan, H. Shao, J. Wang, L. Liu, J. Zhang and C. Cao, Synthesis of foam-like freestanding Co3O4 nanosheets with enhanced electrochemical activities, Chem. Commun., 2011, 47, 3469–3471 RSC.
- W. Zhou, J. Liu, T. Chen, K. S. Tan, X. Jia, Z. Luo, C. Cong, H. Yang, C. M. Li and T. Yu, Fabrication of Co3O4–reduced graphene oxide scrolls for high-performance supercapacitor electrodes, Phys. Chem. Chem. Phys., 2011, 13, 14462–14465 RSC.
- D. W. Wang, Q. H. Wang and T. M. Wang, Morphology-Controllable Synthesis of Cobalt Oxalates and Their Conversion to Mesoporous Co3O4 Nanostructures for Application in Supercapacitors, Inorg. Chem., 2011, 50, 6482–6492 CrossRef CAS PubMed.
- H. Farsi, F. Gobal, H. Raissi and S. Moghiminia, The pH effects on the capacitive behavior of nanostructured molybdenum oxide, J. Solid State Electrochem., 2010, 14, 681–686 CrossRef CAS.
- W. Tang, L. L. Liu, S. Tian, L. Li, Y. B. Yue, Y. P. Wu and K. Zhu, Aqueous supercapacitors of high energy density based on MoO3 nanoplates as anode material, Chem. Commun., 2011, 47, 10058–10060 RSC.
- Y. F. Yuan, X. H. Xia, J. B. Wu, X. H. Huang, Y. B. Pei, J. L. Yang and S. Y. Guo, Hierarchically porous Co3O4 film with mesoporous walls prepared via liquid crystalline template for supercapacitor application, Electrochem. Commun., 2011, 13, 1123–1126 CrossRef CAS.
- T. Xiao, B. J. Heng, X. Y. Hu and Y. W. Tang,
In Situ CVD Synthesis of Wrinkled Scale-Like Carbon Arrays on ZnO Template and Their Use to Supercapacitors, J. Phys. Chem. C, 2011, 115, 25155–25159 CAS.
- O. N. Kalugin, V. V. Chaban, V. V. Loskutov and O. V. Prezhdo, Uniform diffusion of acetonitrile inside carbon nanotubes favors supercapacitor performance, Nano Lett., 2008, 8, 2126–2130 CrossRef CAS PubMed.
- J. A. Yan, E. Khoo, A. Sumboja and P. S. Lee, Facile Coating of Manganese Oxide on Tin Oxide Nanowires with High-Performance Capacitive Behavior, ACS Nano, 2010, 4, 4247–4255 CrossRef CAS PubMed.
- R. Liu and S. B. Lee, MnO2/poly(3,4-ethylenedioxythiophene) coaxial nanowires by one-step co-electrodeposition for electrochemical energy storage, J. Am. Chem. Soc., 2008, 130, 2942–2943 CrossRef CAS PubMed.
- Y. B. He, G. R. Li, Z. L. Wang, C. Y. Su and Y. X. Tong, Single-crystal ZnO nanorod/amorphous and nanoporous metal oxide shell composites: controllable electrochemical synthesis and enhanced super-capacitor performances, Energy Environ. Sci., 2011, 4, 1288–1292 CAS.
- R. H. Wang, C. H. Xu and J. M. Lee, High performance asymmetric supercapacitors: new NiOOH nanosheet/graphene hydrogels and pure graphene hydrogels, Nano Energy, 2016, 19, 210–221 CrossRef CAS.
- A. X. Yin, X. Q. Min, W. Zhu, H. S. Wu, Y. W. Zhang and C. H. Yan, Multiply twinned Pt–Pd nanoicosahedrons as highly active electrocatalysts for methanol oxidation, Chem. Commun., 2012, 48, 543–545 RSC.
- L. Q. Mai, F. Yang, Y. L. Zhao, X. Xu, L. Xu and Y. Z. Luo, Hierarchical MnMoO4/CoMoO4 hetero-structured nanowires with enhanced supercapacitor performance, Nat. Commun., 2011, 2, 381 CrossRef PubMed.
