DOI:
10.1039/C7RA13454A
(Paper)
RSC Adv., 2018,
8, 4624-4633
Albumin-assisted exfoliated ultrathin rhenium disulfide nanosheets as a tumor targeting and dual-stimuli-responsive drug delivery system for a combination chemo-photothermal treatment†
Received
19th December 2017
, Accepted 15th January 2018
First published on 25th January 2018
Abstract
Herein, we prepared an ultrathin rhenium disulfide nanosheet (utReS2) through the bovine serum albumin (BSA)-assisted ultrasonic exfoliation method, which showed great biocompatibility and high near-infrared (NIR) absorbance. The large surface specific area and the presence of BSA facilitate a high loading ratio and modification of multifunctional molecules. The low solubility anti-cancer drug resveratrol (RSV) was loaded onto the utReS2 surface to form a biocompatible nanocomposite (utReS2@RSV). A targeting molecule, folic acid (FA), was then conjugated to the BSA molecule of utReS2@RSV, resulting in utReS2@RSV–FA. The utReS2@RSV–FA exhibited a photothermal effect under an 808 nm laser irradiation. At pH = 6.5, about 16.5% of the RSV molecules was released from utReS2@RSV–FA over 24 h, while the value reached 55.3% after six cycles of NIR irradiation (5 min, 1 W cm−2). In vitro experiments of utReS2@RSV–FA showed that it had low cytotoxicity and an excellent HepG2 cells targeting effect. Upon pH/temperature dual-stimuli, utReS2@RSV–FA showed an enhanced cytotoxic effect. In vivo experiments of utReS2@RSV–FA intravenously injected into tumor-bearing mice showed that at 24 h post-injection, it could actively target and was largely accumulated in tumor tissue. When the injection was further accompanied by three cycles of NIR irradiation for 5 min, once a day, the tumor was efficiently suppressed, without relapse after 30 days. These findings demonstrate that utReS2@RSV–FA has a remarkable targeting ability while providing a dual-stimuli-responsive drug delivery system, and could effectively be used in a combination chemo-photothermal cancer treatment.
1. Introduction
Nowadays, cancer is undoubtedly one of the leading causes of deaths worldwide.1 Although various cancer treatment methods are available, such as surgery, radiotherapy, and chemotherapy, their disadvantages limit their therapeutic efficacy.2–4 For example, surgery has a potential hazard of organ malfunction and a risk of relapse when malignant cells are not completely removed;5 radiotherapy is radiotoxic to the nearby healthy tissues;6 and chemotherapy is toxic to nearby normal and fast dividing cells (e.g., hair loss) due to its non-specificity, as well as inherent leakages of drugs to nearby healthy tissues.7,8 In the last decades, a new and minimal invasive cancer treatment, photothermal therapy (PTT), has emerged. The therapy causes hyperthermia generated by photothermal agents (which absorb laser energy), leading to cancer cell death.9–13 PTT has been used as a promising alternative or as a supplementary treatment to the traditional cancer therapies. However, a number of PTT research studies reported that the use of PTT on its own often has a deficiency, such as incomplete tumor suppression, which could potentially generate a tumor relapse.14,15 Therefore, a more efficient tumor therapy strategy—simultaneous specific delivery of hyperthermia and chemotherapeutic drugs into tumor cells—has reportedly been able to overcome the shortcomings of single tumor PTT or chemotherapy. The strategy integrates multiple therapeutic abilities into a single nanoplatform.16,17
In addition to great biocompatibility and stability, high specific area and strong near infrared (NIR) absorbance are regarded as the most important factors for a chemo-photothermal treatment nanoplatform.18,19 Whilst high specific area allows the nanoplatform to have a higher drug loading ratio, strong NIR absorbance facilitates the nanoplatform with increased photothermal effect.20,21 To date, some nanomaterials, such as metal nanostructures, including noble metal-based materials (e.g., Au and Pd nanomaterials) and copper-based nanoparticles, have been shown to possess strong NIR absorbance capabilities and have promising photothermal therapeutic effects demonstrated by various in vitro and in vivo experiments.22–24 However, further applications of these nanomaterials are limited to their low drug ratio caused by their low specific area. These issues were overcome when two-dimensional (2D) nanomaterials, including 2D carbon nanomaterials (e.g., grapheme oxide and reduced grapheme oxide) and transition metal dichalcogenides (TMDCs) were developed. The materials were designed to have a large specific area and high intrinsic NIR absorbance, in addition to high drug loading, so that they can provide a more effective chemo-photothermal treatment.25–27
According to multiple recent research studies, a large variety of TMDCs, such as MoS2, MoSe2, WS2, TiS2, and Bi2Se3 nanosheets, have been explored and applied in biological sensing and imaging, drug delivery, and PTT.28,29 The exfoliated TMDCs (i.e., MoSe2, MoS2, WS2, and WSe2) have been reported to have lower cytotoxicity than the classic 2D materials (i.e., graphene and its analogues).30 A new family member to the 2D TMDCs, rhenium disulfide (ReS2) nanosheets have been shown to have a strong NIR absorbance and are great potential NIR photothermal transducers.31,32 Moreover, they have a large surface specific area, providing a potentially high loading ratio of the delivered drug to tumor cells.
Herein, we developed albumin-assisted exfoliated ultrathin rhenium disulfide nanosheets (utReS2) with great biocompatibility, large surface specific area, and modifiable surface. Resveratrol (RSV) is a natural molecule that has been proven to have cancer preventive and therapeutic activities without any potential side effects.33,34 In recent years, RSV has also been shown to have cytotoxic potential against liver cancer.35 However, the disadvantages of low solubility and systemic circulation time, and non-specificity limit the practical application of RSV.36,37 RSV was thus loaded onto the utReS2 surface (utReS2@RSV), which was then conjugated with a target molecule, folic acid (FA), to form a nanocomposite (utReS2@RSV–FA). In vitro and in vivo experiments demonstrated that the utReS2@RSV–FA had a high liver cancer targeting effect, and could be a pH/temperature dual-stimuli-responsive drug delivery system in a combination chemo-photothermal treatment (Fig. 1).
