A coelenterazine-type bioluminescent probe for nitroreductase imaging†
Received
25th October 2017
, Accepted 30th November 2017
First published on 30th November 2017
Abstract
Novel coelenterazine-type bioluminescent probes have been designed and synthesized to detect nitroreductase (NTR) in hypoxic tumors. The design strategy is that NTR catalyzes the reduction of the nitrobenzyl moiety to the aniline group with an electron donor, thus resulting in 1,4 or 1,6-rearrangement–elimination to release coelenterazine analogues, which can be catalyzed by Renilla luciferase to emit bioluminescence. After careful evaluation, almost all probes had a 3-fold greater response for NTR over other biologically relevant substances at >100-fold dose more than NTR. In the dose-independent and selectivity study, probes A1, A2 and A5 presented a high selectivity in a dose-dependent manner. Overall, among all molecules, probe A5 showed high sensitivity, low cytotoxicity and good compatibility, so as to be successfully applied for assessing the hypoxic status in cellulo and in vivo as the first coelenterazine-type bioluminescent probe.
Introduction
Hypoxia is not only associated with many kinds of diseases, such as solid tumours,1,2 inflammatory disease3 and vascular ischemia,4 but also has adverse effects on the metabolism of various anticancer drugs against treatment.5,6 Clinical research has also certificated that the level of hypoxia in tumour regions is in a positive correlation with the effects.7,8 Hypoxia is a detectable index for some kinds of cancer.9,10 Thence, detecting the level of hypoxia in tumours is significant for evaluating the tumour status and predicting the efficiency of anticancer medicine. At present, several means have been exploited to examine the degree of hypoxia.11–15 Among them, biomarker detection methods play an important role in trials as high selectivity and sensitivity. In most cases, hypoxia is accompanied by elevated levels of reductive enzymes such as nitroreductase (NTR).16 In the presence of an endogenous electron donor (nicotinamide adenine dinucleotide (NADH) or nicotinamide adenine dinucleotide phosphate (NADPH)), nitroreductase can deoxygenize nitrobenzene to aniline. Based on this specific reaction, NTR has been applied to detect the level of hypoxia in tumors17 and bioreductive prodrugs.18,19
Currently, a lot of NTR probes have been developed, such as fluorescent, chemiluminescent and bioluminescent probes.4,20–29 However, some flaws, such as low signal-to-noise ratio and being invasive to cells and organs, restrict their applications. Bioluminescence-based probes are ideal toolkits, which can perfectly surmount the above-mentioned problems. Using Renilla, Gaussia or Oplophorus luciferase activity, coelenterazine emits intense blue light at 480 nm with no cofactor. The coelenterazine-luciferase system has many advantages over firefly and bacterial bioluminescence systems. For example, it is the simplest system with suitable properties to apply in biological studies. The substrate coelenterazine (CTZ) has widespread existence and is utilized by different kinds of luciferases.30 Apparently, such a system displays an excellent ability to detect molecular (thiophenols31) and cellular events32–34 in living animals. Herein, we developed a new type of bioluminescent probe based on coelenterazine-luciferase for nitroreductase, which is highly sensitive and selective in detecting NTR in vitro and in vivo.
