DOI:
10.1039/C7FO01453H
(Paper)
Food Funct., 2018,
9, 525-533
Dietary compound proanthocyanidins from Chinese bayberry (Myrica rubra Sieb. et Zucc.) leaves attenuate chemotherapy-resistant ovarian cancer stem cell traits via targeting the Wnt/β-catenin signaling pathway and inducing G1 cell cycle arrest
Received
19th September 2017
, Accepted 3rd December 2017
First published on 4th December 2017
Abstract
Cancer stem cells (CSCs) represent a small population of cancer cells characterized by self-renewal ability, tumorigenesis and drug resistance. Ovarian cancer is one of the leading causes of death related to the female reproductive system in Western countries and has been evaluated as a type of CSC-related cancer in recent years. Natural products have attracted great attention in cancer treatment in recent years due to drug resistance and a high relapse rate of ovarian cancer. Chinese bayberry leaf proanthocyanidins (BLPs) contain epigallocatechin-3-O-gallate as their terminal and major extension units, which is quite unusual in the plant kingdom. BLPs showed strong antioxidant and antiproliferative abilities in previous studies. In the present study, chemotherapy-resistant OVCAR-3 spheroid (SP) cells were obtained by sphere culturing and exhibited CSC-like properties by showing a higher ALDH+ population and higher expression of stemness-related proteins. BLPs exhibited inhibitory effects on the growth and CSC characteristics of OVCAR-3 SP cells by showing decreased cell viability, sphere and colony formation ability, ALDH+ population and expression of stemness-related proteins. BLPs also targeted the Wnt/β-catenin pathway by reducing the expression of β-catenin, cyclin D1 and c-Myc and thus inhibited the self-renewal ability of OVCAR-3 SP cells. Furthermore, BLPs also induced G1 cell cycle arrest in OVCAR-3 SP cells. Taken together, these findings suggested that BLPs may be an important agent in the development of therapeutics for ovarian cancer patients.
1. Introduction
Ovarian cancer is the most lethal disease among all gynecologic malignancies and the fifth leading cause of cancer deaths in women. Ovarian cancer patients might respond to chemotherapy in the initial stage. However, the 5-year survival is less than 30% due to late-stage diagnosis, disease recurrence and resistance to standard platinum-based chemotherapy.1 Cancer stem cells (CSCs) are proposed to be one of the reasons for disease relapse and drug resistance. CSCs, also known as tumor-initiating cells, represent a small population of cells that have self-renewal and tumor initiative properties in vivo.2 Due to the limited number within the bulk of tumor cells and properties similar to normal stem cells, CSCs are more resistant to DNA damage induced cell death and therefore are more tolerant to traditional chemotherapy than the majority of other cancer cells.3 CSCs can be enriched in sphere cultures. In 2008, ovarian CSCs were identified from primary human tumors,4 and many studies have reported that ovarian CSC-enriched spheres were commonly found in the ascites of ovarian cancer patients. And these ovarian CSC-enriched spheres are able to initiate tumors and induce drug resistance in patients.5,6 Many studies showed that the drug resistance of CSCs was associated with increased expression of multidrug resistance proteins, such as Oct-4, Sox-2, Notch, ABCG2, etc.7 Furthermore, it has been reported that ovarian cancer cells with high aldehyde dehydrogenase (ALDH) activity exhibited CSC-like properties and chemoresistance. Thus, ALDH is also regarded as a marker of ovarian CSCs.8 Facing the current situation of ovarian cancer treatment, other than traditional platinum-based chemotherapy, the requirement for novel therapeutics against ovarian cancer is demanding and of great importance.
Emerging studies have reported the effects and related mechanisms of natural products on the inhibition of the growth and proliferation of CSCs based on different CSC models in recent years.9 Proanthocyanidins (PAs), also known as condensed tannins, are oligomers and polymers of flavan-3-ols and epigallocatechin-3-O-gallate (EGCG) is a type of flavan-3-ol monomer.10 They were reported to be potent chemoprevention agents against certain types of CSCs by targeting different pathways, such as NF-κB, MAPK, Notch, etc.11,12 The Chinese bayberry (Myrica rubra Sieb. et Zucc.) has been cultivated in southern China for more than 2000 years and is popular among local people due to its special flavor.13 However, leaves from bayberry trees are always abandoned after harvest, which causes huge ecological waste. Chinese bayberry leaves contain rich PAs with special structures. Chinese bayberry leaf PAs (BLPs) contain EGCG as the terminal and most of their extension units, and greater than 98% of them are galloylated, which is quite unusual in the plant kingdom.14–16 BLPs contain about 77.1% of oligomers (monomeric, dimeric, trimeric and tetrameric PAs). Results from previous studies demonstrated that proanthocyanidin oligomers with a degree of polymerization lower than 5 are absorbable based on different in vitro and in vivo models, despite the fact that the absorption rate was different.10 Having a high content of oligomeric PA, our former study showed that BLPs exhibited strong cellular antioxidant and antiproliferative activities via the HepG2 cell model.14 However, their anti-cancer function remains unknown and is worth further investigation.