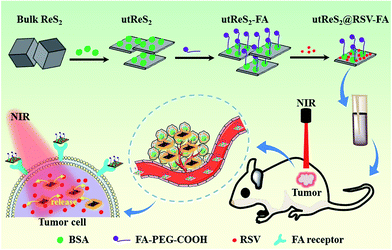 |
| Fig. 1 A schematic illustration of the utReS2@RSV–FA synthesis for tumor targeted chemo-photothermal therapy. | |
2. Experimental section
2.1 Materials and instruments
Rhenium disulfide (ReS2) powder, 1-ethyl-3-(3-dimethylaminopropyl)carbodiimide (EDC) and N-hydroxysuccinimide (NHS), bovine serum albumin (BSA, ≥98.0%) and fluorescein isothiocyanate (FITC) were purchased from Sigma-Aldrich. Dulbecco's Modified Eagle's Medium (DMEM), fetal bovine serum (FBS) and 4′,6′-diamidino-2-phenylindole (DAPI) were obtained from Invitrogen (Carlsbad, CA, USA).
Transmission electron microscopy (TEM) images were collected on a transmission electron microscope (SU8010, Hitachi, Japan). Atomic force microscopy (AFM) images were captured by an atomic force microscope (OMCL, Olympus, Japan). The size and zeta potential of nanoparticles was detected by a nanosizer (Malvern Instruments). The UV-vis absorption spectra were detected by a UV-vis spectrophotometer (UV-2550, Shimadzu, Japan). An inductively coupled plasma optical emission spectrometer (ICP-OES, iCAP 7000 Plus, Thermo Scientific, USA) was used to measure the Re element content.
2.2 Preparation of utReS2@RSV–FA
Firstly, 10 mg ReS2 powder was mixed with 20 mL water and stirred for 20 min. Afterwards, the mixture was first ice-bath ultra-sonic dissociated using a 500 W and 20 kHz tip sonication (SONICS, VCX130, USA) for 3 h, and then was ice-bath ultra-sonic dissociated (200 W, 20 kHz) in the presence of 10 mg BSA powder for 12 h. To remove large aggregates and superfluous reagents, the prepared mixture was centrifuged at 10
000 rpm for 10 min. The supernatant was collected and purified by high-speed centrifugation at 15
000 rpm for 20 min, resulting in utReS2.
Secondly, 20 mg NH2–PEG2000–FA dissolved in 1 mL ethanol solution was mixed with utReS2 solution and reacted for 3 h under the presence of 4 mM EDC and 10 mM NHS with stirring at room temperature and pH 6.0. To remove the unconjugated NH2–PEG2000–FA and redundant chemical reagents, the mixture was dialyzed in deionized water for 24 h, resulting in utReS2–FA.
Lastly, the RSV was dissolved in DMSO, and added into 10 mL utReS2–FA nanosheets water suspension with constant and slight stirring at 25 °C for 12 h. Unbound RSV was removed by dialyzing in deionized water for 24 h to give utReS2@RSV–FA nanosheets, which were stored at 4 °C. The RSV loading ratio was detected via monitoring of the absorption peak of RSV at 306 nm and calculated according to the equation:
where
Aa (mg),
Ab (mg) and
Ac (mg) respectively represent the initial, unbound RSV and the ReS
2 nanosheets (mg).
2.3 Cell culture and cell uptake assay
The human hepatic HepG2 cells were obtained from the Chinese Academy of Sciences Cell Bank of Type Culture Collection (CBTCCCAS, Shanghai, China). All cells were cultured in complete DMEM media (10% FBS + 90% DMEM) in a humidified incubator containing 5% CO2 at 37 °C.
Firstly, utReS2 nanosheets were labeled by FITC. 1 mg FITC was dissolved in 1 mL DMSO and mixed with the utReS2@RSV and utReS2@RSV–FA suspension with slight stirring at room temperature for 12 h. The mixture was dialyzed in deionized water for 24 h to remove the unbound FITC, resulting in purified FITC labeled utReS2@RSV and utReS2@RSV–FA (utReS2@RSV/FITC and utReS2@RSV–FA/FITC). HepG2 cells were cultured in six-well plates for 24 h and then incubated with free FITC, utReS2@RSV/FITC or utReS2@RSV–FA/FITC (with same FITC concentration) for 3 h. After thrice washing the cells with PBS, the cells were fixed by glutaraldehyde and stained with DAPI for 8 min. One part of the cells was used to observe the cellular fluorescence using the laser scanning microscope (LSM 510, Zeiss, Germany). The other part of the cells was collected for flow cytometry (FCM, EPICS XL, Beckman, USA) analysis to calculate the uptake ratios through counting of the cellular FITC fluorescence intensity (Ex = 488 nm).
2.4 In vitro biocompatibility
A standard CCK-8 assay (Bestbio, China) was first used to evaluate the cytotoxicity of utReS2–FA. HepG2 cells (1 × 105 cells per mL) were cultured in a 96-well plate for 24 h. After removing the old media, utReS2–FA (0.02, 0.05, 0.1, and 0.2 mg mL−1) was incubated with HepG2 cells for 24 h. Afterwards, the cells were washed with PBS thrice mildly. According to the protocol of the CCK-8 assay, a CCK-8 working solution (100 μL) was added to each well and incubated with cells at 37 °C for 30 min. Lastly, a microplate reader (EnVision, PerkinElmer, USA) was used to detect the absorbance value at 450 nm.