Coelenterazine analogues that we modified can be easily synthesized and have the ability to detect ions, endogenous substances, and enzyme activity. The synthesis of coelenterazine analogues started from 2-amino-3,5-dibromopyrazine by using the Negishi coupling to provide the key pyrazine intermediate (3-benzyl-5-bromo-2-amino-pyrazine). Subsequently, the pyrazine core was prepared by using the Suzuki coupling reaction. Finally, a diethoxy derivative was condensed with the pyrazine core in ethanol with concentrated HCl to generate the target molecules. In the strategy for preparing bioluminescent probes compounds B1 and B2
35 are caged with the nitrobenzyl moiety, which is usually applied for nitroreductase detection that nitro is reduced to amino by nitroreductase for the development of prodrugs and sensors.4,36–38 In the presence of NADH, NTR catalyzes the reduction of the nitroaromatic moiety in probes, followed by a cascade reaction, 1,4 or 1,6-rearrangement-elimination reaction, ejects coelenterazine analogues, and produces light under Renilla luciferase catalysis (Scheme 1). We have previously demonstrated35 that compounds B1 and B2 exhibit a higher intensity (7.6- and 16.9-fold) and are more stable than DeepBlueC™. Compared to the emission wavelength of DeepBlueC™, compounds B1 and B2 had red-shifted bioluminescence light (428, 440 nm vs. 411 nm). As the tissue absorption of photons is reduced at near-infrared wavelengths, a red-shift is of great interest in vivo. In addition, compounds B1 and B2 have less chemically reactive groups, which make it easier to use them for preparing probes compared to the native substrate coelenterazine h. Finally, nitroreductase probes were well designed and synthesized on the basis of compounds B1 and B2 as shown in Schemes 1 and S1.†
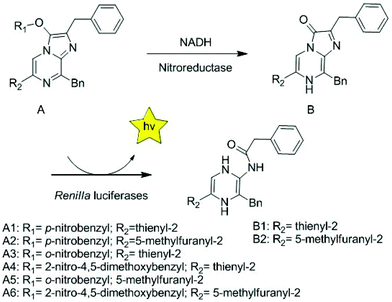 |
| Scheme 1 Proposed mechanism of bioluminescent probes for nitroreductase. | |
Results and discussion
Dose-independent and selectivity study of NTR
Firstly, nitroreductase-independent experiments were carried out in aqueous buffer at physiological pH in order to evaluate the sensibility and specificity of the probes. With different concentrations of NTR (dissolved in Tris-HCl at pH 7.46, with 0.5 mM NADH), solutions of probes at 12.5 μM released the substrate with incubation for 1 h, and the bioluminescence was monitored by using IVIS Kinetic as shown in Fig. S1.† Prior to the addition of NTR, probes were very stable in aqueous buffer and bioluminescence could hardly be acquired. After adding different concentrations of NTR and Renilla luciferase, bioluminescence was immediately acquired without incubation. Probe A1 presented around 500-fold bioluminescence intensity with the concentration of NTR at 10 μg mL−1, probe A2 exhibited around 170-fold enhancement, and probe A5 displayed 3.5-fold enhancement over control. Even at 0.156 μg mL−1 NTR, probe A1 detected it definitely (Fig. S1†). In addition, when the relative bioluminescence intensity increased with the concentration of NTR 0–10 μg mL−1, a linearity was obtained. According to the results, probe A1 has a high intensity to NTR and can be applied to measure the concentration of NTR. As shown in Fig. S1e,† the relative bioluminescence intensity presented a linear relationship with the concentration ranging from 0 to 5 μg mL−1. The detection limit (S/N = 3) of A5 for NTR was 4.32 μg mL−1.
However, the bioluminescence intensities of probes A3–A6 were lower than those of probes A1 and A2. It was probable that nitroreductase which is from Escherichia coli could not well reduce the 2-nitrobenzyl moiety.
In the case of a selectivity study, almost all probes disclosed high selectivity for NTR over other biologically relevant substances. As shown in Fig. 1, these probes displayed more than 3-fold greater response for NTR over 1 mM NaSH, Na2S2O4, VcNa, Cys, Glu and Arg (100-fold higher than NTR tested), NaCl (5000-fold) and glucose (1000-fold) (Fig. 1). It should be noted that aromatic nitro compounds could not be reduced by NTR alone, but could selectively be reduced by NTR in the presence of NADH.