CSCs produce a tumor mass via continuous self-renewal, which can be regulated by several signaling pathways, such as Wnt/β-catenin, Hedgehog, Notch, etc.9 Understanding the underlying mechanism of the self-renewal behavior of CSCs is of great importance. Since Wnt1 was identified as a mammary oncogene and various studies have revealed that Wnt/β-catenin potently regulated the characteristics of CSCs, such as sphere formation, differentiation ability and tumor development, Wnt/β-catenin is strongly associated with tumorigenesis compared with the Notch and Hedgehog signaling pathways.17 In general, Wnt proteins bind with Frizzle receptors and result in the cytoplasmic accumulation of β-catenin whose translocation leads to the activation of some target genes, such as c-Jun, c-Myc, cyclin D1, etc. The overexpression of β-catenin resulted in resistance against radiation of mouse mammary stem/progenitor cells.18 Also, high expression of β-catenin has been commonly found in different types of ovarian cancers and it was strongly associated with tumor grade and poor prognosis.19 On the other hand, elimination of β-catenin was reported to abrogate chemoresistance in tumorigenic liver progenitor cells.20 The presence of activated β-catenin in various cancers underlines the fact that Wnt/β-catenin signaling is an attractive target for cancer treatment, including ovarian cancer. Therefore, targeting at the Wnt/β-catenin self-renewal pathway might provide an effective strategy to fight against ovarian CSCs and to overcome tumor resistance and recurrence. Furthermore, defective cell cycle events lead to uncontrolled cell proliferation, which is considered as one of the hallmarks of cancer. Oncogenic processes exhibit their greatest effects by targeting G1 phase progression.21 Thus, focusing on the regulation of the G1 cell cycle phase is also one of the important anti-cancer therapeutics.
In the present study, we examined the efficacy of BLPs against chemotherapy-resistant ovarian cancer spheroid (SP) cells. The OVCAR-3-SP cells were enriched by nonadherent sphere culturing and exhibited stem cell traits with higher ALDH+ population and higher expression of Oct-4 and Sox-2. We further investigated how the BLP treatment affected the stem cell traits of OVCAR-3-SP cells. The effects of BLPs on the Wnt/β-catenin self-renewal pathway and cell cycle distribution of OVCAR-3 SP cells were further explored as well. BLPs as a valuable source of bioactive compounds exhibited strong inhibitory effects on the growth of OVCAR-3-SP cells and might hold great value in the treatment against ovarian cancer.
2. Methods and materials
2.1 Chemicals and reagents
Propidium iodide was purchased from Sigma-Aldrich (Sigma, St Louis, MO, USA). Antibodies against Oct-4, Sox-2, p-β-catenin, β-catenin, p-Akt, Akt, c-Myc, p-GSK3β, GSK3β and cyclin D1 were purchased from Cell Signaling Technology (Beverly, MA, USA). Antibodies against GAPDH were purchased from Santa Cruz Biotechnology (Dallas, Texas, USA).
2.2 Proanthocyanidins from Chinese bayberry leaves
BLPs were obtained according to former studies from our group.14 The total phenolic content of BLPs is 378.28 ± 0.97 milligrams of gallic acid equivalents per gram dry weight. BLPs contain 5.4% monomeric, 10.9% dimeric, 13% trimeric, 47.8% tetrameric PAs and 22.9% polymeric PAs or other phenolics.
2.3 Cell culture
The platinum-resistant human ovarian cancer cell line OVCAR-3 was kindly provided by Dr Jiang of West Virginia University. OVCAR-3 cells were cultured in RPMI 1640 medium (Sigma, St Louis, MO, USA) supplemented with 20% US-qualified fetal bovine serum (Invitrogen, Grand Island, NY, USA) at 37 °C with 5% CO2. To culture spheres of cells, OVCAR-3 cells were harvested and seeded at a density of 2 × 104 cells per well in 6-well ultra-low attachment plates (Corning, NY, USA) in Mammocult complete medium (STEMCELL Technologies, Vancouver, BC, Canada) for 7 days. Spheres were transferred to the next generation by dissociation into single cells with Accutase (STEMCELL Technologies, Vancouver, BC, Canada) and seeded at a density of 2 × 104 cells per well. Third-generation spheroid (SP) cells were used for all the following experiments.
2.4 Determination of aldehyde dehydrogenase (ALDH) activity by flow cytometry
ALDH activity was determined using the Aldefluor assay kit (STEMCELL Technologies, Vancouver, BC, Canada) as described by the manufacturer. Dissociated single cells were suspended in the Aldefluor assay buffer containing the ALDH substrate, bodipy-aminoacetaldehyde (BAAA) and incubated for 45 min at 37 °C with 5% CO2 (test tube). An identical reaction was also performed with 15 mM diethylaminobenzaldehyde (DEAB), an ALDH-specific inhibitor (control tube). Cells were analyzed by using a flow cytometry system (BD Biosciences) and the results were analyzed using FCS software (De Novo Software, CA, USA). The ALDH activity of samples was measured based on the fluorescence intensity beyond the threshold defined by the reaction with DEAB.
2.5 Cell viability assay
OVCAR-3 SP cells were seeded into 96-well plates at a density of 2 × 104 cells per well in medium with 10% FBS at 37 °C with 5% CO2 and were treated with BLPs at different concentrations for 24 h. Cell viability was determined by using the CellTiter 96 Aqueous One Solution Cell Proliferation assay (Promega, Madison, WI, USA) based on the manufacturer's instructions. The results were expressed as a percentage compared to the control cells (vehicle treatment).
2.6 Sphere formation assay
To determine the self-renewal ability of OVCAR-3 SP cells in vitro, a sphere formation assay was performed based on a previous study.11 Single cells were seeded in 6-well ultra-low attachment plates (Corning, NY, USA) in Mammocult complete medium at a density of 1000 cells per well. Spheres were photographed after 7 days of incubation. All assays were performed in at least three replicates.
2.7 Colony formation assay
The ability of BLPs to alter the stemness of OVCAR-3 SP cells was determined by a colony formation assay based on a previous study.22 Briefly, 150
000 OVCAR-3 SP cells were seeded per well in 6-well ultra-low attachment plates and incubated for 24 h in complete growth media. Then, the cells were collected and treated with BLPs at different concentrations for another 24 h. The cells were collected and seeded into new 6-well plates at a density of 1000 cells per well and incubated for 10–12 days in complete growth media. Afterwards, the media were removed and the cells were fixed in 70% ethanol for 15 min and the cells were stained with crystal violet for observation.