- H. Dong, Y. C. Chen and C. Feldmann, Polyol synthesis of nanoparticles: status and options regarding metals, oxides, chalcogenides, and non-metal elements, Green Chem., 2015, 17, 4107–4132 RSC.
- M.-C. Liu, L.-B. Kong, X.-J. Ma, C. Lu, X.-M. Li, Y.-C. Luo and L. Kang, Hydrothermal process for the fabrication of CoMoO4·0.9H2O nanorods with excellent electrochemical behavior, New J. Chem., 2012, 36, 1713–1716 RSC.
- P. Villa, F. Trifiro and I. Pasquon, Study of the interaction between CoMoO4 and 341-1341-1341-1-Al2O3 by Raman spectroscopy, React. Kinet. Catal. Lett., 1974, 1, 341–344 CrossRef CAS.
- J. M. Ko, D. Soundarajan, J. H. Park, S. D. Yang, S. W. Kim, K. M. Kim and K. H. Yu, Gamma-ray-induced synthesis and electrochemical properties of a mesoporous layer-structured alpha-Co(OH)(2) for supercapacitor applications, Curr. Appl. Phys., 2012, 12, 341–345 CrossRef.
- Z. S. Wu, W. C. Ren, L. Wen, L. B. Gao, J. P. Zhao, Z. P. Chen, G. M. Zhou, F. Li and H. M. Cheng, Graphene Anchored with Co3O4 Nanoparticles as Anode of Lithium Ion Batteries with Enhanced Reversible Capacity and Cyclic Performance, ACS Nano, 2010, 4, 3187–3194 CrossRef CAS PubMed.
- J. Yan, Z. J. Fan, W. Sun, G. Q. Ning, T. Wei, Q. Zhang, R. F. Zhang, L. J. Zhi and F. Wei, Advanced Asymmetric Supercapacitors Based on Ni(OH)2/Graphene and Porous Graphene Electrodes with High Energy Density, Adv. Funct. Mater., 2012, 22, 2632–2641 CrossRef CAS.
- X. F. Xia, Q. L. Hao, W. Lei, W. J. Wang, H. L. Wang and X. Wang, Reduced-graphene oxide/molybdenum oxide/polyaniline ternary composite for high energy density supercapacitors: synthesis and properties, J. Mater. Chem., 2012, 22, 8314–8320 RSC.
- S. N. Wang, Q. S. Gao, Y. H. Zhang, J. Gao, X. H. Sun and Y. Tang, Controllable Synthesis of Organic–Inorganic Hybrid MoOx/Polyaniline Nanowires and Nanotubes, Chem.–Eur. J., 2011, 17, 1465–1472 CrossRef CAS PubMed.
- L. Zheng, Y. Xu, D. Jin and Y. Xie, Polyaniline-Intercalated Molybdenum Oxide Nanocomposites: Simultaneous Synthesis and their Enhanced Application for Supercapacitor, Chem.–Asian J., 2011, 6, 1505–1514 CrossRef CAS PubMed.
- T. Morishita, Y. Soneda, H. Hatori and M. Inagaki, Carbon-coated tungsten and molybdenum carbides for electrode of electrochemical capacitor, Electrochim. Acta, 2007, 52, 2478–2484 CrossRef CAS.
- J. Rajeswari, P. S. Kishore, B. Viswanathan and T. K. Varadarajan, One-dimensional MoO2 nanorods for supercapacitor applications, Electrochem. Commun., 2009, 11, 572–575 CrossRef CAS.
- V. R. Shinde, S. B. Mahadik, T. P. Gujar and C. D. Lokhande, Supercapacitive cobalt oxide (Co3O4) thin films by spray pyrolysis, Appl. Surf. Sci., 2006, 252, 7487–7492 CrossRef CAS.
- C. Portet, P. L. Taberna, P. Simon, E. Flahaut and C. Laberty-Robert, High power density electrodes for carbon supercapacitor applications, Electrochim. Acta, 2005, 50, 4174–4181 CrossRef CAS.
- X. Du, C. Y. Wang, M. M. Chen, Y. Jiao and J. Wang, Electrochemical Performances of Nanoparticle Fe3O4/Activated Carbon Supercapacitor Using KOH Electrolyte Solution, J. Phys. Chem. C, 2009, 113, 2643–2646 CAS.
