DOI:
10.1039/C8RA00234G
(Paper)
RSC Adv., 2018,
8, 15056-15068
Tribocatalytic behaviour of a TiO2 atmospheric plasma spray (APS) coating in the presence of the friction modifier MoDTC: a parametric study
Received
9th January 2018
, Accepted 13th April 2018
First published on 20th April 2018
Abstract
Recent engine design and emission trends have led to the commercial use of Atmospheric Plasma Spray (APS) coatings for cylinder liner applications like the TiO2 APS coating. It was shown in our previous work that this type of coating showed better friction results compared to steel lubricated with MoDTC. To further investigate this feature, a parametric study was carried out involving the effect of MoDTC concentration, test temperature, Hertzian contact pressure and the change of counterpart materials from steel balls to ceramic balls (Al2O3 and ZrO2). Ball-on-flat tribotests were carried out on a reciprocating (ball-on-flat) tribometer lubricated with base oil containing MoDTC. Results show that for all the test conditions used including the concentration of MoDTC, test temperature and the contact pressure, lower friction and wear is observed for the TiO2 APS coating compared to reference steel. To explain the low friction behavior, tribofilm compositions were investigated and it was observed that MoS2 is always formed in the case of TiO2 APS with no oxysulphide species. For the reference steel, MoOxSy species are mainly detected in the tribofilms. XPS analyses performed on TiO2 APS flats when the counterpart material was changed from steel balls to ceramic balls suggested the formation of MoS2 (Mo in +IV oxidation state) and Mo–C (Mo in +IV or +II oxidation state) species with a negligible amount of MoO3 (Mo in +VI oxidation state). It was also shown that a significant amount of molybdenum atoms inside the tribofilm, originating from MoDTC (Mo in +V oxidation state) were reduced in the tribological contact. A mechanism for the decomposition of MoDTC on the basis of tribocatalytic behaviour hypothesized in our previous work was proposed and discussed.
Introduction
In the automotive industry, strict regulations are in place for engine emissions due to air pollutants like SOx, NOx, COx, hydrocarbons, benzene etc. which are expelled from Internal Combustion (IC) engines. Therefore, it is necessary to reduce and control the amount of these pollutants in the atmosphere. These products are formed due to the use of hazardous chemicals in the engines in the form of additives, fuels as well as oils. Thus, it is necessary to replace the previously used chemicals with environmentally friendly biolubricants and biofuels.1,2 Lubricant additives play an important role in the effective performance of the lubricant3 by reducing friction4 and wear5 in severe contacts. As they indirectly control the engine efficiency, it is necessary to ensure that the right amount of lubricant additives are used. These molecules are specifically efficient in boundary lubrication conditions. In this lubrication regime, lot of asperity–asperity contact occurs and helps additive decomposition. The most common friction modifier used is the molybdenum dithiocarbamate (MoDTC) since it significantly reduces the friction between the tribopairs6 due to the generation of low shear MoS2 flaky structure on the surface of the friction surfaces. The decomposition of MoDTC has been shown to depend on various factors like test temperature, Hertzian contact pressure,7 humidity, slide-to-roll ratio (SRR),8 concentration of MoDTC,8,10 ageing of MoDTC,10 tribopair material properties (roughness, chemistry)8,12,13 etc. It has also been shown that an optimum set of parameters is required to ensure complete decomposition of MoDTC to MoS2.8
Studies on decomposition of MoDTC have been carried out. For example, Grossiord et al.6 and De Barros Bouchet et al.12 suggested a two-step mechanism for decomposition. Results indicated that electron transfer is necessary in the initial step to form an intermediate Mo complex (Mo oxysulphide). The thiocarbamate groups of MoDTC also dissociate from the molecule in this step. In the second step, the molybdenum oxysulphide decomposes to form MoS2 and MoO3 (oxidises to form MoO3) and the two thiocarbamate groups combine to form thiuram disulphide. The tribofilms formed in these works were characterised by XPS and revealed the formation of Mo-oxysulphide, MoS2 and MoO3. Formation of MoS2 was confirmed by TEM.
Khaemba et al.7 suggested an alternative reaction pathway for the decomposition of MoDTC and concluded that the Mo +VI contribution in XPS which was attributed to the formation of MoO3 is due to FeMoO4 which was confirmed by Raman spectroscopy. The mechanism suggested involved formation of amorphous MoSx from MoDTC which in presence of high local pressures gets converted to MoS2. If optimum set of parameters (temperature, pressure and concentration of MoDTC) are not used, decomposition of MoDTC is not complete and leads to the formation of FeMoO4 along with MoSx and few amount of MoS2. Optimisation of tribotest parameters for specific tribological conditions yields high amount of MoS2, less amount of MoSx and FeMoO4 formation.7
For the reduction reaction of MoDTC to occur (from Mo +V to Mo +IV), a reducing agent seems to be needed in the form of negatively charged particles (ions, electrons, free radicals) or additives (electron donor).11,14 The change in the oxidation state of Mo is also needed in order to lower the energy barriers leading to a linkage isomer of the MoDTC that has been identified as a good candidate to be easily decomposed to MoS2. We theoretically studied a possible reaction pathway for reduction of MoDTC to the linkage isomer (LI MoDTC). After calculating the energy required for each step, we found that an initial reduction of Mo +V to Mo +IV lowers the highest energy barrier by a factor of two (from 86.83 to 39.5 kcal mol−1). As shown in Fig. 1, as soon as one of the two molybdenum atoms of MoDTC molecule is reduced, the energy barrier for individual intermediate steps to form LI-MoDTC and then MoS2 is lowered. Therefore, it can be concluded that reducing agent and energy both help in faster decomposition of MoDTC to MoS2.
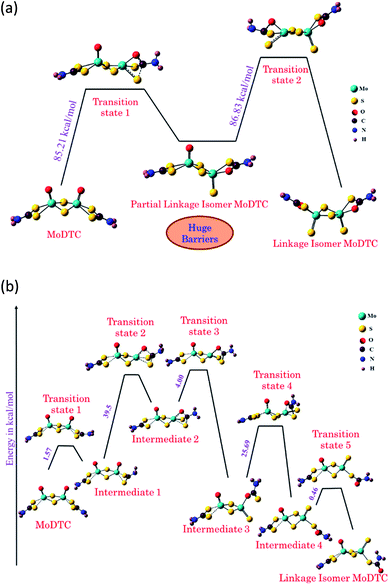 |
| Fig. 1 Reaction mechanism pathway for the conversion of MoDTC to linkage isomer of MoDTC when: (a) both Mo atoms are in +V oxidation state (b) one Mo is in +V and the other in +IV oxidation state. The structure of various isomers of MoDTC, the transition states and intermediates in the reaction pathway were optimized using the hybrid DFT functional B3LYP with 6-31+g (d,p) basis set for C, S, O, H and N atoms and def2-TZVP for Mo atom as implemented in Gaussian 09.38 | |
Majority of the experimental and theoretical studies involving decomposition of MoDTC have been carried out in case of steel/steel contacts.7,10,15–17 However, it was suggested from our previous work1 that additives could behave in different ways on a material with different chemical compositions. It was shown that better friction behavior is obtained in case of steel/TiO2 APS coating (μ ≈ 0.040) than with steel/reference steel (μ ≈ 0.055). This low friction behavior was attributed to the differences in tribochemistry and morphology of the MoS2 tribofilm formed on the coating surface. From the XPS results, it was shown that MoS2 and MoO3 is formed in case of TiO2 APS flats while mainly Mo-oxysulphide, a small amount of MoS2 and MoO3 is formed in case of reference steel flats. From the FIB-TEM results, formation of long and crystalline MoS2 flakes on the surface of TiO2 APS coatings was observed compared to the short flakes embedded in an amorphous matrix on the surface of steel.10 It was also shown that wear resistant Magneli phases are formed in case of TiO2 APS, decreasing wear even when the contact was lubricated with only base oil. This type of wear reduction had been previously observed in Ti-based materials19,21 and attributed to the formation of Magneli phases.18,19,21,22 Results obtained in our previous work are summarized in Fig. 2, highlighting the various compounds formed inside the tribofilm.