In addition, mouse red blood cells (RBCs) were collected and mixed with different concentrations of utReS2@RSV–FA (20, 50, 100, 200 and 400 μg mL−1). After incubating at 37 °C for 1 h, the treated RBCs above were centrifuged (10
000 rpm) for 1 min. RBCs incubated with deionized water and PBS were used as the positive and negative controls, respectively. The absorbance value at 541 nm of the supernatant was measured using a UV-vis spectroscopy. The hemolytic percentage (HP) was calculated according to the equation:
where
At,
Apc and
Anc are the absorbance of the test samples, and positive and negative controls, respectively.
2.5 In vitro tumor therapy
For in vitro tumor therapy, HepG2 cells (5 × 104 cells per well) were cultured in 96-well plates for 24 h. Then various concentrations of utReS2, RSV, and utReS2@RSV–FA were added into the cell wells and incubated with the cells for an extra 24 h. The cell viability was detected by CCK-8 assay as mentioned above. For in vitro PTT, various concentrations of utReS2, RSV, and utReS2@RSV–FA treated cells were irradiated using the 808 nm laser (1 W cm−2) for 5 min. The real-time temperature and thermal images (once every 30 seconds) of the cells were first recorded by the thermocouple thermometer (BAT-7001H, Physitemp, USA) and infrared thermal camera (TI25, FLUKE, USA), respectively. These cells were the continuously cultured for 24 h. The cell viabilities were also evaluated by the CCK-8 assay. Simultaneously, these cells were co-stained by calcein-AM/PI (Aladdin, Shanghai, China) for 30 min and then imaged using a confocal laser scanning microscope (calcein-AM Ex = 488 nm, PI Ex = 535 nm).
2.6 Animal model and in vivo biodistribution
To establish the HepG2 subcutaneous tumor model, 1 × 107 HepG2 cells (100 μL, in PBS) were subcutaneously injected into the back of Balb/c nude mice. The tumor volume was established by: tumor volume = length × width2/2. All animal procedures were performed in accordance with the Guidelines for Care and Use of Laboratory Animals of the National Institutes of Health (NIH publication no. 85-23, revised 1996) and approved by the Animal Ethics Committee of Southwest Medical University (Luzhou, China).
Biodistribution of utReS2@RSV and utReS2@RSV–FA in the tumor-bearing nude mice was detected at 1 h, 1 day, 2 days, 5 days and 7 days post tail intravenous injection with these samples (100 μL). The major organs, including the heart, liver, spleen, lung, kidney, and tumor, were weighed and digested by aqua regia solution. The Re element content in these tissues was quantified by ICP-OES.
2.7 In vivo anticancer efficacy and toxicity study
In the in vivo anticancer experiments, the tumor-bearing nude mice were randomly divided into six groups (n = 7): (1) PBS (100 μL); (2) PBS (100 μL) + NIR laser; (3) RSV (100 μL, 4 mg kg−1); (4) utReS2@RSV (100 μL, 2 mg kg−1) + NIR laser; (5) utReS2@RSV–FA and utReS2@RSV–FA (100 μL, 4 mg kg−1 RSV and 2 mg kg−1 ReS2) + NIR laser. After 24 h intravenous injection, the tumor region of the tumor bearing mice in these groups was irradiated by NIR laser (1 W cm−2, 5 min) once a day for three days. The temperature of the tumor region in the NIR irradiated groups was recorded. During the treatment, the tumor volume and body weight were recorded every three days. The relative tumor volume was calculated by: relative tumor volume = V/V0, in which V0 was the tumor volume when the tumor treatment was initiated.
For the in vivo toxicity study, healthy Balb/c mice were intravenously injected with utReS2@RSV–FA (10 mg kg−1) or an equal volume of saline. The major organs, including the lung, heart, liver, spleen, and kidney were harvested for histological analysis after 15 days. The H&E images were obtained using a digital camera. In addition, whole blood samples of these mice were collected at 1, 7 and 30 days after injection for blood analysis. The complete blood counts, including white blood cell count (WBC), red blood cell count (RBC), hemoglobin concentration (HGB), mean platelet volume (MPV), hematocrit (HCT), mean corpuscular volume (MCV), and mean corpuscular hemoglobin concentration (MCHC) were detected using a hemocytometer (Countess C10227, Invitrogen, USA).
3. Results and discussion
3.1 Synthesis and characterization of utReS2@RSV–FA
An observation by TEM showed that the utReS2@RSV–FA had a flake-like morphology with a lattice spacing of 0.23 nm (Fig. 2a and b), similar to the morphology of the utReS2 nanosheets (Fig. S1†), this indicates that it has an ultrathin structure. According to AFM analysis (Fig. 2c and d), the center thickness (∼7.6 nm) of utReS2@RSV–FA increased compared to the edge thickness (∼2 nm), which is likely due to BSA adhesion, RSV loading, and FA conjugation. The utReS2@RSV–FA had an average diameter of about 150 nm and an average zeta potential of −32 mV, as evaluated by a nanosizer (Fig. 2e and f).
