DOI:
10.1039/C8RA00862K
(Paper)
RSC Adv., 2018,
8, 9202-9210
The effect of co-sensitization methods between N719 and boron dipyrromethene triads on dye-sensitized solar cell performance†
Received
28th January 2018
, Accepted 21st February 2018
First published on 2nd March 2018
Abstract
A boron dipyrromethene (BODIPY) featuring triphenylamine triad, BD, has been synthesized as a co-sensitizer in dye-sensitized solar cells (DSCs). The optical and electrochemical properties of BD have been characterized using UV-vis spectroscopy and cyclic voltammetry. DSCs containing co-sensitizers, N719 and BD, have been prepared in two procedures using co-deposition and stepwise deposition. The influences of the staining processes, co-deposition and stepwise deposition on dye loading, dye dispersion on a TiO2 photoanode and DSC performance have been investigated using FTIR, SEM-EDS, I–V test and IPCE measurement, respectively. We found that stepwise co-sensitization provided higher solar cell efficiency, compared to those stained with a co-deposition method. N719/5% BD showed the highest power conversion efficiency of 5.14%. Interestingly, the enhanced device efficiency was 66% higher than that of a device containing the single N719 dye.
1. Introduction
Dye-sensitized solar cells (DSCs) are one of the promising photovoltaic technologies due to their cheaper cost, thinner thickness, flexibility and easy fabrication as compared to silicon solar cells.1–3 Although dye-sensitized solar cells are still in the research phase, integration of DSCs within buildings or portable devices is capturing the community's interest. A typical DSC consists of a transparent electrode, dye-sensitizers adsorbed on TiO2 semiconductor, electrolyte and a counter electrode. DSC produces electricity by the following steps; (1) dye-sensitizers absorb light and generate exited electrons, (2) the excited electrons transfer to the conduction band of TiO2 and further transport to the anode and (3) the oxidized dyes are regenerated by the iodide redox couple, while the positive charge transported to the cathode.1,2,4 It is known that the dye-sensitizer is essential to the performance of DSCs. Broad absorption of sun spectrum with high molar absorptivity and suitable energy levels of the dye-sensitizer are keys to obtain large amount of photon flux and efficient electron transporting to the TiO2 layer which influences on the overall efficiency of solar cells.1,5,6 Thus, many efforts have been focused on the development of dye-sensitizers. The dye-sensitizers can be classified in two groups, the metal complex sensitizer and metal-free organic sensitizer. The first group consists of metal ions including Ru, Ni and Co complex with bipyridine or terpyridine ligands and adsorbing group.1 The most famous classical dyes are N3, N719 and N749.4,5 Such dyes provided remarkable efficiency above 10% due to their wide absorption range that provide high Jsc value. However, absorption of metal complex sensitizers is not optimal because they inherently have moderate molar extinction coefficient at the long wavelength region which limit amount of photon harvesting.5,7,8 On the other hand, metal-free organic sensitizer possessing high molar extinction coefficient have gained considerable attention. Organic dye-sensitizers featuring donor–acceptor (D–A) molecular structures have been employed to broaden the light absorption properties.9,10 Most of organic sensitizers are based on the triarylamine, carbazole, squaraine, boron dipyrromethene, porphyrin and phthalocyanine structures.11–18 However, most of power conversion efficiency of DSCs containing organic sensitizers are still lower than those of Ru dyes.5,8
Alternative approach that could fulfill the drawback of using single dye sensitizer is co-sensitized titania by two or more dyes to achieve optimal absorption. In the multiple dyes system, the low absorption of Ru dye at the long wavelength will be complemented with the intense absorption of organic dye, resulting in panchromatic absorption and leading to enhance in device efficiency.2,5,10,19 For example, Han et al.20 developed a cis-configuration squaraine dye (HSQ1) to co-sensitized with N3 dye. DSC based on HSQ1 + N3 showed improved Jsc and Voc compared to those of DSC based on SQ1 + N3 due to broader IPCE spectrum and suppress aggregation of N3. Chen et al.21 synthesized porphyrin dye, denoted as HD18, and co-sensitized with N719 for the enhancement of the spectral response. The combination of these two sensitizers showed full color spectrum, resulting increasing of overall PCE. Recently, Hu et al.22 reported that co-sensitization of polyphenylene with N719 can enhanced PCE due to the increase of light absorption and the retardation of electron recombination and dye aggregation. These examples of co-sensitized DSCs suggested that suitable structure of organic sensitizer should be considered to avoid aggregation among dyes on the TiO2 surface and retardation of electron recombination.20–22