 |
| Fig. 2 Schematic highlighting the compounds obtained after friction test under boundary lubrication conditions in presence of MoDTC and TiO2 APS coating (0.7 GPa Hertzian maximum pressure, 100 °C, 0.5 wt% MoDTC in base oil). | |
From the results, it is clear that TiO2 APS does not directly react with the MoDTC additive but plays an important role in the reduction reaction to ensure complete decomposition of MoDTC. TiO2 acts as a catalyst in the reaction. However, since this effect is observed only in tribological conditions it can be termed as tribocatalysis. To evaluate this tribocatalytic behaviour, a parametric study, testing the effect of MoDTC concentration, test temperature, contact pressure, and change of counterpart material (from steel to Al2O3 and ZrO2), is carried out on steel/reference steel and steel/TiO2 APS contacts. Comparison of friction and wear behaviours as well as tribofilm compositions is done and followed by a discussion on tribocatalytic behaviour of TiO2 APS coating related to the decomposition of MoDTC in these types of contacts.
Experimental
Materials
Tribopairs. TiO2 APS coating in rutile phase was purchased from Oerlikon Balzers. Deposition was carried out by Oerlikon Balzers, Switzerland and the process used is explained elsewhere.23 Fused and crushed, micron sized powders were used to obtain desired roughness parameters for cylinder liner applications.24 Standard AISI 52100 steel discs were purchased from PCS Instruments, UK and were used as a reference flat material. Counterpart materials used were standard steel (AISI 52100) balls (Ra = 20 nm). Moreover, bulk alumina and zirconia balls were also used as counterparts. These were purchased from CIMAP, France. Radius of the balls used was 6.35 mm. Table 1 lists the properties (Ra, hardness and elastic modulus) of as received balls and Table 2 lists the properties (Ra, hardness and elastic modulus) of as received flats. Average surface roughness, hardness and elastic modulus of the APS coated flats were measured by using interferometry and Zwick micro-hardness tester respectively. Thickness of TiO2 APS coatings was 70 μm. Thus, the coatings were thick enough to consider the hardness and elastic modulus of only the coating and not the substrate.
Table 1 Properties (Ra, hardness and elastic modulus) of the ball materials
Ball material |
Ra |
Hardness (GPa) |
Elastic modulus (GPa) |
AISI 52100 steel |
<20 nm |
7 |
210 |
ZrO2 |
20 nm |
13 |
200 |
Al2O3 |
20 nm |
16 |
380 |
Table 2 Properties (Ra, hardness and elastic modulus) of the flat materials
Flat material |
Ra |
Hardness (GPa) |
Elastic modulus (GPa) |
AISI 52100 steel |
<20 nm |
7 |
210 |
TiO2 APS |
120 nm |
7 ± 1 |
160 ± 20 |
Lubricants. Lubricant used is a base oil, standard group 3 mineral type with the addition of MoDTC. It had a viscosity of 0.026 Pa s at 40 °C and 0.008 Pa s at 100 °C. MoDTC, an organometallic friction modifier additive was provided by Total Marketing Services, France. It was added in various concentrations from 0.5 wt% to 0.01 wt% in base oil. The lubricant was blended using magnetic stirrers after adding various concentrations of MoDTC in base oil at 60 °C for 2 hours to ensure that the additive is completely dissolved in the base oil.
Methods
Ball-on-flat tribotests using linear tribometer. Reciprocating ball-on-flat tests were carried out with steel (AISI 52100) balls and ceramic balls against reference steel and TiO2 APS flats. All the tribopairs were cleaned in an ultrasonic bath for 10 minutes in n-heptane. To investigate the presence of tribocatalytic activity in TiO2 APS, different parameters like test temperature, maximum contact pressure, concentration of MoDTC and change of counterpart material were used and compared with reference steel flats. Tribotests were carried out considering mechanical properties of all the tribopairs used. General tribotest conditions used are listed in Table 3. From Hertzian theory of contact mechanics, normal loads were calculated for different tribopairs taking into account the elastic moduli of all tribopair materials. Also, to ensure that the tests are carried out in boundary or mixed lubrication conditions, lambda ratio was calculated. Initially, the lubricant film thickness (hmin) was calculated using Hamrock–Dawson equation.25
Table 3 Ball-on-flat reciprocating tribotest conditions
Temperature |
20 °C/60 °C/100 °C |
Frequency |
5 Hz |
Stroke length |
5 mm |
Normal load (max pressure) |
2 N (0.45 GPa), 7 N (0.7 GPa), 21 N (1 GPa) – steel/TiO2 APS, 2 N (0.45 GPa), 6 N (0.7 GPa), 17 N (1 GPa) – steel/steel |
Sliding distance |
180 m |
Test duration |
1 h, 18 000 cycles |
Ball material |
AISI 52100 steel, Al2O3, ZrO2 |
Flat material |
AISI 52100 steel, TiO2 APS |
Lubricant |
Base oil + 0.5/0.3/0.1/0.05/0.03/0.01 wt% MoDTC |
Following is the list of parameters studied:
(a) Effect of concentration of MoDTC:
To study the effect of concentration of MoDTC on the tribological behavior of steel/reference steel and steel/TiO2 APS contacts, tests were performed with varying concentrations of MoDTC starting from 0.01 wt%, 0.03 wt%, 0.05 wt%, 0.1 wt%, 0.3 wt% and 0.5 wt% in base oil at a constant temperature of 100 °C, a sliding speed of 0.05 m s−1 and a maximum Hertzian contact pressure of 0.7 GPa.
(b) Effect of test temperature:
To study the effect of temperature on the tribological behavior of steel/steel and steel/TiO2 APS contacts, tribotests were performed at three different temperatures of 20 °C, 60 °C and 100 °C at a maximum Hertzian pressure of 0.7 GPa and a sliding speed of 0.05 m s−1 lubricated with base oil + 0.3 wt% MoDTC.
(c) Effect of contact pressure:
To study the effect of pressure on the tribological behavior of steel/steel and steel/TiO2 APS contacts, tribotests were performed at three different pressures of 0.4 GPa, 0.7 GPa and 1 GPa at a constant temperature of 100 °C and a sliding speed of 0.05 m s−1 lubricated with base oil + 0.3 wt% MoDTC.
(d) Effect of change of counterpart material against TiO2 APS:
To study the effect of change of counterpart material on the tribological behaviour of TiO2 APS flats, counterpart material was changed from steel balls to alumina and zirconia balls. Also, the effect of absence of Fe on the formation of low friction MoS2 was studied in ceramic/ceramic contacts. Tribotests were carried out at a maximum Hertzian pressure of 0.7 GPa, a constant temperature of 100 °C and a sliding speed of 0.05 m s−1 lubricated with base oil + 0.3 wt% MoDTC. Normal loads were adjusted taking into account the mechanical properties of balls and flats to ensure that the tests were carried out in boundary lubrication conditions.
Wear scar analysis. Wear scars on the balls and flats were analysed using an optical microscope. Wear scar diameter on the ball and the wear scar width on flats were also measured. These measured values were then compared with the respective Hertzian diameters. To compare the wear for different tribopairs, percentage of wear above the Hertzian diameter was calculated.