2.8 Western blot assay
OVCAR-3 SP cells were treated with BLPs at different concentrations for 24 h and were harvested with the M-PER Mammalian Protein Extraction Reagent and Halt Protease and Phosphatase Inhibitor (Thermo Fisher Scientific, Waltham, MA, USA). Total protein concentrations were determined by the BCA protein kit (Thermo Fisher Scientific). Equal amounts of proteins were separated by SDS-polyacrylamide gel electrophoresis and then transferred to polyvinylidene difluoride filter membranes (GE Healthcare, Chicago, IL, USA). Afterwards, the membranes were blocked with 5% nonfat milk with TBST for 1 h and then were incubated with the first antibody overnight at 4 °C and appropriate secondary antibodies conjugated with horseradish peroxidase. Bands were detected by the ECL western blot detection reagents (Thermo Fisher Scientific) and exposed to a Mini-Protean 3 System (Bio-Rad, Atlanta, GA, USA).
2.9 Flow cytometry analysis for cell cycle
OVCAR-3 SP cells were treated with BLPs at different concentrations for 24 h and were then harvested by centrifugation (1500 rpm for 15 min). The cell pellets were suspended with 70% ethanol at −20 °C overnight. Afterwards, cells were washed with PBS twice and incubated with RNase (180 μg mL−1) for 30 min at 37 °C, followed by incubation with PI solution (final concentration: 50 μg mL−1) for 30 min in the dark. Cells were analyzed by using a flow cytometry system (BD Biosciences) and results were analyzed using FCS software (De Novo Software, CA, USA).
2.10 Statistical analysis
Results are presented as the mean ± standard deviation (SD) for at least three replicates for each sample. Statistical analyses were performed using the SPSS program, version 17.0 (SPSS Inc., 2009). Data were analysed by ANOVA and a two-tailed Student's t-test and significant differences were set at p < 0.05, p < 0.01 and p < 0.001.
3. Results
3.1 Spheroid (SP) cells isolated from OVCAR-3 ovarian cancer cells exhibited stem cell-like properties
With self-renewal ability, CSCs grow as nonadherent spheres under stem-cell-selective conditions, which is different from monolayer cancer cells. OVCAR-3 cells were seeded in ultra-low-attachment culture plates in Mammocult complete medium at a density of 2 × 104 cells per well for 7 days. Cell aggregates were formed and some cells died due to the low serum conditions (Fig. 1A). After the first 7-day incubation period, the first generation of OVCAR-3 SP cells was collected and the rest of the cells were isolated by centrifugation at 800 rpm for 5 min and were re-seeded on ultra-low-attachment culture plates for the second and third generation cultures. To determine whether the SP cells generated from the OVCAR-3 cells exhibited CSC-like properties, the cells were investigated by ALDH assay and western blot assay. ALDH has been reported to be a CSC marker and its activity was determined by flow cytometry with the Aldefluor assay kit in the present study. As shown in Fig. 1C & D, the percentage of ALDH+ cells in adherent (AD) cells and the first, second and third generations of SP cells was 0.87 ± 0.03%, 6.31 ± 0.77%, 9.76 ± 0.17% and 23.50 ± 1.93%, respectively. The third generation of SP cells exhibited the highest population of ALDH+ and thus were collected for further investigation. Ovarian CSCs were reported to express stem cell-related genes, such as SOX, OCT-4, etc.23 Therefore, the expression of stem cell-related proteins was further determined by a western blot assay. Compared with OVCAR-3 AD cells, the expressions of Sox-2 and Oct-4 in OVCAR-3 SP cells (3rd generation) were 1.91 ± 0.12 and 2.55 ± 0.25 fold of those of the OVCAR-3 AD cells (Fig. 1B & E). These results indicated that OVCAR-3 SP cells exhibited stem cell-like properties.
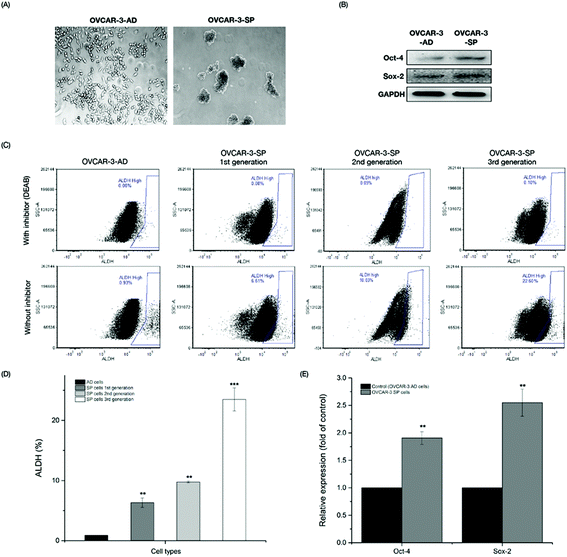 |
| Fig. 1 SP cells from OVCAR-3 ovarian cancer cells exhibited stem cell-like characteristics. (A) Images of OVCAR-3 AD cells and OVCAR-3 SP cells are shown. OVCAR-3 SP cells were generated from OVCAR-3 AD cells and cultured under spheroid-forming conditions and were photographed on day 7. (B) OVCAR-3 SP cells (3rd generation) showed higher expression of stem cell-related proteins (Oct-4 and Sox-2). (C) Flow cytometric analysis of OVCAR-3 AD cells and OVCAR-3 SP cells after incubation with the ALDH substrate (with and without the inhibitor DEAB) is shown. (D) The percentage of ALDH in OVCAR-3 AD cells and OVCAR-3 SP cells (1st, 2nd and 3rd generation) is shown. Results are representative of three independent experiments and are expressed as mean ± SD. (**) p < 0.01, (***) p < 0.001, compared with the control. (E) Relative expression of Oct-4 and Sox-2 in OVCAR-3 SP cells. Oct-4, Sox-2 and GAPDH protein expressions were detected by western blot analysis and quantified by ImageJ software. Results are representative of three independent experiments and are expressed as mean ± SD. (**) p < 0.01, two-tailed Student's t-test. | |
3.2 BLPs inhibited the growth and proliferation of OVCAR-3 SP cells
To investigate the cytotoxic effect of BLPs on OVCAR-3 SP cells, the CellTiter 96 Aqueous One Solution Cell Proliferation assay was performed. As shown in Fig. 2, BLP treatment dose-dependently inhibited the growth and proliferation of OVCAR-3 SP cells (p < 0.01). The cell viability reduced from 81.4 ± 2.0% to 44.4 ± 0.7% after treating with BLPs from 2 to 20 μg mL−1 for 24 h. The IC50 value was about 16.4 μg mL−1. This indicated that BLPs have a great potential to inhibit the growth and proliferation of OVCAR-3 SP cells.