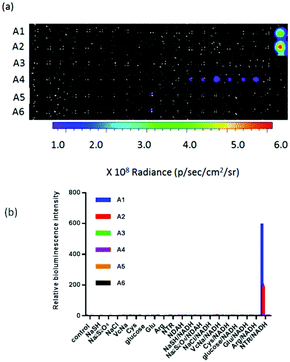 |
| Fig. 1 (a) Bioluminescence imaging of selectivity of the probes with various relevant reductants; (b) relative bioluminescence responses of probes A1–A6 (12.5 μM) reacted with different kinds of species, blank (Tris-HCl), and with NaSH (1 mM), Na2S2O4 (1 mM), NaCl (50 mM), VcNa (1 mM), Cys (1 mM), glucose (10 mM), Glu (1 mM), Arg (1 mM), NTR (10 μM), NADH (0.5 mM), NaSH + NADH (1 mM, 0.5 mM), Na2S2O4 + NADH (1 mM, 0.5 mM), NaCl + NADH (50 mM, 0.5 mM), VcNa + NADH (1 mM, 0.5 mM), Cys + NADH (1 mM, 0.5 mM), glucose + NADH (10 mM, 0.5 mM), Arg + NADH (1 mM, 0.5 mM), and NTR + NADH (10 μg mL−1, 0.5 mM). | |
Cytotoxicity test
To assess the cytotoxicity of probes to cells, we tested probes at various concentrations (6.25, 12.5, 25, 50, 100 and 200 μM) by incubating them with ES-2-Rluc cell cultures for 2, 12 and 24 h, respectively. All probes showed almost no cytotoxicity within a 2-fold experimental concentration and two hours (Fig. S2†). The survival rate declined with the extension of incubation time, but the cytotoxicity of probes had almost no effect on the experimental results during the experimental period.
Moreover, we evaluated the cell viability of cobalt chloride at various concentrations (7.8, 15.6, 31.2, 62.5, 125, 250 and 500 μM) and time points (6, 12 and 24 h). Fig. S3† shows that cobalt chloride exhibited no apparent cytotoxicity to ES-2-Rluc cells below 500 μM. However, the cytotoxicity enhanced with the increasing concentration of CoCl2 and the extension of incubation time. We proposed that when the concentration of CoCl2 was above a certain concentration, the bioluminescence signal was declined (Fig. 2). Even if the cytotoxicity of probes could have occurred, the experimental results were not affected by CoCl2 within six hours.
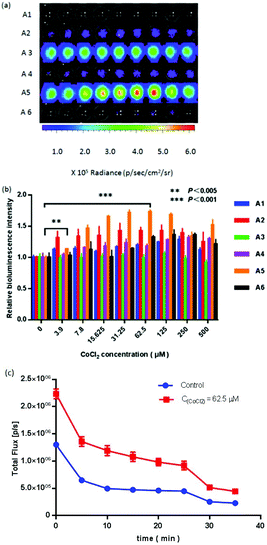 |
| Fig. 2 Bioluminescence imaging of the nitroreductase activities in ES-2-Rluc cells by probes. (a) Bioluminescence imaging of living cells stimulated with various concentrations (0, 3.9, 7.8, 15.6, 31.2, 62.5, 125, 250, and 500 μM) of CoCl2; (b) relative bioluminescence responses of probes A1–A6; (c) time-dependent bioluminescence imaging of cells with the concentration of cobalt chloride at 0 and 62.5 μM by using probe A5 (0.1 mL, 100 μM). | |
Bioluminescence imaging in cellulo
To determine whether the probes could detect NTR in cellulo, experiments where probes responded to the intracellular NTR level were assessed. In this section, cobalt chloride was exploited to irritate Renilla luciferase-transfected ES-2 (ES-2-Rluc) to promote the NTR level.28,39 For probe A5 at 100 μM, the bioluminescence signal reached maximum (1.73-fold) with the CoCl2 concentration of 62.5 μM. In a certain concentration range of CoCl2, the bioluminescence intensities of probes were enhanced and reached the peak. Then they were reduced (Fig. 2). As the signal decreased, the concentration of CoCl2 increased, and we deduced that the cell viability decreased with the growth of CoCl2 (Fig. S2†). Time-dependent bioluminescence imaging of cells by using probe A5 clearly expressed that the bioluminescence intensity transiently reached the maximum peak, and then gradually reduced to the plateau phase (Fig. 2C), which demonstrated that such a probe could timely and accurately detect endogenous NTR. The results confirmed our surmise that the NTR level can be elevated while CoCl2 stimulated cell cultures, and demonstrate that probes are able to detect the quantity of NTR in cellulo. The effect of the probes on the cell viability was examined by the MTT method (Fig. S3).† It showed that probes did not exhibit obvious cytotoxicity at less than 200 μM. In terms of sensitivity, probe A5 is better than others. We put forward that it is easier for probe A5 to enter the cell due to the ester water partition coefficient of probe A5, and probe A5 was more adequate for detecting endogenous NTR in mammals.