Despite the co-sensitization of Ru dyes with NIR organic dyes including porphyrins and squaraines have been reported, another interesting dye is boron dipyrromethene (BODIPY). BODIPY has gained recognition as a building block of NIR dye for using in organic solar cells (OSCs) and DSCs.16,23–29 The BODIPY with appropriate structural modification can provide tunable panchromatic absorption from 500–800 nm.29–33 In addition, DSC containing Ru dye and BODIPY sensitizer have been rarely reported. It was apparent from our previous study that D–π–A–π–D structure of the BODIPY containing ethynyl triphenylamine have effective electronic communication between the donor triphenylamine (TPA) and acceptor BODIPY via the ethynyl linker.34 As a result, the BODIPY triad exhibited broad absorption up to red edge region. Thus, the BODIPY triad is an attractive molecular platform to use as an organic co-sensitizer to complement light absorption window of Ru sensitizer such as N719. In our molecular design as shown in Fig. 1. We reengineered the TPA–BODIPY–TPA by functionalized an cyanoacrylic acid anchoring group at the meso-position of the BODIPY core because theoretical studies reported that the meso-position of BODIPY unit is more suitable for electron injection to the TiO2 conduction band.16,31
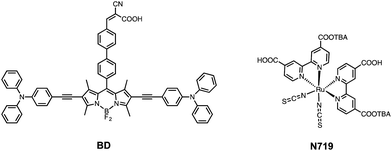 |
| Fig. 1 The molecular structure of BODIPY sensitizer (BD) and N719. | |
We are noticed that similar BODIPY triad structures, distyryl triphenylamine-BODIPY, where triphenylamine units are functionalized on 3-and 5-position of BODIPY, have been reported by Akkaya et al.25 and Feng et al.35 However, the performance of DSC containing co-adsorbents, BODIPY and N719, has not been demonstrated. Here, we reported synthesis and characterizations of BD sensitizer. In addition, two procedures to co-sensitized N719 with BD on TiO2 semiconductor is our interest. In the first procedure, N719 and BD were mixed together prior to co-deposit on the TiO2. In the second procedure, stepwise co-sensitization, N719 was first stained on the TiO2 layer, followed by immersion of BD on the sensitized TiO2. The DSCs efficiency were investigated and the influence of co-sensitization procedures on photovoltaic parameters and device performance were discussed.
2. Experimental sections
Instrumentations
1H-NMR, 13C-NMR spectra were measured in CDCl3 and MeOD solution at 500 MHz, and 125 MHz, respectively, on Bruker NMR spectrometers. The chemical shifts of NMR spectra were recorded using the residual proton and carbon signal of the deuterated solvent as the internal standard. The spectra were reported in ppm, all J values are in Hz, and peak multiplicities are given, the following abbreviations are used: s, singlet; d, doublet; m, multiplet. The optical spectra of BD solution were recorded on a Thermo Scientific UV-Genesys 10s spectrophotometer and the solid state optical spectra of dye-sensitized on TiO2 film were recorded on a Cary 5000, Agilent Technologies UV-vis-NIR spectrophotometer. Cyclic voltammetric (CV) measurements were carried out on a Metrohm Autolab potentiostat with an electrochemical system utilizing a three-electrode configuration consisting of a glassy carbon electrode, Pt electrode, a Ag/AgCl electrode as working electrode, counter electrode and reference electrode, respectively. The cyclic voltammograms of BODIPYs were recorded in anhydrous CH2Cl2 with 0.1 M tetrabutylammonium hexafluorophosphate (TBAPF6) at a scan rate of 50 mV s−1. The voltammogram was normalized to half-wave potential of ferrocene/ferrocenium redox couple (E1/2 (Fc/Fc+)) FTIR spectra were recorded on a Thermo Nicolet 6700 spectrometer. SEM micrograph was carried out using FEI Nova Nanosem 450. Energy dispersive X-ray spectroscopy (EDS) was recorded on Bruker Nano XFlash 6/3. The current density–voltage (J–V) curved were measured using Keithley 2400 source-measurement unit and a Newport 91150V solar simulator. The incident photon-to-current conversion efficiency (IPCE) measurement was performed on Oriel instrument (OPS-A500) with Xe lamp and Oriel 3502 optical chopper to give a monochromatic light source.