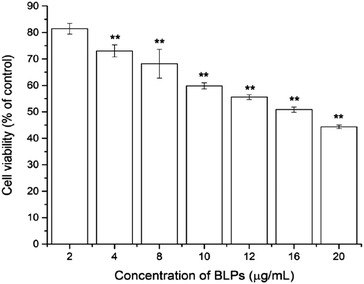 |
| Fig. 2 BLPs inhibited the viability of OVCAR-3 SP cells in a dose dependent manner. Results are representative of three independent experiments and are expressed as mean ± SD. (**) p < 0.01, compared with the control. | |
3.3 BLPs regulated CSC-like characteristics in OVCAR-3 SP cells
Sphere formation and colony formation, which are the characteristics of ovarian CSCs, were investigated in the present study to explore how BLP treatment affected OVCAR-3 SP cells. After treating with BLPs, the sphere formation ability of OVCAR-3 SP cells decreased significantly (Fig. 3A) by showing a much smaller size and diameter of the spheres of BLP treatment groups compared with those of the control group. Furthermore, BLPs also dose-dependently reduced the number of colonies formed by OVCAR-3 SP cells (Fig. 3B & C). BLPs at 10 μg mL−1 reduced approximately 79.6% of colonies compared with the control group, which suggested that BLPs induced cytotoxicity and proliferation inhibition in cells. These results were consistent with Fig. 2 and revealed that BLPs attenuated the stem cell characteristics in OVCAR-3 SP cells.
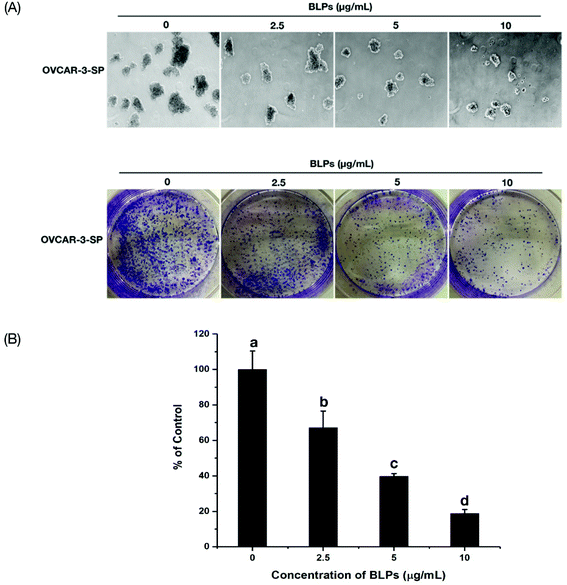 |
| Fig. 3 BLPs regulated CSC-like characteristics in OVCAR-3 SP cells. (A) BLPs inhibited the sphere formation ability of OVCAR-3 SP cells. Single cells were seeded in 6-well ultra-low attachment plates in Mammocult complete medium at a density of 1000 cells per well. Spheres were photographed after 7 days of incubation. All assays were performed in at least three replicates. (B & C) BLPs inhibited the colony formation ability of OVCAR-3 SP cells. Cells were seeded at a density of 150 000 cells per well in the 6-well ultra-low attachment plates and incubated for 24 h in complete growth media and then treated with BLPs at 2.5, 5 and 10 μg mL−1 for another 24 h. Cells were collected and seeded into new 6-well plates at a density of 1000 cells per well and incubated for 10–12 days in complete growth media. Afterwards, media were removed and cells were fixed in 70% ethanol for 15 min and cells were stained with crystal violet for observation. The number of colonies was counted using ImageJ software and was adjusted to the percentage of the control. Results are representative of three independent experiments and are expressed as mean ± SD. Different letters refer to statistically significant differences at p < 0.05. | |
3.4 BLPs regulated the ALDH+ population and the expression of stemness-related proteins in OVCAR-3 SP cells
The mechanism of BLPs regulating the CSC-like characteristics in OVCAR-3 SP cells was further investigated by the ALDH assay and western blot assay. Fig. 4A shows that BLP treatment dose-dependently reduced the percentage of ALDH+ cells compared with the control (p < 0.01). Treating with 5 and 10 μg mL−1 of BLPs approximately reduced about 24.7% and 61.8% of ALDH+ cells compared with the control (Fig. 4B). Furthermore, BLPs also regulated the stemness-related proteins of OVCAR-3 SP cells in a dose-dependent manner (Fig. 4C). At the dosage of 10 μg mL−1, BLPs reduced the expression of Oct-4 and Sox-2 for about 52.7% and 36.3%, compared with the control (p < 0.001) (Fig. 4D). By regulating the ALDH+ population and the expression of stemness-related proteins, BLPs affected the CSC-like characteristics in OVCAR-3 SP cells, which was also consistent with former results.