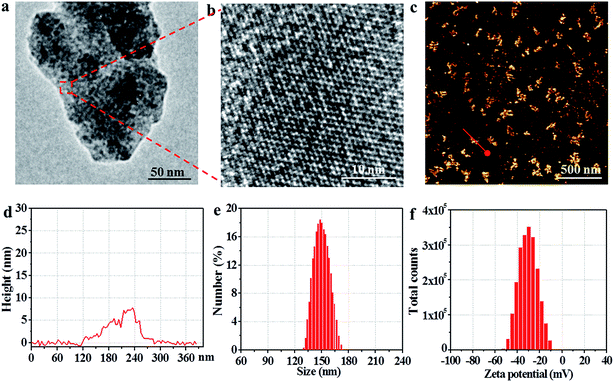 |
| Fig. 2 (a) The TEM image of utReS2@RSV–FA. (b) The high-resolution TEM image of the red dashed frame region in (a). (c) The typical AFM image of utReS2@RSV–FA. (d) The height profile of the red line region in (c). The (e) size and (f) zeta potential distribution of utReS2@RSV–FA. | |
As shown in Fig. 3a, compared with that of the bulk ReS2, the absorption spectrum of the BSA-assisted exfoliated utReS2 exhibited a new peak at 275 nm (originating from the BSA) (Fig. S2†), indicating the presence of BSA in utReS2. The absorption spectrum of the utReS2@RSV–FA (displayed in Fig. 3b) showed a peak associated with RSV at 306 nm and a high NIR absorbance, demonstrating that RSV was successfully loaded onto the utReS2 nanosheets. In addition, a significant fluorescence quenching was observed after RSV was loaded onto the utReS2 surface (Fig. 3c), indicating further interactions between utReS2 and RSV. As a 2D material that is similar to graphene,2 utReS2 nanosheets have large surface areas; they can therefore load and bind functional molecules through non-covalent interactions (e.g., π–π stacking and hydrophobic interactions). The loading capacities of utReS2 nanosheets increased with the increase of RSV concentrations (Fig. 3d), and reached the highest RSV loading ratio of ∼200% (w/w). Moreover, compared to utReS2, bulk ReS2 showed a lower drug loading ratio (∼45.6%) (Fig. 3d). The high drug loading ratio may be due to the large surface specific area and BSA adhesion of utReS2. Fig. 3e shows that the average sizes of utReS2@RSV–FA in water, FBS, cell media, PBS, and saline were almost unchanged over 7 days, suggesting that utReS2@RSV–FA has a high stability, possibly due to BSA adhesion and PEG conjugation on the utReS2 surface. According to the literature, RSV has two natural isomers, cis and trans (trans has a higher bioactivity and stability than the cis configurations), which exhibit featured absorbance peaks at 280 and 304 nm, respectively.38 In this regard, the change of ratio of A304 nm to A280 nm (A304 nm/A280 nm) represents the interconversion between the two isomers. As illustrated in Fig. 3f, the A304 nm/A280 nm ratio was nearly unchanged for 7 days, demonstrating that RSV has a trans-configuration and remains stable in utReS2@RSV–FA.
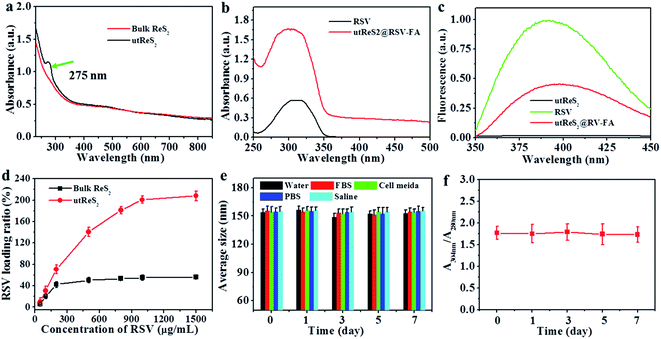 |
| Fig. 3 (a) The absorption spectra of the bulk ReS2 and utReS2 nanosheets. (b) The absorption spectra of RSV and utReS2@RSV–FA. (c) The fluorescence spectra of utReS2, RSV and utReS2@RSV–FA. (d) RSV loading ratio onto bulk ReS2 and utReS2 as the function of added RSV concentrations. (e) The average size change of utReS2@RSV–FA in water, FBS, cell media, PBS and saline over 7 days. (f) The absorbance ratio change of utReS2@RSV–FA between 304 nm and 280 nm (A304 nm/A280 nm) over 7 days. | |
Fig. 4a shows the schematic illustration of the pH/temperature stimuli-responsive RSV release. As shown in Fig. 4b, the temperature of the utReS2@RSV–FA solution was concentration-dependent, varying with different concentrations (0–200 ppm) upon an 808 nm irradiation (1 W cm−2, 5 min). The utReS2@RSV–FA of 200 ppm irradiated with NIR reached a maximum temperature of ∼60 °C. After five cycles of NIR irradiation, the initial photothermal effects of utReS2@RSV–FA remained (Fig. 4c). Moreover, its absorbance spectra before and after five cycles of NIR irradiation were similar (Fig. S3†). According to the literature method,9 the photothermal conversion efficiency of utReS2@RSV–FA was calculated to be 45.1%. These results indicate the utReS2@RSV–FA has great photostability and an excellent photothermal effect. Fig. S4† shows that normal and inner tumor tissues have a pH of about 7.4 and 6.5, respectively. The pH in cellular lysosome is also acidic. Fig. 4d shows the RSV release ratio in response to various pH and irradiation conditions. The 24 h release of RSV from utReS2@RSV–FA was 7.6% at a physiological pH of 7.4, while that was significantly increased to 16.5% at pH 6.5. With the decrease of the pH value (pH = 7.4–3.0), the cumulative released RSV increased obviously (Fig. S5†). In addition, with irradiation for 5 min (808 nm, 1 W cm−2), the RSV release was about 25.1% at pH 7.4 (Fig. S6†), indicating that weak acid conditions and photothermal effects could promote the RSV release. At pH 6.5, and irradiation (808 nm, 1 W cm−2) for 5 min, the RSV release was significantly increased to 55.3%, which was much higher than that without irradiation. These results indicate that the weak acidic environment in tumors and the controllable external photothermal effect could be used as a dual-stimuli controlling an on/off release of RSV from utReS2@RSV–FA. RSV was released from the utReS2 surface due to heat generated by utReS2 upon absorption of NIR light, and such heat could weaken the non-covalent adsorption interactions between RSV and utReS2 surface.3 In addition, in an acidic environment, the H+ could change the surface charge of utReS2, altering the hydrophilic/hydrophobic balance of the nanoparticles.39–41