- G. Q. Zhang, H. B. Wu, H. E. Hoster, M. B. Chan-Park and X. W. Lou, Single-crystalline NiCo2O4 nanoneedle arrays grown on conductive substrates as binder-free electrodes for high-performance supercapacitors, Energy Environ. Sci., 2012, 5, 9453–9456 CAS.
- Z. Q. Liu, K. Xiao, Q. Z. Xu, N. Li, Y. Z. Su, H. J. Wang and S. Chen, Fabrication of hierarchical flower-like super-structures consisting of porous NiCo2O4 nanosheets and their electrochemical and magnetic properties, RSC Adv., 2013, 3, 4372–4380 RSC.
- M. C. Liu, L. B. Kong, C. Lu, X. M. Li, Y. C. Luo and L. Kang, Facile fabrication of CoMoO4 nanorods as electrode material for electrochemical capacitors, Mater. Lett., 2013, 94, 197–200 CrossRef CAS.
- X. W. Geng, Y. F. Ai and G. Z. Shen, Facile construction of novel CoMoO4 microplates@CoMoO4 microprisms structures for well-stable supercapacitors, Prog. Nat. Sci.: Mater. Int., 2016, 26, 243–252 CrossRef CAS.
- H. W. Wang, Z. A. Hu, Y. Q. Chang, Y. L. Chen, Z. Q. Lei, Z. Y. Zhang and Y. Y. Yang, Facile solvothermal synthesis of a graphene nanosheet–bismuth oxide composite and its electrochemical characteristics, Electrochim. Acta, 2010, 55, 8974–8980 CrossRef CAS.
- J. Yan, T. Wei, W. M. Qiao, B. Shao, Q. K. Zhao, L. J. Zhang and Z. J. Fan, Rapid microwave-assisted synthesis of graphene nanosheet/Co3O4 composite for supercapacitors, Electrochim. Acta, 2010, 55, 6973–6978 CrossRef CAS.
- V. Augustyn, P. Simon and B. Dunn, Pseudo-capacitive oxide materials for high-rate electrochemical energy storage, Energy Environ. Sci., 2014, 7, 1597–1614 CAS.
- M. Sathiya, A. S. Prakash, K. Ramesha, J. M. Tarascon and A. K. Shukla, V2O5-Anchored Carbon Nanotubes for Enhanced Electrochemical Energy Storage, J. Am. Chem. Soc., 2011, 133, 16291–16299 CrossRef CAS PubMed.
- F. Luan, G. M. Wang, Y. C. Ling, X. H. Lu, H. Y. Wang, Y. X. Tong, X. X. Liu and Y. Li, High energy density asymmetric supercapacitors with a nickel oxide nanoflake cathode and a 3D reduced graphene oxide anode, Nanoscale, 2013, 5, 7984–7990 RSC.
- L. J. Deng, G. Zhu, J. F. Wang, L. P. Kang, Z. H. Liu, Z. P. Yang and Z. L. Wang, Graphene–MnO2 and graphene asymmetrical electrochemical capacitor with a high energy density in aqueous electrolyte, J. Power Sources, 2011, 196, 10782–10787 CrossRef CAS.
- H. Jiang, C. Z. Li, T. Sun and J. Ma, A green and high energy density asymmetric supercapacitor based on ultrathin MnO2 nanostructures and functional mesoporous carbon nanotube electrodes, Nanoscale, 2012, 4, 807–812 RSC.
- C. H. Tang, Z. Tang and H. Gong, Hierarchically Porous Ni–Co Oxide for High Reversibility Asymmetric Full-Cell Supercapacitors, J. Electrochem. Soc., 2012, 159, A651–A656 CrossRef CAS.
- G. K. Veerasubramani, K. Krishnamoorthy and S. J. Kim, Electrochemical performance of an asymmetric supercapacitor based on graphene and cobalt molybdate electrodes, RSC Adv., 2015, 5, 16319–16327 RSC.
Footnote |
† D. T. Dam and T. Huang contributed equally to this work. |
|
This journal is © The Royal Society of Chemistry 2017 |
Click here to see how this site uses Cookies. View our privacy policy here.