Surface characterisation of the tribofilms. The tribopairs were cleaned once with n-heptane before surface characterization. Tribofilms on the flats and balls were characterized using X-Ray Photoelectron Spectroscopy (XPS). The equipment used was an ULVAC-PHI Versaprobe II spectrometer equipped with a monochromatized Al Kα X-ray source (1486.6 eV). The calibration of binding energy was done using Au4f7/2 and Au4f5/2 as the binding energies were known at 84 and 87.7 eV respectively, following the calibration procedure from the manufacturer. Charge compensation system was used to compensate for the charging effect. The adventitious C1s peak (C–C bond) was fixed at 284.8 eV before fitting for additional charge correction. Initially, a survey spectrum was recorded with a pass energy of 187.85 eV in the range 0–1100 eV to identify all the elements present in the tribofilm. Then, high resolution spectra were obtained with a pass energy of 23.5 eV for all the elements. All the spectra were fitted using a Shirley background and analyzed using PHI Multipak software.
Results and discussion
Effect of concentration of MoDTC
Friction results. Comparison of friction results for steel/reference steel and steel/TiO2 APS contacts is shown in Fig. 3 when the concentration of MoDTC in base oil is lowered from 0.5 wt% to 0.01 wt% MoDTC in base oil. The major difference between steel/TiO2 APS and steel/reference steel is the value of steady state friction coefficient obtained. In case of steel/TiO2 APS contact, steady state friction coefficient is similar whatever the concentration whereas in case of steel/reference steel contact, steady state friction coefficient increases as the concentration of MoDTC is reduced in base oil from 0.5 wt% MoDTC (μ ≈ 0.045) to 0.01 wt% MoDTC (μ ≈ 0.12). Friction curves show that even 0.01 wt% of MoDTC in base oil is enough to achieve low friction in case of steel/TiO2 APS with some induction time; whereas in case of steel/reference steel, friction behavior at 0.01 wt% is similar to that of the base oil. Induction time is observed in steel/reference steel when it is lubricated with base oil + 0.03, 0.05, 0.1 wt% MoDTC as shown in the Fig. 3(a). However, in case of steel/TiO2 APS contact, induction time appears only below the concentration of 0.1% MoDTC.
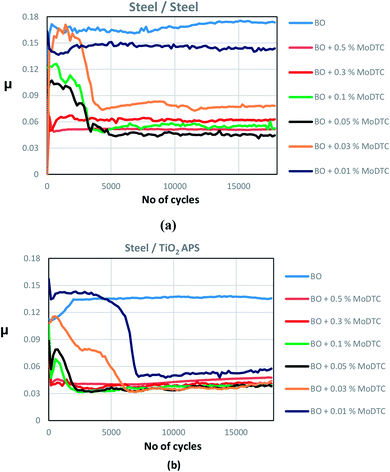 |
| Fig. 3 Friction curves obtained after tests at 100 °C, at 0.05 m s−1, with maximum contact pressure of 700 MPa and at various concentrations of MoDTC in base oil for (a) steel/reference steel (b) steel/TiO2 APS. | |
Wear results. Wear behavior for steel/reference steel and steel/TiO2 APS is compared in the histogram shown in Fig. 4. From the Hertzian theory of contact mechanics, calculated values of Hertzian diameters for steel balls in case of steel/reference steel and steel/TiO2 APS contacts are 125 μm and 141 μm respectively. For base oil + 0.01 wt% MoDTC, it is observed that percentage of steel ball wear above the respective Hertzian diameters is lower in case of TiO2 APS compared to reference steel. All the other concentrations show similar wear behavior probably due to formation of friction reducing tribofilm by decomposition of MoDTC on the contact surfaces. Low friction behavior at 0.01 wt% MoDTC in case of TiO2 APS suggests that a tribofilm is formed whereas it is not formed in case of reference steel flat as it shows higher wear and friction coefficient similar to that of base oil. The best combination of low wear and low friction coefficient without any induction time is observed in case of steel/TiO2 APS contact when it is lubricated with base oil + 0.3% MoDTC. Also, the wear and friction behavior for steel/reference steel is better when it is lubricated with base oil + 0.3% MoDTC compared to other concentrations. Therefore, base oil + 0.3% MoDTC is used for further parametric studies. However, the optimum concentration of MoDTC in case of both the contacts will be different for different loading conditions. In these loading conditions, it is found to be 0.3 wt% MoDTC for steel/steel as well as steel/TiO2 contacts.
 |
| Fig. 4 Comparison of wear trends on the steel ball tested against reference steel and TiO2 APS flats lubricated with various concentrations of MoDTC in base oil (tribotest at 100 °C, at 0.05 m s−1, with maximum contact pressure of 700 MPa). | |
Surface analysis of the tribofilms using XPS. To investigate the reduction in friction coefficient even at low concentration of MoDTC in case of TiO2 APS and the differences in friction coefficients between reference steel and TiO2 APS, XPS analyses were carried out. To analyse the reduction in friction coefficient, Mo3d spectra obtained for three different concentrations (0.3, 0.05 and 0.01 wt% MoDTC) are compared for reference steel and TiO2 APS flats in Fig. 5.
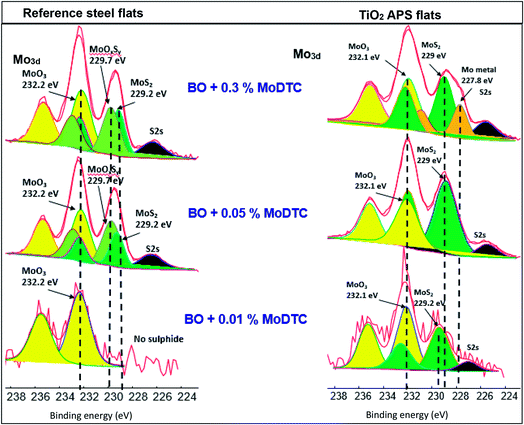 |
| Fig. 5 Comparison of high resolution Mo3d XPS spectra for reference steel (left) and TiO2 APS flats (right) lubricated with (from the top) base oil + 0.3wt%, base oil + 0.05 wt% and base oil + 0.01 wt% MoDTC (tribotest at 100 °C, at 0.05 m s−1, with maximum contact pressure of 700 MPa). | |
The molybdenum peak is divided into various contributions arising from Mo metal – (orange peaks), sulphide – (dark green peaks, Mo +IV), oxide – (yellow peaks, Mo +VI) and oxysulphide – (light green peaks, oxidation state of Mo between +IV and +V). Since the S2s peak (black) of sulphur overlaps the Mo3d peak, S2p peak is fitted initially and then, taking into account the binding energy and the height of the peak, S2s peak is fitted. All the respective contributions are fitted after fixing the binding energy values of Mo3d obtained from literature (Mo metal – 227.8 ± 0.2 eV, sulphide – 229 ± 0.2 eV, oxide – 232 ± 0.2 eV, oxysulphide – 229.7 ± 0.2 eV).9,10,26
XPS results comparison at various concentrations of MoDTC shows that MoS2 is obtained in case of TiO2 APS, although, in case of reference steel flats, MoOxSy contribution is observed along with MoS2. MoO3 is detected in all the cases. In case of 0.3 wt% MoDTC in base oil with TiO2 APS flat, MoS2 and MoO3 is observed in the Mo3d spectra along with an even more reduced Mo compound at the binding energy of 227.8 eV assigned to molybdenum metal. At 0.01 wt% MoDTC in base oil in case of reference steel, Mo3d spectra shows only MoO3 formation inside the tribofilm. This is in agreement with the friction behavior (μ = 0.12) which is similar to that of base oil. However, in case of the tribofilm on the TiO2 APS flat, MoS2 is formed even at the lowest concentration of 0.01 wt%. This clearly suggests that in presence of TiO2 APS coating, complete decomposition of MoDTC occurs even when the concentration of MoDTC is reduced. Thus, low friction could be achieved.