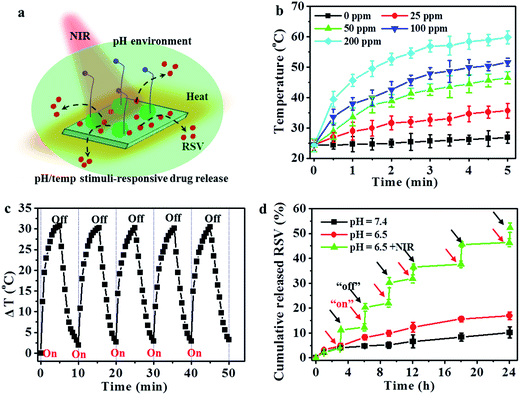 |
| Fig. 4 (a) The schematic illustration of pH/temperature (pH/temp) stimuli-responsive drug release. (b) Temperature curves of the utReS2@RSV–FA solution (0, 25, 50, 100 and 200 ppm) under laser irradiation (5 min, 808 nm, 1 W cm−2). (c) Temperature changes of utReS2@RSV–FA after 5 cycles of irradiations (5 min, 808 nm, 1 W cm−2). (d) Release kinetics of RSV from utReS2@RSV–FA in pH = 7.4 and 6.5 with or without NIR laser irradiation, respectively. | |
3.2 In vitro cell uptake and biocompatibility
The utReS2@RSV–FA could target HepG2 cells through the FA receptor (Fig. 5a). Fluorescein isothiocyanate was used to label the utReS2@RSV–FA, and its fluorescence signal was observed through a fluorescence microscope. As shown in Fig. 5b, utReS2@RSV–FA-treated cells had a stronger green FITC fluorescence in the cytoplasm than the utReS2@RSV- and free-FITC-treated cells. After FA blocking, the utReS2@RSV–FA-treated cells showed weaker green FITC fluorescence inside the cytoplasm, indicating that the FA receptor on the cell membrane is hindered (by free FA), in turn, this reduces the targeting ability and accessibility of utReS2@RSV–FA. The quantitative cellular uptake ratio of utReS2@RSV–FA according to FCM was about 61.1%, which was higher than that of free FITC (2.1%), utReS2@RSV (23.5%), and utReS2@RSV–FA + FA (19.7%) (Fig. 5c). The results demonstrated that the FA conjugation could promote the cell internalization of utReS2@RSV–FA through the receptor-mediated endocytosis.42
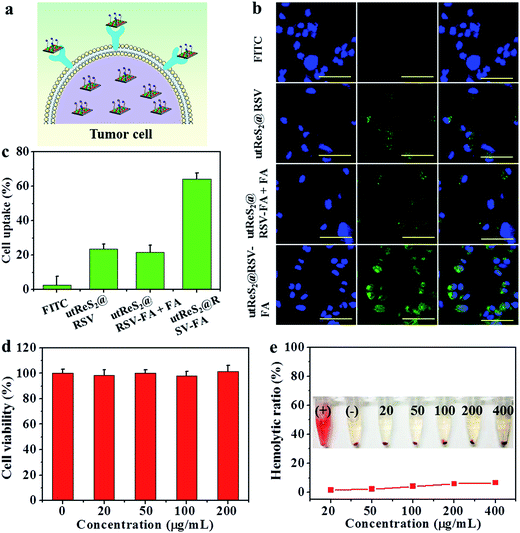 |
| Fig. 5 (a) The schematic illustration of utReS2@RSV–FA target to tumor cell mediated by the FA receptor. (b) Fluorescence images of HepG2 cells after incubation with free FITC and utReS2@RSV, utReS2@RSV–FA + FA and utReS2@RSV–FA (labeled with FITC). Green and blue colors are FITC and DAPI fluorescence, respectively. Scale bar = 20 μm. (c) Flow cytometry quantitative results of cellular FITC fluorescence in HepG2 cells. (d) In vitro cytotoxicity against HepG2 cells treated with different concentrations of ReS2–FA. (e) Hemolytic ratio of RBCs after 1 hour incubation with utReS2@RSV–FA at different concentrations. The inset shows the photograph of water (+), PBS (−), and different concentrations of utReS2@RSV–FA treated RBCs. | |
In vitro biocompatibility of utReS2–FA, as a drug carrier, was evaluated by cell viability and hemolysis of red blood cells (RBCs). As shown in Fig. 5d, the viabilities of the HepG2 cells treated with 0–200 μg mL−1 were more than 95%, indicating that utReS2–FA has a very low cytotoxicity. Fig. 5e illustrates that the hemolysis ratio of utReS2–FA at a concentration range of 20 to 400 μg mL−1 was similar to that of the negative control, suggesting that the utReS2–FA has good hemocompatibility, which could be ascribed to the conjugated BSA and PEG in utReS2.
3.3 In vitro tumor therapy
Fig. 6a shows the temperature of adherent HepG2 cells incubated with PBS, utReS2, utReS2@RSV, and utReS2@RSV–FA solutions (50 μg mL−1 with respect to utReS2) under an 808 nm irradiation (1.0 W cm−2) for 5 min. The results showed that cells treated with utReS2@RSV–FA had the highest temperature increase (ΔT = 25 °C) compared to utReS2, utReS2@RSV, and PBS-treated cells (Fig. 6b). The viabilities of cells treated with various concentrations of utReS2, RSV, utReS2@RSV, and utReS2@RSV–FA for 24 h without NIR irradiation were concentration-dependent, the viability decreased with increasing concentration (except for utReS2). The highest cell viability of 32.1 ± 1.2% was observed in utReS2@RSV–FA-treated cells (Fig. 6c). In contrast, significant concentration-dependent cell deaths were observed in cells treated with utReS2, utReS2@RSV, and utReS2@RSV–FA under a NIR irradiation (1.0 W cm−2, 5 min) (Fig. 6d). The effect was particularly significant in utReS2@RSV–FA-treated cells. It is possible that such in vitro PTT effect is due to FA targeting, which promotes the internalization of utReS2@RSV–FA, thus generating more heat upon NIR irradiation, releasing more drugs, and enhancing the cytotoxicity effects.