In vivo imaging
Subsequently, the probes were examined to detect the level of NTR overexpression in vivo. An ovarian cancer mouse model was constructed by orthotopically implanting in 4-week-old nude mice ES-2-Rluc cells that express Renilla luciferase tumor tissue. The mice were not ready for experiments until the tumors grew to reach a 1.2 cm diameter over 4 weeks. In this part of the experiment, dicoumarol was used to inhibit NTR activity.28,39 The nude mice were divided into the sodium chloride group (N.S.) and the dicoumarol group (Dicoumarol). The dicoumarol group was injected with dicoumarol (0.1 mL, 10 mM, DMSO
:
saline = 1
:
3) and the N.S. group was injected with 0.1 mL 0.9% sodium chloride solution. After 4 hours, probes (0.1 mL, 10 mM) were injected into the tumors. A bioluminescence signal was immediately acquired every 5 min (Fig. 3), in which probe A5 was confirmed as a highly selective and sensitive probe for detecting endogenous NTR in mammals. The bioluminescence signal was raised to around 6-fold in the tumour region for probe A5 compared with the control group. After 50 min, the N.S. group bioluminescence imaging signal was reduced to the Dicoumarol group imaging signal at 0 min. In addition, the signal intensity of the dicoumarol group was the last to decrease, so the time-dependent signal intensity was collected within 20 minutes. Based on these results, it was established that they could be applied to detect endogenous NTR in vivo. Probe 5 is a favourable probe for NTR; this is consistent with an experiment in cellulo.
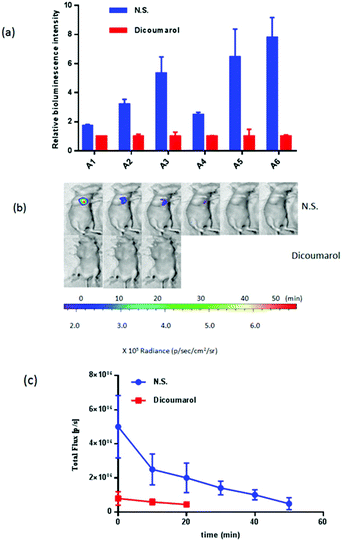 |
| Fig. 3 Bioluminescence imaging of endogenous nitroreductase activities in solid tumours. (a) Relative bioluminescence responses of probes A1–A6 (0.1 mL, 10 mM) with or without the injection of dicoumarol; (b) time-dependent bioluminescence imaging of solid tumors with or without dicoumarol by using probe A5 (0.1 mL, 10 mM); (c) bioluminescence signal of (b). | |
Experimental
Materials and apparatus
NMR spectral data were obtained for 1H NMR and 13C NMR at 500 and 500 MHz, respectively. Chemical shifts are reported in ppm from TMS as the internal reference with the solvent resonance in DMSO-d6 solution. ESI-HRMS spectral data were obtained by using a Water SYNAPTG2 mass spectrometer. HRMS was performed on a Waters SYNAPTG 2 mass spectrometer. Column chromatography was performed on silica gel using the eluents EtOAc and petroleum ether. Glass-backed silica plates were used to prepare the reaction solution, and monitored by using a ZF-1 UV detector. Melting points were obtained from an RY-1 melting point instrument. High performance liquid chromatography (HPLC) tests were carried out with an Agilent Technologies 1260 liquid chromatography system, C18 column (5 μm, 4.6 mm, 250 mm). All materials are commercially available.
A bioluminescence signal was acquired by using an IVIS Kinetic imaging system (Caliper Life Sciences, USA). A cooled charge-coupled device (CCD) camera was equipped with it. The data were quantified in a circular region of signal (ROI) as total photon flux in photons per second. Renilla luciferase was supplied by RayBiotech. ES-2-Rluc cells (human ovarian cancer cell line stably expressing Renilla luciferase) were purchased from Shanghai BioDiagnosis Co., Ltd. Female nude mice (BALB/cA-nu) were purchased from the Animal Center of China Academy of Medical Sciences (Beijing, China). All animal studies were approved by the Ethics Committee and IACUC of Cheeloo College of Medicine, Shandong University, and were conducted in compliance with the European guidelines for the care and use of laboratory animals.