Effect of temperature
Friction results. Fig. 6 shows the comparison of friction curves obtained for steel/reference steel and steel/TiO2 APS. Three different temperatures of 20 °C, 60 °C and 100 °C were used at a constant maximum Hertzian pressure of 0.7 GPa lubricated with base oil + 0.3 wt% MoDTC. For the tests conducted at room temperature (20 °C), it is observed that the steady state friction coefficient obtained for steel/TiO2 APS (μ = 0.06) is lower than for steel/reference steel (μ = 0.08).
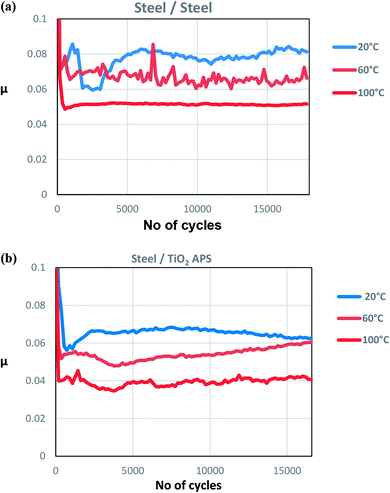 |
| Fig. 6 Comparison of friction behaviour for (a) steel/steel (b) steel/TiO2 APS at different temperatures (tribotests at 0.05 m s−1, with maximum contact pressure of 700 MPa, with 0.3 wt% of MoDTC in base oil). | |
Wear results. Fig. 7 shows the trends for the comparison of percentage wear above the Hertzian diameter on the steel ball for steel/reference steel and steel/TiO2 APS contacts at different temperatures of 20 °C, 60 °C and 100 °C when lubricated with base oil + 0.3% MoDTC. It can be observed that % wear above Hertzian diameter is higher in case of steel balls against reference steel flats at 20 °C and 60 °C as compared to steel balls against TiO2 APS flats.
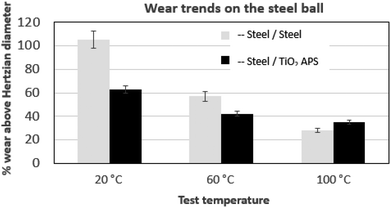 |
| Fig. 7 Comparison of wear trends on the steel ball tested against reference steel and TiO2 APS flats lubricated with base oil + 0.3% MoDTC at different temperatures (20 °C, 60 °C and 100 °C). | |
When the temperature is reduced to lower values, the viscosity of the oil is increased and the lubrication regime is changed. However, since wear is observed in both the contacts and it is higher at low temperatures, contact between asperities is still present due to significant Hertzian contact pressure. The lower wear observed at higher temperatures could be due to the complete activation of MoDTC whereas it fails to activate completely at low temperatures leading to higher wear. Similar trends of friction and wear behaviour in steel/reference steel were observed by Khaemba et al.7 when they studied the effect of temperature on the tribological behaviour.
On the other hand, in case of steel ball sliding against TiO2 APS compared to the steel ball sliding against reference steel, the wear is lower at room temperature.
Surface analysis of the tribofilms using XPS. To analyse the differences in friction behavior at different temperatures, Mo3d high resolution spectra were obtained for reference steel (left side) and TiO2 APS flats (right side) and are shown in Fig. 8. Similar fitting parameters and procedure were used for the Mo3d peak as mentioned in the concentration section.
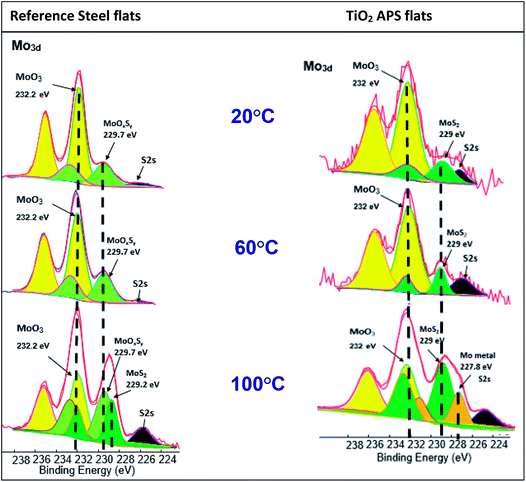 |
| Fig. 8 Comparison of high resolution XPS Mo3d spectra for reference steel (left) and TiO2 APS flats (right) at different temperatures of 20 °C, 60 °C and 100 °C lubricated with base oil + 0.3% MoDTC at a maximum Hertzian contact pressure of 700 MPa and at a speed of 0.05 m s−1. | |
Comparison of tribochemistry at various test temperatures for steel and TiO2 APS flats shows that pure MoS2 (green peaks) is obtained in case of steel/TiO2 APS at all temperatures. This suggests complete decomposition of MoDTC to MoS2; whereas in case of reference steel flats, MoOxSy contribution along with MoO3 is observed at low temperatures of 20 °C and 60 °C. However, MoOxSy, MoO3 along with MoS2 is formed at higher temperature of 100 °C which is in agreement with the lower friction coefficient of 0.055. However in case of TiO2 APS at 100 °C, Mo metal contribution is observed at a binding energy of 227.8 eV which suggests even more reduction of Mo species in the tribofilm from Mo +IV to Mo(0).
The lower friction obtained at higher temperatures is due to the complete activation of MoDTC additive in boundary lubrication regime (contact between surface asperities) whereas it fails to activate completely at low temperatures leading to higher friction. High temperature provides energy for the chemical reaction to proceed faster to completion. In this case, decomposition of MoDTC requires high temperatures to form MoS2 in case of steel/reference steel contacts as Mo-oxysulphides are formed and MoS2 is not formed at low temperatures. Therefore, it can be concluded that higher temperatures are required to decompose MoDTC to form MoS2.27 Similar effect was observed by Khaemba et al.7 in case of steel/reference steel contact, where they studied the effect of temperature on the decomposition of MoDTC and suggested that high temperatures are required to form pure MoS2 as lower temperatures tend to oxidise the tribofilm and lead to higher friction. They suggested that products like FeMoO4 are formed where Mo is in similar oxidation state as in case of MoO3 observed in this study. MoSx is also formed as an intermediate where the oxidation state of Mo remains higher (+V or +VI) which is similar to the case of MoOxSy in this study.
In case of TiO2 APS, complete decomposition of MoDTC occurs even at lower temperatures but at a slower rate. The amount of MoS2 formed may not cover the whole surface and it may be different at various temperatures. Thus, the friction coefficient obtained is higher at 20 °C than at 100 °C in case of TiO2 APS flats. It can be concluded that low temperature slows the rate of decomposition of MoDTC but does not prevent it to form MoS2 which helps in reducing the friction coefficient compared to steel/reference steel contact.
Effect of contact pressure
Friction results. Comparison of friction results obtained for steel/reference steel and steel/TiO2 APS contacts at different pressures of 0.4 GPa, 0.7 GPa and 1 GPa and at a constant temperature of 100 °C lubricated with base oil + 0.3% MoDTC is shown in Fig. 9. For tests conducted at low pressure (0.4 GPa), it is observed that the steady state friction coefficient obtained for steel/TiO2 APS (μ = 0.05) is lower than for steel/reference steel (μ = 0.085). Also, for tests conducted at the pressures of 0.7 GPa and 1 GPa, it is observed that the steady state friction coefficient obtained for steel/TiO2 APS (μ ≈ 0.042 at 0.7 GPa and 1 GPa) is lower than for steel/reference steel (μ ≈ 0.06 at 0.7 GPa and μ ≈ 0.052 at 1 GPa). For reference steel flats, friction coefficient is the lowest at 1 GPa and the highest at 0.4 GPa. This suggests that high pressure is required to obtain the lowest friction coefficient in case of reference steel; whereas in case of TiO2 APS, even at low pressures, lower friction coefficient of 0.05 is obtained. Therefore, it can be concluded that friction behaviour of steel/TiO2 APS contact is insensitive to pressure to a certain extent whereas steel/reference steel contact is sensitive to pressure.