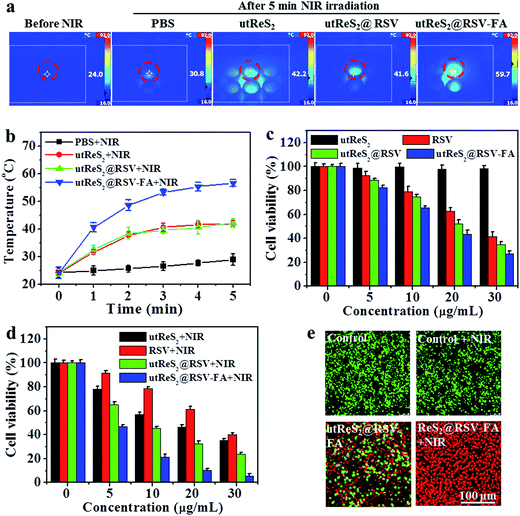 |
| Fig. 6 (a) Thermal images of PBS, utReS2, utReS2@RSV and utReS2@RSV–FA treated cells in 96-well plates after 5 min NIR irradiation, and (b) the corresponding temperature change curves. (c and d) Viability of the cells treated with RSV, utReS2, utReS2@RSV and utReS2@RSV–FA with or without 808 nm laser irradiation (5 min, 1 W cm−2). (e) The calcium AM/PI dual-staining images of cells after treatment by control (PBS), control + NIR, utReS2@RSV–FA and utReS2@RSV–FA + NIR, respectively. | |
Furthermore, calcein-AM/PI dual staining was further used to investigate the cytotoxic effect of utReS2@RSV–FA with or without NIR irradiation. Both the control and the control + NIR cells exhibited green fluorescence, indicating that the laser irradiation has no effects on the cells (Fig. 6e). Cells treated with utReS2@RSV–FA without irradiation were partially dead, exhibiting red fluorescence, whereas those with irradiation were almost completely dead (Fig. 6e). The results corresponded with those from the CCK-8 assay (Fig. 6d).
3.4 In vivo biodistribution and chemo-photothermal combination treatment
Understanding the biodistribution of nanoparticles in major organs (i.e., heart, liver, spleen, lung, and kidney) as well as tumors can guide in vivo combination chemo-photothermal cancer treatments. At 24 h post-injection, tumor-bearing mice intravenously injected with utReS2@RSV–FA exhibited higher accumulation (of the corresponding injected nanoparticles in tumor cells) than those injected with utReS2@RSV (Fig. 7a). The nanoparticles were accumulated in liver and spleen, in addition to tumor (Fig. 7b), suggesting that the nanoparticles may be metabolized in the liver and kidney. These observations suggest that the FA targeting enhances the accumulation of nanoparticles in the tumor.
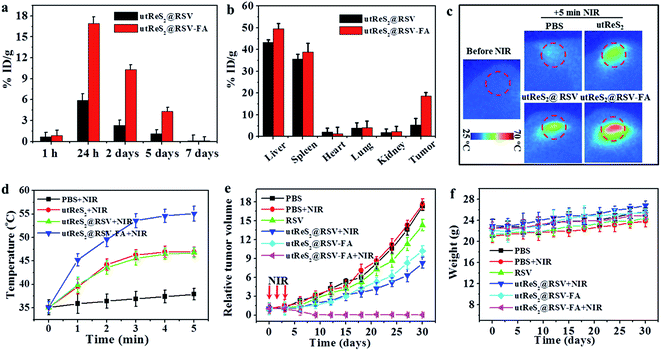 |
| Fig. 7 (a) Biodistribution of the nanoparticles in the tumor tissue post-injection with utReS2@RSV and utReS2@RSV–FA. (b) Biodistribution of the nanoparticles in the major organs (i.e., heart, liver, spleen, lung, kidney) as well as tumors at 24 h post-injection with utReS2@RSV and utReS2@RSV–FA. (c) The thermal images of tumor-bearing mice at 24 h post-injection of PBS, utReS2, utReS2@RSV and utReS2@RSV–FA under 5 min NIR irradiation (808 nm, 1 W cm−2), respectively. (d) The temperature statistical results of tumor regions of tumor-bearing mice post tail vein injection of PBS, utReS2, utReS2@RSV and utReS2@RSV–FA at 24 h under 5 min NIR irradiation (808 nm, 1 W cm−2), respectively. (e) The growth profile of HepG2 tumors after intravenous injection of PBS, RSV, utReS2@RSV and utReS2@RSV–FA with or without three cycles of NIR irradiation (5 min, 808 nm, 1 W cm−2) once a day. (f) Body weight of tumor-bearing mice during 30 days of treatment. | |
In addition, Fig. 7c shows the tumor temperature of tumor-bearing mice intravenously injected with PBS, utReS2, utReS2@RSV, and utReS2@RSV–FA at 24 h post-injection under an 808 nm laser irradiation (1 W cm−2) for 5 min. The highest temperature increase (by about 21 °C) was observed in utReS2@RSV–FA-treated tumor regions. After three cycles of NIR irradiation (once a day) and subsequent treatment for about one month, the groups treated with PBS, PBS + NIR, RSV, utReS2@RSV + NIR, and utReS2@RSV–FA exhibited no clear indication of tumor suppression. As expected, the utReS2@RSV–FA + NIR laser group exhibited significant tumor growth suppression without a relapse (Fig. 7e). During the treatment, the decrease of mice's body weight was not significant, indicating that the injection dose was within a biosafe range. These observations illustrate that the utReS2@RSV–FA with FA targeting and pH/temperature dual-stimuli RSV release possessed a high anticancer effect in the combination chemo-photothermal treatment.