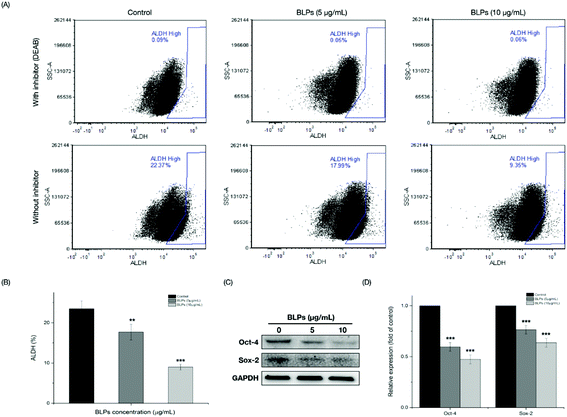 |
| Fig. 4 BLPs regulated the population of ALDH+ and the expression of stemness-related proteins in OVCAR-3 SP cells. (A) Flow cytometric analysis of OVCAR-3 SP cells with or without BLP treatment after incubation with the ALDH substrate (with and without the inhibitor DEAB) is shown. (B) Percentage of ALDH in OVCAR-3 SP cells with or without BLP treatment. Results are representative of three independent experiments and are expressed as mean ± SD. (**) p < 0.01, (***) p < 0.001, compared with the control. (C & D) BLPs reduced the expression of stem cell-related proteins (Oct-4 and Sox-2) in OVCAR-3 SP cells. Oct-4, Sox-2 and GAPDH protein expressions were detected by western blot analysis and quantified using ImageJ software. Results are representative of three independent experiments and are expressed as mean ± SD. (***) p < 0.001, compared with the control. | |
3.5 BLPs downregulated the Wnt/β-catenin self-renewal pathway in OVCAR-3 SP cells
Next, we further investigated the effects of BLPs on the self-renewal pathway in OVCAR-3 SP cells. The Wnt/β-catenin pathway plays an important role in stem cell self-renewal properties and many studies have shown that β-catenin activation is vital for CSC differentiation and inhibition of tumor recurrence.24 Therefore, key proteins related to the Wnt/β-catenin pathway were explored in OVCAR-3 SP cells. As shown in Fig. 5, BLP treatment dose-dependently increased the expression of p-β-catenin (p < 0.01) and reduced the expression of β-catenin at a higher dosage (10 μg mL−1). It has been reported that GSK3β can phosphorylate β-catenin at Ser33/Ser37/Thr41 and cause ubiquitin-proteasome degradation of β-catenin. On the other hand, the phosphorylation of GSK3β decreases the activity of GSK3β and therefore stabilizes β-catenin.25 Our result further showed that BLP treatment reduced the expression of p-GSK3β and thus increased the activity of GSK3β and induced the degradation of β-catenin. The BLP-induced phosphorylation of β-catenin further inhibits its downstream proteins, such as cyclin D1 and c-Myc (p < 0.01). Compared with the control, the expression of cyclin D1 and c-Myc was reduced by about 30.8% and 24.1% after treating with 10 μg mL−1 of BLPs. Furthermore, Akt was also reported to be associated with the self-renewal ability of cells and stem cell survival. The inhibition of Akt in mammary stem cells promotes β-catenin phosphorylation.26 BLP treatment significantly reduced the expression of p-Akt in a dose-dependent fashion (p < 0.01) and thus inhibited the activity of Akt, which might contribute to the degradation of β-catenin in OVCAR-3 SP cells. Our results were in accordance with some previous studies that targeting Akt, GSK3β and β-catenin interfered with the properties of CSCs.24,27
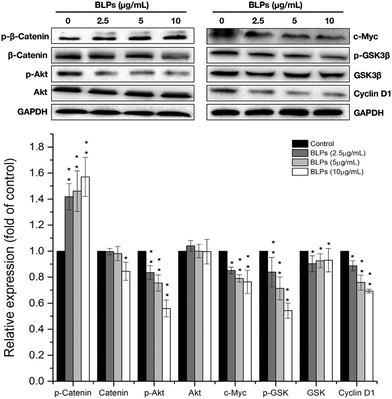 |
| Fig. 5 BLPs downregulated the Wnt/β-catenin self-renewal pathway in OVCAR-3 SP cells. Cells were treated with BLPs at 2.5, 5 and 10 μg mL−1 for 24 h. p-β-Catenin, β-catenin, p-Akt, Akt, c-Myc, p-GSK3β, GSK3β, cyclin D1 and GAPDH protein expressions were detected by western blot analysis and quantified using ImageJ software. Results are representative of three independent experiments and are expressed as mean ± SD. (*) p < 0.05 and (**) p < 0.01, compared with the control. | |
3.6 BLPs induced G1 cell cycle arrest in OVCAR-3 SP cells
Due to the potent inhibitory effects of BLPs on the proliferation and growth of OVCAR-3 SP cells, we further examined whether cell cycle progression was one of the mechanisms or not. OVCAR-3 SP cells were treated with BLPs at 2.5, 5 and 10 μg mL−1 for 24 h and the distribution of cell cycle phases was determined by flow cytometry. Table 1 shows that the distribution of G1 significantly increased after BLP treatment (p < 0.01). BLPs at 10 μg mL−1 increased 16.4% of G1 cell cycle distribution compared with the control. However, there were no significant changes in the distribution of the S phase and G2 phase after the BLP treatment. These results indicated that BLPs induced G1 cell cycle arrest in OVCAR-3 SP cells.