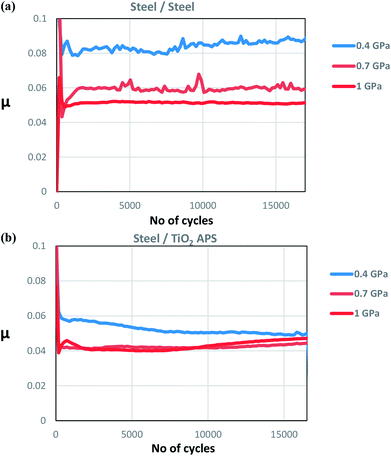 |
| Fig. 9 Comparison of friction behavior at different pressures for (a) steel/steel (b) steel/TiO2 APS. | |
Wear results. Fig. 10 shows the trends for the comparison of percentage wear above the Hertzian diameters on the steel ball for steel/reference steel and steel/TiO2 APS contacts at different pressures of 0.4 GPa, 0.7 GPa and 1 GPa when lubricated with base oil + 0.3% MoDTC. In case of steel/reference steel contact, it can be observed that the wear is clearly higher at 0.4 GPa than at 0.7 GPa and 1 GPa. This higher wear is related to higher friction coefficient at lower pressures and is probably observed because MoDTC is not activated which is evident from the friction behaviour. It can be observed that the % wear above Hertzian diameter is considerably lower in case of steel/TiO2 APS contact at 0.4 GPa and 1 GPa than steel/reference steel contact. At 0.7 GPa, the wear observed is almost similar in both the contacts. Also, comparing the % wear above Hertzian diameter for different pressures in case of steel/TiO2 APS contact, they are found to be in a similar range (34–48%) which suggests that the effect of the additive is similar at all pressures.
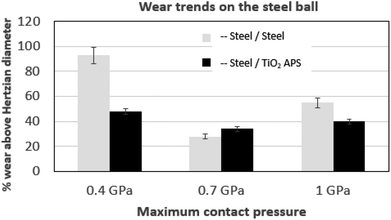 |
| Fig. 10 Comparison of wear trends on the steel ball tested against reference steel and TiO2 APS flats lubricated with base oil + 0.3% MoDTC at different contact pressures (0.4 GPa, 0.7 GPa and 1 GPa). | |
Surface analysis of the tribofilms using XPS. To investigate the differences in friction behavior at different pressures, Mo3d high resolution spectra were obtained at different pressures for reference steel and TiO2 APS flats. Comparison of the XPS spectra is shown in Fig. 11. Similar fitting procedure and parameters were used for fitting Mo3d peaks as mentioned in the concentration section.
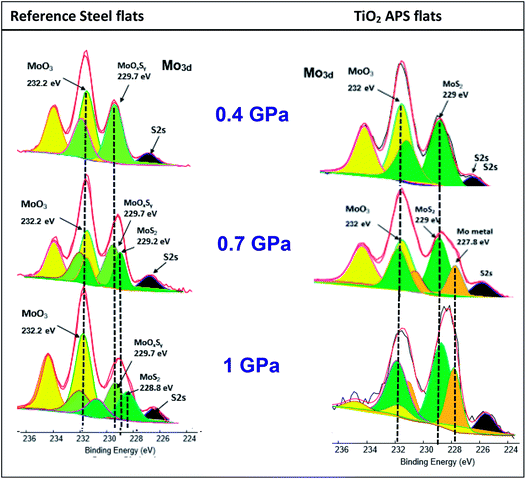 |
| Fig. 11 Comparison of high resolution XPS Mo3d spectra for reference steel (left) and TiO2 APS flats (right) at different pressures of 0.4 GPa, 0.7 GPa and 1 GPa lubricated with base oil + 0.3% MoDTC at a constant temperature of 100 °C. | |
Comparison at various test pressures for steel and TiO2 APS flats shows pure MoS2 (green peaks on the right side) is formed on the surface of TiO2 APS at all pressures which suggests complete decomposition of MoDTC to MoS2. In case of reference steel flats, only MoOxSy contribution is observed at 0.4 GPa and as the pressure is increased to 0.7 GPa and 1 GPa, MoS2 is observed along with MoOxSy. The presence of MoS2 at higher pressures explains the lower friction coefficient obtained (μ = 0.06 at 0.7 GPa and μ = 0.052 at 1 GPa) than at lower pressures. From the results, it can be suggested that higher pressures are required for complete decomposition of MoDTC in case of reference steel like mentioned previously.7 At 0.4 GPa, higher friction coefficient is observed because the amount of MoOxSy is higher in the tribofilm formed on the reference steel flat.
On the contrary, in case of steel/TiO2 APS, MoS2 is formed at all pressures which corresponds to the lower friction coefficients. The amount of MoS2 formed may be different at all pressures. However, this does not affect the friction coefficient considerably as the friction coefficient is more dependent on the amount of MoS2 covering the surface than the amount of MoO3 present. This suggests that decomposition of MoDTC additive proceeds even at the lowest pressure of 0.4 GPa. The friction behaviour obtained is in agreement with the XPS results where high amount of MoS2 is observed at 0.7 GPa and 1 GPa as compared to 0.4 GPa. At 0.7 GPa and 1 GPa, MoS2 and MoO3 is observed along with some amount of molybdenum metal species. This Mo metal species is due to further reduction of Mo atoms formed in the tribofilm. This behaviour is not observed in case of steel/reference steel.
Effect of change of counterpart material against TiO2 APS
Counterpart material sliding against TiO2 APS coating was changed from reference steel balls to Al2O3 and ZrO2 balls to investigate the effect of absence of iron and the role of tribocatalytic behaviour of TiO2 APS in the contact.
Friction results for TiO2 APS flats. Comparison of friction results obtained for steel/TiO2 APS, ZrO2/TiO2 APS and Al2O3/TiO2 APS contacts are shown in Fig. 12. The tests were carried out at a maximum Hertzian contact pressure of 0.7 GPa, a constant temperature of 100 °C lubricated with base oil + 0.3% wt MoDTC. Normal loads were adjusted taking in to account the mechanical properties of all the tribopairs to maintain similar contact pressure for comparison. It can be observed that all the three contacts - steel/TiO2 APS, ZrO2/TiO2 APS and Al2O3/TiO2 APS show similar steady state friction coefficient (μ = 0.04) and similar trends.
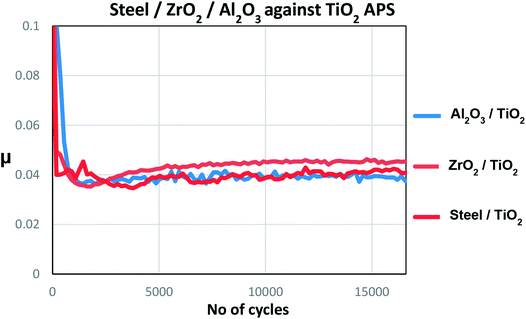 |
| Fig. 12 Comparison of friction behaviour for steel/TiO2 APS, ZrO2/TiO2 APS and Al2O3/TiO2 APS at a maximum Hertzian contact pressure of 0.7 GPa lubricated with base oil + 0.3% MoDTC and a constant temperature of 100 °C. | |
Wear scar analysis on TiO2 APS flats. Optical images of wear scars on both the tribopairs in case of steel/TiO2 APS, ZrO2/TiO2 APS and Al2O3/TiO2 APS contacts are shown in the Fig. 13. Similar method is used to calculate the Hertzian diameters like for reference steel as the mechanical properties of the tribopairs are different. Hertzian diameters are mentioned on the top of optical images for each contact.