Materials
4-Iodobenzyl-meso-BODIPY (compound 1) and 4-ethynyltriphenylamine were synthesized according to reported literature.34 4-Formylphenylboronic acid pinacol ester, N-iodosuccinimide, copper iodide, tetrakis(triphenylphosphine)palladium(0) (Pd(PPh3)4), cyanoacetic acid were purchased from Aldrich. Anhydrous sodium sulfate, potassium carbonate, triethylamine, ammonium acetate, and glacial acetic acid were purchased from Fisher. HPLC grade dichloromethane, methanol, anhydrous N,N′-dimethylformamide, toluene and acetonitrile were purchased from Fisher. For DSC fabrication materials, FTO glass, titanium isopropoxide (TTIP), anhydrous acetonitrile and tert-butanol were purchased from Aldrich. TiO2 (P25) was purchased from PlasmaChem, Germany. N719, HI-30 electrolyte and Pt paste were purchased from Solaronix. NMR solvents include deuterated chloroform (CDCl3) and deuterated methanol (MeOD) were purchased from Cambridge Isotope Laboratories. Silica gel for column chromatography was purchased from Silicycle. All chemicals were used as received.
Synthesis detail
Synthesis of (1,1′-biphenyl)-4-carbaldehyde-meso-BODIPY, compound 2. 4-Iodobenzyl-meso-BODIPY (0.48 g, 1.0 mmol) and 4-formylphenylboronic acid pinacol ester (0.3 g, 1.2 mmol) were dissolved in toluene (20 mL). The solution was degassed with N2 for 10 minutes. Pd(PPh3)4 (0.1 g, 0.1 mmol) were added, followed by addition of 2 M K2CO3 (5 mL). The reaction mixture was refluxed under N2 for 24 hours. The reaction mixture extracted with dichloromethane (DCM) and water. The organic layers were dried by sodium sulfate (Na2SO4) and evaporated in vacuo. The crude mixture was purified by column chromatography on silica gel using DCM/hexane as an eluent to give the product 2 as orange solid (0.25 g, 42%) 1H-NMR (500 MHz, CDCl3): δ (ppm), 10.09 (s, 1H), 8.01 (d, J = 10 Hz, 2H), 7.86 (d, J = 10 Hz, 2H), 7.81 (d, J = 10 Hz, 2H), 7.42 (d, J = 10 Hz, 2H), 6.00 (s, 2H), 2.57 (s, 6H), 1.44 (s, 6H) 13C-NMR (125 MHz, CDCl3): δ (ppm), 191.75, 155.75, 145.89, 142.97, 140.87, 140.27, 135.61, 135.29, 131.32, 130.35, 128.86, 127.93, 127.66, 121.36, 14.57 MALDI-TOF MS (m/z) calculated for C26H23BF2N2O [M + Na], 451.1872; found 451.1889.
Synthesis of ((1,1′-biphenyl)-4-carbaldehyde-meso-2,6-diiodo-BODIPY), compound 3. Compound 2 (0.20 g, 0.46 mmol) was dissolved in DCM (20 mL) and then degassed with N2 for 5 minutes. A solution of N-iodosuccinimide (NIS) (0.42 g, 1.86 mmol) in dimethylformamide (DMF) (4 mL) was added dropwise to the solution mixture. The reaction mixture was stirred under N2 at room temperature and was kept in the dark for 2 days. After that, the crude mixture was extracted with DCM and water. The organic phase was dried over Na2SO4 and concentrated in vacuo. The crude product was then purified by column chromatography over silica with DCM/hexane as an eluent to give the product 3 as red solid (0.20 g, 63%) 1H-NMR (500 MHz, CDCl3): δ (ppm), 10.10 (s, 1H), 8.02 (d, J = 5 Hz, 2H), 7.86 (d, J = 5 Hz, 2H), 7.84 (d, J = 10 Hz, 2H), 7.40 (d, J = 10 Hz, 2H), 2.66 (s, 6H), 1.46 (s, 6H) 13C-NMR (125 MHz, CDCl3): δ (ppm), 191.69, 157.09, 145.57, 145.18, 140.91, 140.2, 135.77, 134.95, 131.21, 130.40, 128.71, 128.24, 127.72, 17.20, 16.06. MALDI-TOF MS (m/z) calculated for C26H21BF2I2N2O [M + Na], 679.9804; found 702.9674.