Table 1 Cell cycle phase distribution of OVCAR-3 SP cells with BLP treatment
BLPs (μg mL−1) |
Cell cycle phase distribution |
%G1 |
%G2 |
%S |
Data are expressed as mean values ± SD (n = 3). Values with no letters in common with each column are significantly different (p < 0.05). |
0 |
46.66 ± 1.28c |
13.56 ± 2.60ab |
39.78 ± 1.34b |
2.5 |
49.43 ± 1.12b |
17.27 ± 3.10b |
33.29 ± 2.47a |
5 |
52.73 ± 0.70a |
10.96 ± 2.42a |
36.31 ± 1.74a |
10 |
54.29 ± 1.73a |
12.70 ± 2.83ab |
33.02 ± 1.11a |
4. Discussion
Ovarian cancer is one of the most lethal gynecological cancers among women in the world. Platinum-based chemotherapy is effective at the initial stage for ovarian cancer treatment. However, a high recurrence rate and relapse are still the main impediments to ovarian cancer treatment.1 CSCs as a malignant and aggressive cancer phenotype have been studied extensively in recent years. CSCs are known to be platinum-resistant and therefore are attributed to be one of the root causes of chemotherapy failure and tumor recurrence.27 Ovarian cancer has been evaluated as a type of CSC-related cancer in recent years; therefore, targeting ovarian CSCs is of great importance in ovarian cancer treatment. In the present study, the CSC population was enriched in ovarian cancer cells through spheroid formation, which is a defining characteristic of epithelial stem cells.23 Many studies have reported that several cancer cells with high levels of migration, tumorigenic activity and metastatic ability exhibited high-ALDH populations. ALDH not only plays an important role in maintaining the functions of stem cells, but also acts as an important marker of various types of cancers. Thus, high ALDH activity detected by the ALDEFLUOR assay can be used as a functional marker for CSC isolation and identification, including ovarian, breast, lung, pancreatic and colon cancers.28 Compared with OVCAR-3 AD cells, the percentage of the ALDH+ population was increasing from the first generation (6.31 ± 0.77%) to the third generation (23.50 ± 1.93%) of the OVCAR-3 SP cells (Fig. 1C & D). Thus, the third generation of OVCAR-3 SP cells was collected for further assays. Other than showing a higher population of ALDH+, OVCAR-3 SP cells also exhibited a higher expression of stemness-related proteins, such as Oct-4 and Sox-2, compared with OVCAR-3 AD cells. As transcriptional factors, Oct-4 and Sox-2 are highly expressed in undifferentiated embryonic stem cells. Oct-4 can form a heterodimer with Sox-2 and bind to thousands of gene regulatory sites, which are important for the regulation of cell pluripotency and early embryonic development.29 Studies have shown that high expression of Oct-4 and Sox-2 is also associated with tumors, and therefore they are implicated as markers for CSCs.30 With a higher ALDH+ population and expression of stemness-related proteins, OVCAR-3 SP cells exhibited stem cell-like properties and therefore were used as the model to study the effects of BLPs on the regulation of ovarian CSCs.
The regulation by BLPs regarding the CSC-like characteristics of OVCAR-3 SP cells was firstly examined by sphere formation and colony formation assays. The ability to form spheres and colonies is one of the main characteristics of CSCs. PAs have been reported to inhibit the growth of different types of CSCs in vitro by attenuating the ability to form spheres and colonies.9 For instance, EGCG was reported to inhibit cell proliferation and sphere formation via the AMP-activated protein kinase pathway in breast CSCs and inhibit sphere formation in neuroblastoma CSCs via targeting sonic Hedgehog signaling.11 Such results were also in accordance with our results that BLPs containing EGCG as most of its extension and terminal units also exhibited a strong ability to inhibit sphere and colony formation in ovarian CSCs by significantly reducing the size of spheres and the number of colonies. The ability to inhibit CSC-like properties in OVCAR-3 SP cells was further confirmed by the reduced population of ALDH+ cells and the decreased expression of stemness-related proteins (Oct-4 and Sox-2) after BLP treatment. Previous studies have revealed that high levels of ALDH, Sox-2 and Oct-4 increased the CSC characteristics and maintained platinum resistance in CSCs.6,23 EGCG was reported to reduce the expression of stem cell markers, such as Oct-4, Sox-2, Nanog, etc., in head and neck squamous carcinoma (HNSC) CSCs in vivo and thus regulated the self-renewal capacity and chemosensitivity of HNSC CSCs.11 Similar results were also observed in the present study that BLPs reduced the levels of ALDH, Sox-2 and Oct-4 and therefore inhibited the growth and drug resistance of OVCAR-3 SP cells.
Multiple signaling pathways are involved in the maintenance and self-renewal capacity of CSCs, and the Wnt/β-catenin signaling pathway is one of those. β-Catenin, as an essential mediator of Wnt signaling, can translocate into the nucleus and bind with the transcription factors T-cell factor/lymphoid enhancer factor (TCF/LEF), which eventually leads to the activation of Wnt target genes, such as c-Myc, cyclin D1, c-Jun, etc.9 c-Myc as a transcriptional factor can bind with DNA, and thus activates the transcription for cell proliferation, differentiation and stem cell self-renewal.31 Cyclin D1 is a protein required for the G1 phase of the cell cycle and its mutation, amplification or overexpression can alter cell cycle progression, which might contribute to tumorigenesis.32 Thus, targeting the activity of β-catenin is the key point to inhibit the self-renewal capacity of CSCs in Wnt/β-catenin signaling. The level of intracellular β-catenin can be regulated by GSK3β, which promotes the ubiquitin–proteasome degradation of β-catenin by phosphorylating it at Ser33/Ser37/Thr41.24 Furthermore, activated Akt (i.e., phospho-Akt Ser473) has been reported to be able to phosphorylate Ser9 on GSK3β, which leads to the inactivation of GSK3β and thus stabilizes β-catenin.33,34 Therefore, inhibiting the activity of Akt is also important for the destabilization of β-catenin and the inhibition of Wnt/β-catenin signaling. Our results revealed that BLP treatment decreased the expression of p-Akt, which led to the reduction of p-GSK3β and therefore promoted the activity of GSK3β. Afterwards, the BLP-induced GSK3β upregulation enhanced the expression of p-β-catenin and led to the decreased activity of β-catenin and further resulted in the reduction of c-Myc and cyclin D1. Thus, our results further revealed that BLPs inhibited the self-renewal capacity and therefore inhibited the CSC-like characteristics of OVCAR-3 SP cells by targeting the Wnt/β-catenin signaling pathway.