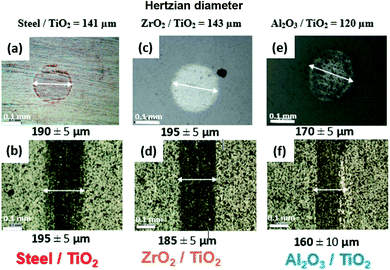 |
| Fig. 13 Optical images of the wear scars on (a) steel ball vs. reference steel flat, (b) steel flat, (c) steel ball vs. TiO2 flat, (d) TiO2 flat, (e) steel ball vs. steel APS, (f) steel APS flat when lubricated with base oil + MoDTC. | |
It can be observed that the wear observed in case of all the three contacts is similar to each other and is in the range of 30–40% above the Hertzian diameter on both tribopair materials. Low wear is observed on the TiO2 APS flats due to the wear resistant properties of TiO2.20
Fig. 14 shows the wear trends on the different balls and TiO2 APS flats tested against them. It is clear from the percentage of wear above Hertzian diameter on both the ball and the flats that the wear occurring in all the contacts is limited.
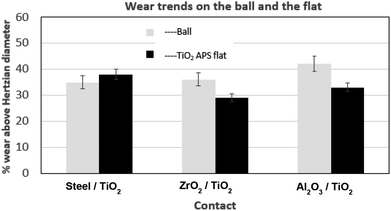 |
| Fig. 14 Comparison of wear trends on the various balls tested against TiO2 APS flats lubricated with base oil + 0.3% MoDTC at a pressure of 0.7 GPa and temperature of 100 °C. | |
Surface analysis of the tribofilms using XPS. To investigate the differences in the tribological behavior of steel, ZrO2 and Al2O3 balls sliding against TiO2 APS flats, Mo3d high resolution spectra were obtained on the wear scars of reference steel and TiO2 APS flats. Comparison of the Mo3d and C1s high resolution XPS spectra for each contact is shown in Fig. 15.
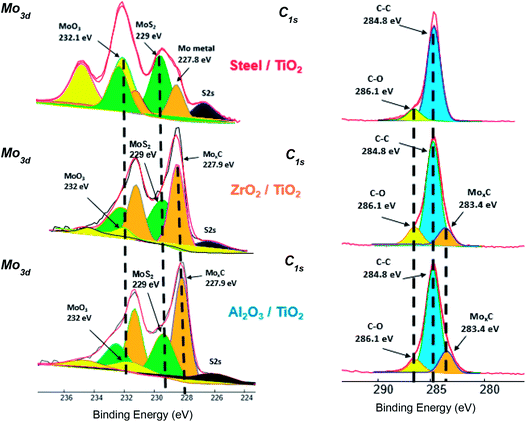 |
| Fig. 15 Comparison of high resolution XPS Mo3d and C1s spectra for TiO2 APS flats (right) sliding against steel, ZrO2 and Al2O3 balls respectively (from top to bottom) at maximum contact pressure of 0.7 GPa lubricated with base oil + 0.3% MoDTC at a constant temperature of 100 °C. | |
C1s spectra were fitted with two or three different contributions. Binding energy values used for various carbon species were C–C (284.8 ± 0.2 eV), C–O (286.1 ± 0.2 eV) and carbide (283.4 ± 0.2 eV). It is clear from the C1s spectra obtained in case of ZrO2/TiO2 APS and Al2O3/TiO2 APS contacts that carbide species is present which was also observed in the Mo3d peaks. Similar fitting procedure and parameters were used for fitting the Mo3d peak as mentioned in the previous sections. The only difference in the fitting procedure used in this case for Mo3d is the addition of a new contribution in the Mo spectra at the binding energy of 227.8 ± 0.2 eV which was assigned to the carbide species (MoxC).11 Since MoxC and Mo metal contributions have similar binding energies, it is possible that both of them are present.
Therefore, from the above results it can be confirmed that MoxC species is formed in the contact involving ZrO2 and Al2O3 balls. However, in case of steel/TiO2 APS, C1s peak does not show any carbide contribution but the Mo3d spectra shows the presence of a contribution with binding energy similar to that of carbide. This contribution is assigned to molybdenum metal as the binding energies of Mo-metal and Mo-carbide are close to each other.
Comparison of the tribochemistries for different balls sliding against TiO2 APS flats shows that MoS2 along with MoO3 is obtained in case of steel/TiO2 APS which is in agreement with the friction coefficient (μ = 0.04). Similar friction coefficient is obtained due to the formation of MoS2 in all the cases with no MoOxSy. However, MoS2 and MoO3 are observed along with further reduced contribution of molybdenum assigned to molybdenum metal (227.8 ± 0.2 eV). Also, in case of ZrO2/TiO2 APS and Al2O3/TiO2 APS contacts, MoS2 is observed but along with a new contribution at much lower energies assigned to MoxC (227.9 ± 0.2 eV) confirmed by C1s peak fitting. This could be due to further reduction of molybdenum contribution to Mo +IV (MoC) or Mo +II (Mo2C). The presence of molybdenum metal is still possible.
From the XPS results, Mo-carbide (Mo +II or +IV) and Mo-sulphide (Mo +IV) is formed in case of Al2O3/TiO2 APS and ZrO2/TiO2 APS contacts with MoO3 (Mo +VI) whereas in case of steel/TiO2 APS contact, MoO3 (Mo +VI) is formed along with MoS2 (Mo +IV) and Mo metal (Mo in oxidation state of 0). This clearly suggests that there is more reduction of Mo atoms in MoDTC molecule in case of ceramic/ceramic contact than with steel/ceramic contact.
Mechanism
From the results and discussions for the effect of various parameters obtained in case of reference steel and TiO2 APS flats on their tribological behaviour, it is clear that complete decomposition of MoDTC occurs in case of TiO2 APS leading to the formation of MoS2 inside the tribofilm at tested temperatures, pressures and concentrations. As discussed before, it was suggested that since the TiO2 APS coating does not directly react with MoDTC, it acts as a tribocatalytic coating. Therefore, tribocatalytic behaviour of TiO2 coating was considered as the mechanism responsible for the complete decomposition of MoDTC to MoS2. Also, it was suggested that the tribocatalytic behaviour involves a step of triboemission where negatively charged particles are generated from the surface of TiO2 which are responsible for the initial reduction of Mo atoms in the MoDTC molecule.
To investigate more about this tribocatalytic behaviour of TiO2 APS coatings, results obtained from various parameters are summarized and discussed:
(a) Effect of temperature. XPS results obtained for steel/TiO2 APS contact suggested formation of MoS2 for all tested temperatures. However, more reduced contribution (Mo metal) is observed at 100 °C. At lower temperatures (20 °C and 60 °C), the contact is less severe and the Mo metal species were not observed. This Mo metal contribution is not observed in case of reference steel.
(b) Effect of pressure. Similar friction coefficients were observed at all tested contact pressures for steel/TiO2 APS contacts which is in agreement with the XPS results where pure MoS2 was observed in all cases. Also, reduced species of molybdenum (Mo metal) were observed at higher pressures of 0.7 GPa and 1 GPa along with MoS2 and MoO3. This further reduction in Mo species is again observed only for steel/TiO2 APS contacts.