3.5 In vivo toxicity study
In vivo toxicity of utReS2@RSV–FA was further evaluated. Healthy Balb/c mice were intravenously injected with utReS2@RSV–FA (10 mg kg−1) or an equal volume of saline (control). Major organs, including the lung, heart, liver, spleen, and kidney, were harvested for histological analysis after 15 days. As shown in Fig. 8a, the H&E stained sections of all mice (both utReS2@RSV–FA-injected and control) showed that there was no noticeable organ damage. Moreover, whole blood samples of these mice were collected at 1, 7, and 30 days post-injection for blood analysis. As illustrated in Fig. 8b and c, the complete blood counts (WBC, RBC, HGB, MPV, HCT, MCV, and MCHC) of the utReS2@RSV–FA-injected mice were within the normal ranges, and were not significantly different from the control. The results demonstrate that the utReS2@RSV–FA has no significant cytotoxicity in vivo, which indicates its excellent biocompatibility.
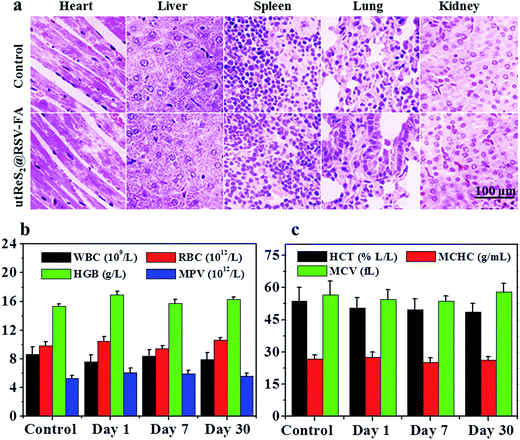 |
| Fig. 8 (a) H&E-stained images of heart, liver, spleen, lung, and kidney from mice treated with saline (control) or utReS2@RSV–FA at days 30. (b and c) Blood cells count of mice at days 1, 7 and 30 post-injection of saline (control) and utReS2@RSV–FA. | |
4. Conclusions
In summary, we prepared utReS2, a new family member of TMDCs, as a tumor targeting and dual-stimuli-responsive drug delivery system in a combination chemo-photothermal treatment. The utReS2, prepared by a facile albumin-assisted exfoliation method, exhibited high stability in aqueous media and great biocompatibility, in addition to excellent NIR absorbance and large surface area. It could thus induce a high photothermal effect and increase the drug-loading ratio via a non-covalent interaction. The utReS2@RSV–FA possessed a controllable drug release in response to heat induced by a NIR laser irradiation and the endogenous weak acidic conditions of tumor tissue. FA targeting promoted the accumulation of utReS2@RSV–FA in tumor cells both in vitro and in vivo. The tumor-targeted utReS2@RSV–FA accompanied with NIR-mediated hyperthermia and pH/temperature-triggered RSV release showed a remarkable synergistic inhibition of tumor growth without a relapse both in vitro and in vivo. The results also confirmed that utReS2@RSV–FA was not toxic to healthy tissues within the experimental dosage. These findings demonstrate that the utReS2@RSV–FA prepared in this study could be a potential and high-efficiency nanoplatform for cancer therapy.
Conflicts of interest
The authors report no conflicts of interest in this work.
Acknowledgements
This work was supported by the fund of the Sichuan Provincial Science and Technology Department – Luzhou People's Government – Luzhou Medical College Joint Research Project [2014] No. 10.
References
- Y. Liu, K. Ai, J. Liu, M. Deng, Y. He and L. Lu, Adv. Mater., 2013, 25, 1353–1359 CrossRef CAS PubMed.
- J. Chen, H. Liu, C. Zhao, G. Qin, G. Xi, T. Li, X. Wang and T. Chen, Biomaterials, 2014, 35, 4986–4995 CrossRef CAS PubMed.
- R. Deng, H. Yi, F. Fan, L. Fu, Y. Zeng, Y. Wang, Y. Li, S. Ji and Y. Su, RSC Adv., 2016, 6, 77083–77092 RSC.
- F. M. Kievit and M. Zhang, Acc. Chem. Res., 2011, 44, 853–862 CrossRef CAS PubMed.
- D. S. Chauhan, S. Indulekha, R. Gottipalli, B. P. K. Reddy, T. R. Chikate, R. Gupta, D. N. Jahagirdar, R. Prasad, A. De and R. Srivastava, RSC Adv., 2017, 7, 44026–44034 RSC.
- H. Yue, J. Qian, V. M. Elner, J. Guo, Y. F. Yuan, R. Zhang and Q. Ge, Br. J. Ophthalmol., 2013, 97, 739–745 CrossRef PubMed.
- M. L. Mancini and S. T. Sonis, Front. Pharmacol., 2014, 5, 51 Search PubMed.
- A. Maji and D. Sen, Curr. Cancer Ther. Rev., 2017, 13, 43–62 CAS.
- J. Chen, X. Wang and T. Chen, Nanoscale Res. Lett., 2014, 9, 86 CrossRef PubMed.
- X. Song, H. Gong, S. Yin, L. Cheng, C. Wang, Z. Li, Y. Li, X. Wang, G. Liu and Z. Liu, Adv. Funct. Mater., 2014, 24, 1194–1201 CrossRef CAS.
- P. Huang, J. Lin, W. Li, P. Rong, Z. Wang, S. Wang, X. Wang, X. Sun, M. Aronova, G. Niu, R. D. Leapman, Z. Nie and X. Chen, Angew. Chem., Int. Ed., 2013, 125, 14208–14214 CrossRef.
- Q. Chen, C. Wang, L. Cheng, W. He, Z. Cheng and Z. Liu, Biomaterials, 2014, 35, 2915–2923 CrossRef CAS PubMed.
- M. A. Mackey, M. R. K. Ali, L. A. Austin, R. D. Near and M. A. El-Sayed, J. Phys. Chem. B, 2014, 118, 1319–1326 CrossRef CAS PubMed.
- N. Kuthala, R. Vankayala, Y. N. Li, C. S. Chiang and K. C. Hwang, Adv. Mater., 2017, 29, 1700850 CrossRef PubMed.
- C. Ayala-Orozco, C. Urban, S. Bishnoi, A. Urban, H. Charron, T. Mitchell, M. Shea, S. Nanda, R. Schiff, N. Halas and A. Joshi, J. Controlled Release, 2014, 191, 90–97 CrossRef CAS PubMed.