Abnormal cell cycle control results in the uncontrolled proliferation and growth of tumors, which is also regarded as one of the hallmarks of cancer.35 Generally, cells undergo four stages (G0, G1, G2 and S phases) to divide and duplicate their DNA. During the G1 phase, cells synthesize mRNA and proteins in preparation for the following steps leading to mitosis. Several proteins participate in the regulation of the G1 phase, such as D type cyclins. In the present study, we have demonstrated that BLPs induced G1 cell cycle arrest in OVCAR-3 SP cells and such a result was also confirmed by the reduced expression of cyclin D1. Cyclin D1, as an important regulatory subunit of cyclin-dependent kinase (CDK) 4/6, can assemble with CDK4/6 into holoenzyme complexes to regulate the G1/S phase transition.21 Many previous studies have reported that cyclin D1 was overexpressed in human melanomas.36 Thus, focusing on the G1 cell cycle checkpoint by targeting the cyclin D1–CDK complex is one of the anti-cancer therapeutics. EGCG and other types of PAs have been reported to induce G1 cell cycle arrest in different types of cancer cells by targeting D type cyclins.37,38 However, their effects on the cell cycle distribution of ovarian CSCs remain unknown. Our results demonstrated that BLPs containing EGCG as their terminal and major extension units caused G1 cell cycle arrest in OVCAR-3 SP cells via reducing the expression of cyclin D1, which was in accordance with some previous studies and also further confirmed the anti-cancer function of PAs.
5. Conclusion
In the present study, chemotherapy-resistant OVCAR-3 SP cells were enriched by sphere culturing and exhibited CSC-like properties by showing a significantly higher ALDH+ population and higher expression of stemness-related proteins compared with OVCAR-3 AD cells. BLP treatment reduced cell viability, sphere and colony formation ability, ALDH level and the expression of stemness-related proteins and thus inhibited the growth and CSC traits of OVCAR-3 SP cells. Furthermore, BLPs targeted the Wnt/β-catenin signaling pathway to inhibit the self-renewal capacity of OVCAR-3 SP cells and also induced G1 cell cycle arrest. Overall, BLPs as a valuable source of natural products strongly inhibited the growth and the CSC traits of OVCAR-3 SP cells. Therefore, they have great potential to be developed as a potent antitumour initiating agent to promote public health.
Author contributions
Y. Z. designed the research, carried out the experiments, and prepared the manuscript. C. Y. W. and Y. C. C. participated in the design of the study. Y. C. C., G. O. R., X. Q. Y. and S. G. C. conceived and coordinated the project, and prepared the manuscript. All authors read and approved the final manuscript.
Conflicts of interest
The authors declare no conflict of interest.
Acknowledgements
The authors thank Dr Kathy Brundage from the Flow Cytometry Core at the West Virginia University for providing technical help on apoptosis analysis. This research was supported by NIH grants P20RR016477 from the National Center for Research Resources and P20GM103434 from the National Institute for General Medical Sciences (NIGMS) awarded to the West Virginia IDeA Network of Biomedical Research Excellence. This research was supported by grant number P20GM104932 from NIGMS, a component of the National Institutes of Health (NIH) and its contents are solely the responsibility of the authors and do not necessarily represent the official view of NIGMS or NIH. This study was also supported by the COBRE grant GM102488/RR032138, the ARIA S10 grant RR020866, the FORTESSA S10 grant OD016165 and the INBRE grant GM103434. This research was also supported by the National Natural Science Foundation of China (C200501) and the National Key Research and Development Program (2016YFD0400805).
References
- B. Li, Y. Gao, G. O. Rankin, Y. Rojanasakul, S. J. Cutler, Y. Tu and Y. C. Chen, Cancer Lett., 2015, 356, 418–433 CrossRef CAS PubMed.
- J. Li, S. Condello, J. Thomes-Pepin, X. Ma, Y. Xia, T. D. Hurley, D. Matei and J. X. Cheng, Cell Stem Cell, 2017, 20, 303–314e305 CrossRef CAS PubMed.
- H. G. Kang, D.-H. Kim, S.-J. Kim, Y. Cho, J. Jung, W. Jang and K.-H. Chun, Oncotarget, 2016, 7, 68229 Search PubMed.
- S. Zhang, C. Balch, M. W. Chan, H. C. Lai, D. Matei, J. M. Schilder, P. S. Yan, T. H. Huang and K. P. Nephew, Cancer Res., 2008, 68, 4311–4320 CrossRef CAS PubMed.
- K. M. Burleson, M. P. Boente, S. E. Pambuccian and A. P. Skubitz, J. Transl. Med., 2006, 4, 6 CrossRef PubMed.
- E. J. Seo, D. K. Kim, I. H. Jang, E. J. Choi, S. H. Shin, S. I. Lee, S.-M. Kwon, K.-H. Kim, D.-S. Suh and J. H. Kim, Oncotarget, 2016, 7, 55624 Search PubMed.
- L. Ma, D. Lai, T. Liu, W. Cheng and L. Guo, Acta Biochim. Biophys. Sin., 2010, 42, 593–602 CrossRef CAS PubMed.
- T. Mizuno, N. Suzuki, H. Makino, T. Furui, E. Morii, H. Aoki, T. Kunisada, M. Yano, S. Kuji and Y. Hirashima, Gynecol. Oncol., 2015, 137, 299–305 CrossRef CAS PubMed.
- Y. Li, M. S. Wicha, S. J. Schwartz and D. Sun, J. Nutr. Biochem., 2011, 22, 799–806 CrossRef CAS PubMed.