(c) Effect of change of counterpart material. Similar friction results were obtained when TiO2 APS flats were tested against ceramic balls and steel balls which was in agreement with the XPS results where pure MoS2 formation was observed. However, replacing the steel ball counterpart with ceramic balls like ZrO2 or Al2O3 led to even more reduction of Mo atoms in the tribofilm as MoxC are found. Few amount of MoO3 is detected.These results clearly show evidence of reduced Mo species in tribological contacts involving TiO2 APS flats and lubricated with MoDTC. Actually, molybdenum, initially in +V oxidation state in the MoDTC molecule, is found to be reduced to species such as MoS2 (+IV), Mo metal (0) and also MoC (+II) or Mo2C (+IV) in case of ceramic/TiO2 APS contacts.
The formation of this kind of reduced Mo species could be due to negatively charged particles generated under boundary lubricated tribological conditions. Formation of even more reduced species in ceramic/TiO2 APS contact could be due to more generation of negatively charged particles from the ceramic/ceramic contact than steel/ceramic contact.
From the above discussion concerning the indirect evidences of negatively charged particles, it can be considered that negatively charged particles could be generated under boundary lubricated tribological conditions in case of steel/TiO2 and ceramic/TiO2 APS contact which help in complete decomposition of MoDTC to MoS2 as well as more reduced species inside the tribofilm. This is in agreement with theoretical work on MoDTC decomposition pathway presented in the introduction,12 as it was shown that the reduction of Mo atom in MoDTC molecule is required to reduce the energy barriers experimentally to form MoS2. However, it is necessary to find out the source of these negatively charged particles. They could come from different tribological processes occurring in a contact which are discussed in the following:
(1) Triboemission. First source could be the generation of negatively charged particles (ions, electrons, free radicals) from ceramic oxides in lubricated tribological conditions as observed by Molina et al.28–31 and Nakayama et al.32,33 where they showed that high amount of emission is observed in case of insulating ceramic oxides like alumina even in boundary lubrication conditions. This generation of negatively charged particles is a necessary step of the tribocatalytic behaviour. Kajdas et al.28,33 also suggested that this triboemission process could occur in lubricated contact involving aluminum oxide and help in additive degradation process (ZDDP in their study). In this work, as a ceramic, TiO2 could also generate the negatively charged particles under lubricated conditions i.e. triboemission which then helps in the decomposition of MoDTC to MoS2. The importance of triboemission process for tribochemical reaction was also considered and discussed by Kajdas.34
(2) Magneli phases. As shown in our previous work,1 wear resistant Magneli phases are generated from the surface of TiO2 APS coating in tribological conditions. The second source of negatively charged particles could be the generation of electrons from the vacancies generated due to the formation of wear resistant Magneli phases. Magneli phases are the oxygen deficient species with the general formula TiOx, where x is between 1.75 and 1.83. Therefore, whenever the oxygen is lost from the TiO2 rutile surface, an oxygen vacancy created at the site has two electrons to maintain the electrical charge neutrality as explained by Gardos et al.35 Electrons obtained at this site could be used for initial reduction of MoDTC to accelerate the reaction of forming MoS2. Also, these Magneli phases due to the presence of a mixture of corundum and rutile structure are known for charge storage ability.36 Therefore, whenever electrons are generated on the surface of the TiO2 APS coating, these could be stored for a long period of time and could ensure complete decomposition of MoDTC as well as avoid the formation of oxygen containing compounds like MoOxSy and sulphate formed in case of reference steel. As mentioned before, Magneli phase generation was confirmed by Raman spectroscopy in our previous work. Even if there is a lack of a direct evidence, this strong indirect evidences could lead us to consider that electrons are generated in the contact through the Magneli phase sites which help in the complete decomposition of MoDTC to MoS2. Considering the fact that reduced Mo metal and carbide species are observed in case of steel/TiO2 APS contact as well as ceramic/TiO2 APS contacts, it could be due to the combination of both the sources of negatively charged particles, triboemission as well as Magneli phase formation that generate excess negatively charged particles in the contact and in turn help to form Mo metal as well as carbide species. However, it is true that there is no direct evidence of triboemission or negatively charged particles in the contact.
Proposed mechanism
The proposed reaction mechanism for the decomposition of MoDTC in case of steel/TiO2 APS contacts is sum-up in Fig. 16. This mechanism shows that when a steel/TiO2 APS contact is subjected to reciprocating sliding under boundary lubrication conditions in presence of base oil + MoDTC, it leads to complete decomposition of MoDTC due to the negatively charged particles generated from the TiO2 surface. Also, wear resistant Magneli phases are generated on the surface of TiO2.1 This type of tribocatalytic behaviour is unique to TiO2 APS coating and not observed in case of reference steel. Also, the replacement of S by O species occurs in case of reference steel flats as XPS always shows the formation of oxysulphides, sulphates (Fe-sulphates) which could lead to higher wear and corrosion.37
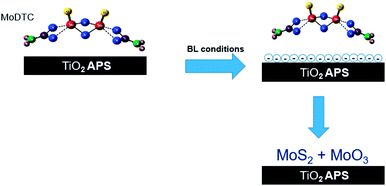 |
| Fig. 16 Schematic of the decomposition mechanism of MoDTC in steel/TiO2 APS contacts. | |
Overall results obtained from the parametric studies suggest that TiO2 APS coating exhibits tribocatalytic behaviour and could be an alternative to the Fe-based materials used for cylinder liners.
Conclusions
This study investigated the tribocatalytic behavior of TiO2 APS coating by a parametric evaluation. The effect of reduction in concentration of MoDTC, test temperature, contact pressure and change of counterpart materials was studied on the tribological behavior of steel/TiO2 APS. Comparisons were made with reference steel on the basis of tribological results and the tribochemistry involved. Following are the conclusions obtained:
(1) Steel/TiO2 APS contact is more effective in reducing friction and wear at the concentration of MoDTC as low as 0.01 wt% in base oil compared to the steel/reference steel contacts. XPS studies concluded that MoS2 is clearly formed at this concentration of MoDTC in case of tribofilm on the TiO2 APS flats whereas it is not formed on the reference steel flat leading to higher friction.
(2) Studies on the effect of temperature suggested that TiO2 APS flat shows formation of pure MoS2 at all tested temperatures whereas it is not formed in case of reference steel flats at 20 and 60 °C. Therefore, higher friction is observed in case of steel/reference steel contacts compared to steel/TiO2 APS contact at all tested temperatures.
(3) Studies on the effect of pressure showed that steel/TiO2 APS shows formation of MoS2 at all tested contact pressures and thereby shows lower friction coefficient compared to steel/reference steel in which oxysulphide formation is observed at all pressures. Overall, the steel/TiO2 APS contact is insensitive to pressure to a certain extent whereas steel/reference steel contact is sensitive to pressure.
(4) Effect of change of counterpart material in case of TiO2 APS from steel balls to ceramic balls showed no change in tribological behaviour as well as an increase in amount of reduction of MoDTC to form pure MoS2 and further reduced compounds of Mo like MoxC (carbides) along with traces of molybdenum oxides.
(5) Tribocatalytic behaviour of TiO2 APS could be confirmed as the TiO2 APS helps in complete decomposition of MoDTC to MoS2 in presence of tribological conditions. This help is in the form of generation of negatively charged particles from the surface of TiO2 APS either coming from: (i) the oxygen vacancy formation on the TiO2 surface due to Magneli phase formation and/or (ii) triboemission from TiO2 surface in boundary lubrication conditions.
Conflicts of interest
There are no conflicts of interest to declare.