Synthesis
General synthetic method of probe (Scheme S1†): to a mixture of B1 (14 mg, 0.035 mmol), p-nitrobenzyl bromide (23 mg, 0.107 mmol) and K2CO3 (14.76 mg, 0.107 mmol) was added a solution of acetone (5 mL). The solution mixture was stirred at room temperature for 8 hours under the argon protection. After 8 hours, to the reaction mixture was added water (10 mL) and extracted with EtOAc (10 mL, twice). The organic phase was combined and dried with MgSO4 (5 g). After 2 hours, the solution was filtered and concentrated. The filtrate was purified by chromatography on silica gel (200–300 mesh), and then the eluent was steamed out to give the white solid A1 (17.8 mg, 94.88%).
Selectivity and sensitivity assay in vitro
At 37 °C, sensitivity experiments were carried out in 50 mM Tris-HCl buffer, at physiological pH with 0.5 mM NADH. The probe solutions (50 μM) were added to a 96-well plate. To each well was added 50 μL of the solution. Then, 50 μL of different concentrations of NTR solutions (0–10 μg mL−1) were added to each well, and 50 μL of Renilla luciferase containing 0.5 mM NADH was immediately added. A bioluminescence signal was acquired by using an IVIS Kinetic imaging system (Caliper Life Sciences, USA). A cooled charge-coupled device (CCD) camera was equipped with it. The data were quantified in a circular region of signal (ROI) as total photon flux in photons per second and exposure time was 10 s. The bioluminescence intensity was calculated by dividing the experimental value by the control value, in which buffer was added instead of nitroreductase.
After adding different concentrations of NTR and Renilla luciferase, bioluminescence was immediately acquired with incubation for 1 h (Fig. S1†). The bioluminescence intensity of probe A1 was higher. The detection limit was 0.156 μg mL−1 NTR for probe A1. Even at an NTR concentration of 0.156 μg mL−1, the bioluminescence intensity of probe A1 was around 1.5 times over the control group. As P < 0.05, it was a significant change. Therefore, probe A1 was shown to be a potent toolkit to detect nitroreductase.
Cytotoxicity evaluation
The MTT method was performed to assess the cytotoxicity of probes to cells. The various concentrations of probes were incubated with ES-2-Rluc cell cultures ranging from 6.25, 12.5, 25, 50, 100 and 200 μM. The different incubation times that have been evaluated were2, 12 and 24 h, respectively.
The MTT method was also performed to assess the cytotoxicity of cobalt chloride on cell viability. Cobalt chloride was incubated with ES-2-Rluc cell cultures for 6, 12 and 24 h at different concentrations ranging from 7.8, 15.6, 31.2, 62.5, 125, 250 and 500 μM, respectively.
Selectivity and sensitivity test in cells
Into each well (96-well plate, Corning, 3603) were added 4 × 104 cells that were suspended in 100 μL medium. The plates were incubated in an incubator for 24 h. Then, the medium of each well was discarded, a new medium containing no FBS, which dissolved different concentrations of CoCl2 was added to each well. The concentrations of CoCl2 were 0, 7.8, 15.6, 31.25, 62.5, 125, 250 and 500 μM, respectively. Subsequently, the plates were incubated in an incubator for 6 h. Then, the CoCl2 medium was discarded and to each well was added the solution of probes (100 μL) dissolved in saline solution containing 1% DMSO. The bioluminescence imaging was immediately carried out; the exposure time was 5 min.
Animal test
The probes were examined to detect the level of NTR overexpression in solid tumours. The experiments of the mice model were performed. Every nude mouse was grafted with around 1 × 106 ES-2-Rluc cell lines. After 4 weeks, solid tumours with a diameter of about 1.2 cm were formed. These solid tumours were mashed into a homogenate. The new nude mice were grafted with different amounts of the tumour homogenate. These nude mice were divided into the sodium chloride group and dicoumarol group after 4 weeks. The control group was injected with dicoumarol (0.1 mL, 10 mM, DMSO
:
saline = 1
:
3) and the sodium chloride group was injected with 0.1 mL sodium chloride solution. 4 hours later, 0.1 mL of probe solutions (0.1 mL, 10 mM, DMSO
:
saline = 4
:
1) were injected into tumours, respectively. The bioluminescence signal was acquired immediately after the injection; the exposure time was 5 min.