- K. Ou and L. Gu, J. Funct. Foods, 2014, 7, 43–53 CrossRef CAS.
- S. H. Lee, H. J. Nam, H. J. Kang, H. W. Kwon and Y. C. Lim, Eur. J. Cancer, 2013, 49, 3210–3218 CrossRef CAS PubMed.
- S. S. Chung and J. V. Vadgama, Anticancer Res., 2015, 35, 39–46 CAS.
- Y. Zhang, S. Chen, C. Wei, H. Gong, L. Li and X. Ye, PLoS One, 2016, 11, e0167484 Search PubMed.
- Y. Zhang, X. Zhou, W. Tao, L. Li, C. Wei, J. Duan, S. Chen and X. Ye, J. Funct. Foods, 2016, 27, 645–654 CrossRef CAS.
- Y. Fu, L. Qiao, Y. Cao, X. Zhou, Y. Liu and X. Ye, PLoS One, 2014, 9, e96162 Search PubMed.
- H. Yang, X. Ye, D. Liu, J. Chen, J. Zhang, Y. Shen and D. Yu, J. Agric. Food Chem., 2011, 59, 1622–1629 CrossRef CAS PubMed.
- N. Wang, Z. Wang, Y. Wang, X. Xie, J. Shen, C. Peng, J. You, F. Peng, H. Tang and X. Guan, Oncotarget, 2015, 6, 9854 Search PubMed.
- W. A. Woodward, M. S. Chen, F. Behbod, M. P. Alfaro, T. A. Buchholz and J. M. Rosen, Proc. Natl. Acad. Sci. U. S. A., 2007, 104, 618–623 CrossRef CAS PubMed.
- S. Deng, C. K. C. Wong, H.-C. Lai and A. S. T. Wong, Oncotarget, 2017, 8, 25897 Search PubMed.
- W. Yang, H.-X. Yan, L. Chen, Q. Liu, Y.-Q. He, L.-X. Yu, S.-H. Zhang, D.-D. Huang, L. Tang and X.-N. Kong, Cancer Res., 2008, 68, 4287–4295 CrossRef CAS PubMed.
- C. J. Sherr, Science, 1996, 274, 1672–1677 CrossRef CAS PubMed.
- A. R. Massey, L. Reddivari, S. Radhakrishnan, V. Charepalli, E. K. Kurundu Hewage, V. Ramakrishna and J. K. P. Vanamala, J. Funct. Foods, 2016, 23, 601–613 CrossRef CAS.
- D. K. Kim, E. J. Seo, E. J. Choi, S. I. Lee, Y. W. Kwon, I. H. Jang, S. C. Kim, K. H. Kim, D. S. Suh, K. Seong-Jang, S. C. Lee and J. H. Kim, Exp. Mol. Med., 2016, 48, e255 CrossRef CAS PubMed.
- Y. Li, T. Zhang, H. Korkaya, S. Liu, H. F. Lee, B. Newman, Y. Yu, S. G. Clouthier, S. J. Schwartz, M. S. Wicha and D. Sun, Clin. Cancer Res., 2010, 16, 2580–2590 CrossRef CAS PubMed.
- C. Liu, Y. Li, M. Semenov, C. Han, G.-H. Baeg, Y. Tan, Z. Zhang, X. Lin and X. He, Cell, 2002, 108, 837–847 CrossRef CAS PubMed.
- C.-H. Wu, B.-H. Hong, C.-T. Ho and G.-C. Yen, J. Agric. Food Chem., 2015, 63, 2432–2441 CrossRef CAS PubMed.
- A. B. Nagaraj, P. Joseph, O. Kovalenko, S. Singh, A. Armstrong, R. Redline, K. Resnick, K. Zanotti, S. Waggoner and A. DiFeo, Oncotarget, 2015, 6, 23720 Search PubMed.
- S. Shankar, D. Nall, S. N. Tang, D. Meeker, J. Passarini, J. Sharma and R. K. Srivastava, PLoS One, 2011, 6, e16530 CAS.
- L. A. Boyer, T. I. Lee, M. F. Cole, S. E. Johnstone, S. S. Levine, J. P. Zucker, M. G. Guenther, R. M. Kumar, H. L. Murray, R. G. Jenner, D. K. Gifford, D. A. Melton, R. Jaenisch and R. A. Young, Cell, 2005, 122, 947–956 CrossRef CAS PubMed.
- L. Cheng, M. T. Sung, P. Cossu-Rocca, T. D. Jones, G. T. MacLennan, J. De Jong, A. Lopez-Beltran, R. Montironi and L. H. Looijenga, J. Pathol., 2007, 211, 1–9 CrossRef CAS PubMed.
- T. A. Baudino and J. L. Cleveland, Mol. Cell. Biol., 2001, 21, 691–702 CrossRef CAS PubMed.
- M. Fu, C. Wang, Z. Li, T. Sakamaki and R. G. Pestell, Endocrinology, 2004, 145, 5439–5447 CrossRef CAS PubMed.
- P. Cohen and S. Frame, Nat. Rev. Mol. Cell Biol., 2001, 2, 769 CrossRef CAS PubMed.
- M. Pap and G. M. Cooper, J. Biol. Chem., 1998, 273, 19929–19932 CrossRef CAS PubMed.
- B. Gabrielli, K. Brooks and S. Pavey, Front. Pharmacol., 2012, 3, 9 Search PubMed.
- J. Massagué, Nature, 2004, 432, 298 CrossRef PubMed.
- N. Ahmad, P. Cheng and H. Mukhtar, Biochem. Biophys. Res. Commun., 2000, 275, 328–334 CrossRef CAS PubMed.
- S. M. Meeran and S. K. Katiyar, Front. Biosci., 2008, 13, 2191 CrossRef CAS.
|
This journal is © The Royal Society of Chemistry 2018 |
Click here to see how this site uses Cookies. View our privacy policy here.