- S. Shen, H. Tang, X. Zhang, J. Ren, Z. Pang, D. Wang, H. Gao, Y. Qian, X. Jiang and W. Yang, Biomaterials, 2013, 34, 3150–3158 CrossRef CAS PubMed.
- X. Wang, Q. Zhang, L. Zou, H. Hu, M. Zhang and J. Dai, RSC Adv., 2016, 6, 20949–20960 RSC.
- J. T. Robinson, S. M. Tabakman, Y. Liang, H. Wang, H. S. Casalongue, D. Vinh and H. Dai, J. Am. Chem. Soc., 2011, 133, 6825–6831 CrossRef CAS PubMed.
- W. Yin, L. Yan, J. Yu, G. Tian, L. Zhou, X. Zheng, X. Zhang, Y. Yong, J. Li, Z. Gu and Y. Zhao, ACS Nano, 2014, 8, 6922–6933 CrossRef CAS PubMed.
- X. Sun, Z. Liu, K. Welsher, J. T. Robinson, A. Goodwin, S. Zaric and H. Dai, Nano Res., 2008, 1, 203–212 CrossRef CAS PubMed.
- D. P. O'Neal, L. R. Hirsch, N. J. Halas, J. D. Payne and J. L. West, Cancer Lett., 2004, 209, 171–176 CrossRef PubMed.
- W. Fang, S. Tang, P. Liu, X. Fang, J. Gong and N. Zheng, Small, 2012, 8, 3816–3822 CrossRef CAS PubMed.
- M. Chen, S. Tang, Z. Guo, X. Wang, S. Mo, X. Huang, G. Liu and N. Zheng, Adv. Mater., 2014, 26, 8210–8216 CrossRef CAS PubMed.
- M. Everts, V. Saini, J. L. Leddon, R. J. Kok, M. Stoff-Khalili, M. A. Preuss, C. L. Millican, G. Perkins, J. M. Brown, H. Bagaria, D. E. Nikles, D. T. Johnson, V. P. Zharov and D. T. Curiel, Nano Lett., 2006, 6, 587–591 CrossRef CAS PubMed.
- T. Liu, C. Wang, X. Gu, H. Gong, L. Cheng, X. Shi, L. Feng, B. Sun and Z. Liu, Adv. Mater., 2014, 26, 3433–3440 CrossRef CAS PubMed.
- C. Tan and H. Zhang, Chem. Soc. Rev., 2015, 44, 2713–2731 RSC.
- Z. Liu, J. T. Robinson, S. M. Tabakman, K. Yang and H. Dai, Mater. Today, 2011, 14, 316–323 CrossRef CAS.
- J. Chen, C. Liu, D. Hu, F. Wang, H. Wu, X. Gong, X. Liu, L. Song, Z. Sheng and H. Zheng, Adv. Funct. Mater., 2016, 26, 8715–8725 CrossRef CAS.
- G. Song, C. Liang, H. Gong, M. Li, X. Zheng, L. Cheng, K. Yang, X. Jiang and Z. Liu, Adv. Mater., 2015, 27, 6110–6117 CrossRef CAS PubMed.
- W. Z. Teo, E. L. K. Chng, Z. Sofer and M. Pumera, Chemistry, 2014, 20, 9627–9632 CrossRef CAS PubMed.
- K. Keyshar, Y. Gong, G. Ye, G. Brunetto, W. Zhou, D. P. Cole, K. Hackenberg, Y. He, L. Machado, M. Kabbani, A. H. C. Hart, B. Li, D. S. Galvao, A. George, R. Vajtai, C. S. Tiwary and P. M. Ajayan, Adv. Mater., 2015, 27, 4640–4648 CrossRef CAS PubMed.
- S. Shen, Y. Chao, Z. Dong, G. Wang, X. Yi, G. Song, K. Yang, Z. Liu and L. Cheng, Adv. Funct. Mater., 2017, 27, 1700250 CrossRef.
- M. R. Vijayakumar, R. Kosuru, S. K. Singh, C. B. Prasad, G. Narayan, M. S. Muthu and S. Singh, RSC Adv., 2016, 6, 74254–74268 RSC.
- H. R. Gwak, S. Kim, D. N. Dhanasekaran and Y. S. Song, Cancer Lett., 2016, 371, 347–353 CrossRef CAS PubMed.
- Q. Jiang, M. Yang, Z. Qu, J. Zhou and Q. Zhang, BMC Complementary Altern. Med., 2017, 17, 477 CrossRef PubMed.
- S. Tortorella and T. C. Karagiannis, Curr. Drug Delivery, 2014, 11, 427–443 CrossRef CAS.
- S. Das, H. S. Lin, P. C. Ho and K. Y. Ng, Pharm. Res., 2008, 25, 2593–2600 CrossRef CAS PubMed.
- Y. Xin, T. Liu and C. Yang, Int. J. Nanomed., 2016, 11, 5807–5821 CrossRef CAS PubMed.
- Y. Chen, C. Tan, H. Zhang and L. Wang, Chem. Soc. Rev., 2015, 44, 2681–2701 RSC.
- Y. Zhu, J. Shi, W. Shen, X. Dong, J. Feng, M. Ruan and Y. Li, Angew. Chem., Int. Ed., 2005, 117, 5213–5217 CrossRef.
- D. Schmaljohann, Adv. Drug Delivery Rev., 2006, 58, 1655–1670 CrossRef CAS PubMed.
- S. K. Jones, A. Sarkar, D. P. Feldmann, P. Hoffmann and O. M. Merkel, Biomaterials, 2017, 138, 35–45 CrossRef CAS PubMed.
Footnote |
† Electronic supplementary information (ESI) available. See DOI: 10.1039/c7ra13454a |
|
This journal is © The Royal Society of Chemistry 2018 |
Click here to see how this site uses Cookies. View our privacy policy here.