Conclusions
In summary, a novel specific and sensitive bioluminescent probe for nitroreductase has been well developed herein. The probes can monitor NTR by the enzyme-catalyzed reduction of nitrobenzyl moiety, and then undergo a 1,4 or 1,6-rearrangement-elimination reaction to release coelenterazine analogues. It should be noted that the probe is not only able to detect the NTR quantity, but also able to determine the hypoxic status in cellulo and in vivo. As a result, our probes have suitable properties such as rapid response, high specificity and noninvasiveness, hence they can be applied as a potential bioanalytical toolkit for detecting NTR in vitro and in vivo.
Conflicts of interest
There are no conflicts to declare.
Acknowledgements
This work was supported by grants from the National Program on Key Basic Research Project (no. 2013CB734000), the National Natural Science Foundation of China (no. 81673393), the Taishan Scholar Program at Shandong Province, the Qilu Scholar Program at Shandong University and the Major Project of Science and Technology of Shandong Province (no. 2015ZDJS04001).
Notes and references
- S. Kizaka-Kondoh, M. Inoue, H. Harada and M. Hiraoka, Cancer Sci., 2003, 94, 1021–1028 CrossRef CAS PubMed.
- A. L. Harris, Nat. Rev. Cancer, 2002, 2, 38 CrossRef CAS PubMed.
- C. Murdoch, M. Muthana and C. E. Lewis, J. Immunol., 2005, 175, 6257–6263 CrossRef CAS.
- L. Cui, Y. Zhong, W. Zhu, Y. Xu, Q. Du, X. Wang, X. Qian and Y. Xiao, Org. Lett., 2011, 13, 928 CrossRef CAS PubMed.
- K. J. Williams, M. R. Albertella, B. Fitzpatrick, P. M. Loadman, S. D. Shnyder, E. C. Chinje, B. A. Telfer, C. R. Dunk, P. A. Harris and I. J. Stratford, Mol. Cancer Ther., 2009, 8, 3266–3275 CrossRef CAS PubMed.
- E. T. Shinohara and A. Maity, Curr. Mol. Med., 2009, 9, 1034–1045 CrossRef CAS PubMed.
- M. Hockel, K. Schlenger, B. Aral, M. Mitze, U. Schaffer and P. Vaupel, Cancer Res., 1996, 56, 4509–4515 CAS.
- E. K. Rofstad, H. Rasmussen, K. Galappathi, B. Mathiesen, K. Nilsen and B. A. Graff, Cancer Res., 2002, 62, 1847–1853 CAS.
- A. Fyles, M. Milosevic, M. Pintilie, A. Syed, W. Levin, L. Manchul and R. P. Hill, Radiother. Oncol., 2006, 80, 132–137 CrossRef PubMed.
- J. H. Kaanders, K. I. Wijffels, H. A. Marres, A. S. Ljungkvist, L. A. Pop, V. D. H. Fj, P. C. de Wilde, J. Bussink, J. A. Raleigh and V. D. K. Aj, Cancer Res., 2002, 62, 7066–7074 CAS.
- A. Daşu and J. Denekamp, Int. J. Radiat. Oncol., Biol., Phys., 1999, 43, 1083–1094 CrossRef.
- S. Stern and M. Guichard, Radiother. Oncol., 1996, 41, 143–149 CrossRef CAS PubMed.
- W. Zhu, M. Dai, Y. Xu and X. Qian, Bioorg. Med. Chem., 2008, 16, 3255–3260 CrossRef CAS PubMed.
- M. Dai, W. Zhu, Y. Xu, X. Qian, Y. Liu, Y. Xiao and Y. Yin, J. Fluoresc., 2008, 18, 591–597 CrossRef CAS PubMed.
- K. Tanabe, N. Hirata, H. Harada, M. Hiraoka and S. Nishimoto, ChemBioChem, 2008, 9, 426–432 CrossRef CAS PubMed.
- W. R. Wilson and M. P. Hay, Nat. Rev. Cancer, 2011, 11, 393–410 CrossRef CAS PubMed.
- K. Okuda, Y. Okabe, T. Kadonosono, T. Ueno, B. G. Youssif, S. Kizaka-Kondoh and H. Nagasawa, Bioconjugate Chem., 2012, 23, 324–329 CrossRef CAS PubMed.