Acknowledgements
This study was funded by the FP 7 MC-ITN program titled Engineering Tribochemistry of Internal Combustion Engines (ENTICE) [290077]. It was carried out in LTDS, Ecole Centrale de Lyon and Centre de Recherche de Solaize, France. The authors would like to thank Oerlikon (Mr Frederic Meunier and Peter Ernst) for providing the coatings for this project.
References
- P. Deshpande, C. Minfray, F. Dassenoy, B. Thiebaut, Th. Le Mogne, B. Vacher and F. Jarnias, Tribol. Int., 2018, 118, 273–286 CrossRef CAS.
- A. Igartua, X. Fernandez, O. Areitiaurtena, R. Luther, C. Seyfert, J. Rausch, I. Killarramendi, M. Berg, H. Schultheis, B. Duffau, S. Plouseau and M. Woydt, Tribol. Int., 2009 DOI:10.1016/j.triboint.2008.10.015.
- S. Baskar, G. Sriram and S. Arumugam, Tribol. Int., 2015, 37, 449–454 Search PubMed.
- H. Spikes, Tribol. Lett., 2015 DOI:10.1007/s11249-015-0589-z.
- H. Spikes, Tribol. Lett., 2004, 17, 469–489 CrossRef CAS.
- C. Grossiord, K. Varlot, J. M. Martin, Th. Le Mogne, C. Esnouf and K. Inoue, Tribol. Int., 1998, 31, 737–743 CrossRef CAS.
- D. N. Khaemba, A. Neville and A. Morina, RSC Adv., 2016, 6, 38637–38646 RSC.
- D. N. Khaemba, F. Jarnias, B. Thiebaut, A. Neville and A. Morina, J. Phys. D: Appl. Phys., 2017, 50, 85302 CrossRef.
- A. Morina, A. Neville, M. Priest and J. H. Green, Tribol. Lett., 2006, 24, 243–256 CrossRef CAS.
- M. De Feo, C. Minfray, M. I. De Barros Bouchet, B. Thiebaut, T. Le Mogne, B. Vacher and J. M. Martin, Tribol. Int., 2015, 92, 126–135 CrossRef CAS.
- M. De Feo, C. Minfray, M. I. De Barros Bouchet, B. Thiebaut, T. Le Mogne and J. M. Martin, Wear, 2016, 348–349, 116–125 CrossRef CAS.
- M. I. De Barros Bouchet, J. M. Martin, Th. Le-Mogne and B. Vacher, Tribol. Int., 2005, 38, 257–264 CrossRef CAS.
- D. Jose, M. De Feo, C. Minfray and M. Cobian, 42nd Leeds-Lyon Symposium on Tribology, 2015 Search PubMed.
- T. Onodera, Y. Morita, A. Suzuki, R. Sahnoun, M. Koyama, H. Tsuboi, N. Hatakeyama, A. Endou, H. Takaba, C. A. Del Carpio and M. Kubo, Tribology Online, 2008, 3, 80–85 CrossRef.
- M. Kano, Y. Yasuda and J. Ye, Lubr. Sci., 2004, 17(1), 95–103 CrossRef CAS.
- D. N. Khaemba, F. Jarnias, B. Thiebaut, A. Neville and A. Morina, J. Phys. D: Appl. Phys., 50(8), 085302 CrossRef.
- K. C. Mutyala, H. Singh, J. A. Fouts, R. D. Evans and G. L. Doll, Tribol. Lett., 2016, 61 Search PubMed.
- S. C. Tung and H. Gao, Wear, 2003, 255, 1276–1285 CrossRef CAS.
- J. Landa, Ind. Lubr. Tribol., 2007, 59, 217–229 CrossRef.
- A. Skopp, N. Kelling, M. Woydt and L. M. Berger, Wear, 2007, 262, 1061–1070 CrossRef CAS.
- M. Woydt, Tribol. Lett., 2000, 8, 117–130 CrossRef CAS.
- M. N. Gardos, Tribol. Lett., 2000, 8, 65–78 CrossRef CAS.
- P. Ernst, SAE International Journal of Engines, 2012, 5 DOI:10.4271/2012-01-1992.
- P. Ernst and B. Distler, SAE [Tech. Pap.], 2012, 4, 1–16 Search PubMed.
- B. J. Hamrock and D. Dowson, J. Lubr. Technol., 1976, 1976(99), 15 Search PubMed.
- E. Schmidt, F. Weill, G. Meunier and A. Levasseur, Thin Solid Films, 1994, 245, 34–39 CrossRef CAS.
- B. Dacre and C. Bovington, ASLE Trans., 1982, 25, 546–554 CrossRef CAS.
- G. J. Molina, M. J. Furey, C. Kajdas and N. Steika, Fasc.VIII (Tribology), The Annals of Univ. Dunarea de Jos, Romania, 2005, pp. 17–23 Search PubMed.
- G. J. Molina, M. J. Furey, A. L. Ritter and C. Kajdas, Wear, 2001, 249, 214–219 CrossRef CAS.
- G. J. Molina, M. J. Furey, A. L. Ritter, C. Kajdas, Proceedings 9th Nordic Symposium on Tribology NORDTRIB 2000, 2000, pp. 11–14. Search PubMed.
- K. Nakayama, Wear, 2004, 178, 61–67 CrossRef.
- K. Nakayama and H. Hashimoto, Tribol. Int., 1996, 29, 385–393 CrossRef CAS.
- C. Kajdas, Tribol. Int., 2016, 7–9 Search PubMed.
- C. Kajdas, A. Kulczycki and D. Ozimina, Tribol. Int., 2016 DOI:10.1016/j.triboint.2016.08.022.
- M. N. Gardos, Tribol. Trans., 1988, 31, 427–436 CrossRef CAS.
- A. C. Padilha, H. Raebiger, A. R. Rocha and G. M. Dalpian, Sci. Rep., 2016, 6, 28871 CrossRef CAS PubMed.
- F. H. Stott, J. E. Breakell and R. C. Newman, Corros. Sci., 1990 DOI:10.1016/0010-938X(90)90005-P.
- M. J. Frisch, G. W. Trucks, H. B. Schlegel, G. E. Scuseria, M. A. Robb, J. R. Cheeseman, G. Scalmani, V. Barone, G. A. Petersson, H. Nakatsuji, X. Li, M. Caricato, A. Marenich, J. Bloino, B. G. Janesko, R. Gomperts, B. Mennucci, H. P. Hratchian, J. V. Ortiz, A. F. Izmaylov, J. L. Sonnenberg, D. Williams-Young, F. Ding, F. Lipparini, F. Egidi, J. Goings, B. Peng, A. Petrone, T. Henderson, D. Ranasinghe, V. G. Zakrzewski, J. Gao, N. Rega, G. Zheng, W. Liang, M. Hada, M. Ehara, K. Toyota, R. Fukuda, J. Hasegawa, M. Ishida, T. Nakajima, Y. Honda, O. Kitao, H. Nakai, T. Vreven, K. Throssell, J. A. Montgomery Jr, J. E. Peralta, F. Ogliaro, M. Bearpark, J. J. Heyd, E. Brothers, K. N. Kudin, V. N. Staroverov, T. Keith, R. Kobayashi, J. Normand, K. Raghavachari, A. Rendell, J. C. Burant, S. S. Iyengar, J. Tomasi, M. Cossi, J. M. Millam, M. Klene, C. Adamo, R. Cammi, J. W. Ochterski, R. L. Martin, K. Morokuma, O. Farkas, J. B. Foresman, and D. J. Fox, Gaussian 09, Revision D.01, Gaussian, Inc., Wallingford CT, 2016 Search PubMed.
|
This journal is © The Royal Society of Chemistry 2018 |
Click here to see how this site uses Cookies. View our privacy policy here.