- A. Celik and G. Yetiş, Bioorg. Med. Chem., 2012, 20, 3540–3550 CrossRef CAS PubMed.
- W. A. Denny, Lancet Oncol., 2000, 1, 25–29 CrossRef CAS PubMed.
- Y. Shi, S. Zhang and X. Zhang, Analyst, 2013, 138, 1952–1955 RSC.
- T. Guo, L. Cui, J. Shen, W. Zhu, Y. Xu and X. Qian, Chem. Commun., 2013, 49, 10820–10822 RSC.
- J. Yuan, Y. Q. Xu, N. N. Zhou, R. Wang, X. H. Qian and Y. F. Xu, RSC Adv., 2014, 4, 56207–56210 RSC.
- Z. Li, X. Li, X. Gao, Y. Zhang, W. Shi and H. Ma, Anal. Chem., 2013, 85, 3926 CrossRef CAS PubMed.
- J. Xu, S. Sun, Q. Li, Y. Yue, Y. Li and S. Shao, Analyst, 2015, 140, 574–581 RSC.
- K. Xu, F. Wang, X. Pan, R. Liu, J. Ma, F. Kong and B. Tang, Chem. Commun., 2013, 49, 2554–2556 RSC.
- B. Jungeun, L. E. Mcnamara, M. A. Nael, M. Fakhri, R. J. Doerksen, G. L. Bidwell, N. I. Hammer and J. Seongbong, Chem. Commun., 2015, 51, 12787–12790 RSC.
- J. Cao, J. Campbell, L. Liu, R. P. Mason and A. R. Lippert, Anal. Chem., 2016, 88, 4995–5002 CrossRef CAS PubMed.
- Y. Li, Y. Sun, J. Li, Q. Su, W. Yuan, Y. Dai, C. Han, Q. Wang, W. Feng and F. Li, J. Am. Chem. Soc., 2015, 137, 6407 CrossRef CAS PubMed.
- P. Feng, H. Zhang, Q. Deng, W. Liu, L. Yang, G. Li, G. Chen, L. Du, B. Ke and M. Li, Anal. Chem., 2016, 88, 5610–5614 CrossRef CAS PubMed.
-
Lighting up bioluminescence with coelenterazine: strategies and applications, ed. T. Jiang, L. Du and M. Li, 2016 Search PubMed.
- M. Yuan, X. Ma, T. Jiang, C. Zhang, H. Chen, Y. Gao, X. Yang, L. Du and M. Li, Org. Biomol. Chem., 2016, 14, 10267–10274 CAS.
- E. Lindberg, S. Mizukami, K. Ibata, T. Fukano, A. Miyawaki and K. Kikuchi, Chem. Sci., 2013, 4, 4395 RSC.
- R. Uchiyama and H. Tsutsui, Arch. Immunol. Ther. Exp., 2015, 63, 1–13 CrossRef CAS PubMed.
- S. Shalini, L. Dorstyn, S. Dawar and S. Kumar, Cell Death Differ., 2014, 22, 526 CrossRef PubMed.
- T. Jiang, X. Yang, Y. Zhou, I. Yampolsky, L. Du and M. Li, Org. Biomol. Chem., 2017, 15, 7008 CAS.
- J. M. Brown and W. R. Wilson, Nat. Rev. Cancer, 2004, 4, 437–447 CrossRef CAS PubMed.
- W. A. Denny, Eur. J. Med. Chem., 2001, 36, 577–595 CrossRef CAS PubMed.
- G. Xu and H. L. Mcleod, Clin. Cancer Res., 2001, 7, 3314 CAS.
- E. Johansson, G. N. Parkinson, W. A. Denny and S. Neidle, J. Med. Chem., 2003, 46, 4009–4020 CrossRef CAS PubMed.
Footnotes |
† Electronic supplementary information (ESI) available: The details of the preparation of the probes and their NMR and HR-MS spectra. See DOI: 10.1039/c7ob02618h |
‡ These authors contributed equally. |
|
This journal is © The Royal Society of Chemistry 2018 |
Click here to see how this site uses Cookies. View our privacy policy here.