Synthesis of (1,1′-biphenyl)-4-carbaldehyde-meso-2,6-bis(ethynyltriphenylamine)-BODIPY, compound 4. Compound 3 (0.20 g, 0.29 mmol) was dissolved in dry toluene (20 mL). The reaction mixture was purged with N2 for 15 minutes. Pd(PPh3)4 (0.03 g, 0.03 mmol) and copper iodide (CuI) (0.005 g, 0.03 mmol) were added, followed by addition of 4-ethynyltriphenylamine (0.3 g, 1 mmol). The reaction mixture was stirred at room temperature for 15 minutes, followed by addition of Et3N (2 mL). The reaction mixture was refluxed under N2 protection for 36 hours. The mixture was filtered with Celite and the solvent was removed using rotary evaporator. The reaction mixture was poured with 50 mL of water and extracted with DCM. The combined organic layers were dried over sodium sulfate and concentrated under reduced pressure to afford the crude mixture. The residue was then purified by column chromatography over silica gel with DCM/hexane as an eluent to provide the product 4 as deep blue solid (0.11 g, 36%) 1H-NMR (500 MHz, CDCl3): δ (ppm), 10.10 (s, 1H), 8.02 (d, J = 5 Hz, 2H), 7.88 (d, J = 5 Hz, 2H), 7.85 (d, J = 10 Hz, 2H), 7.44 (d, J = 10 Hz, 2H), 7.30–7.24 (m, 9H), 7.10–6.97 (m, 19H), 2.72 (s, 6H), 1.57 (s, 6H) 13C-NMR (125 MHz, CDCl3): δ (ppm) 191.73, 158.58, 147.86, 147.13, 145.73, 143.19, 141.21, 120.63, 132.25, 130.38, 129.36, 128.81, 128.08, 127.71, 124.89, 123.53, 122.35, 116.12, 96.86, 80.61, 13.72, 13.59. MALDI-TOF MS (m/z) calculated for C66H49BF2N4O [M + Na] 985.3967; found 985.3943.
Synthesis of ([1,1′-biphenyl]-4-yl-2-cyanoacrylic acid-meso-2,6-bis(ethynyltriphenylamine)-BODIPY), compound 5 (BD). Compound 4 (0.07 g, 0.07 mmol) was dissolved in chloroform (10 mL) and acetic acid (3 mL). Cyanoacetic acid (0.02 g, 0.57 mmol) and ammonium acetate (0.02 g, 0.057 mmol) were added to a solution. The reaction mixture was refluxed under N2 atmosphere for 12 hours and then was allowed to cool at room temperature. After that, water (15 mL) was added to the crude mixture, followed by extraction with CHCl3. The organic layers were dried over sodium sulfate. The crude product was concentrated under reduced pressure and was purified on silica gel using MeOH and CHCl3 as eluents. The dark blue powder was obtained as product 5, BD (0.04 g, 55%) 1H-NMR (500 MHz, CDCl3/MeOD): δ (ppm), 8.24 (s, 1H), 8.05 (d, 2H), 7.82 (m, 4H), 7.38 (d, 2H), 7.32–6.92 (m, 28H), 2.64 (s, 6H), 1.53 (s, 6H) 13C-NMR (125 MHz, CDCl3/MeOD): δ (ppm), 158.71, 148.24, 147.45, 144.16, 143.69, 141.85, 140.93, 134.87, 132.52, 131.70, 131.44, 129.65, 129.11, 128.24, 127.99, 125.21, 123.86, 122.57, 116.99, 116.35, 97.27, 80.79, 13.80 MALDI-TOF MS (m/z) calculated for C69H50BF2N5O2 [M + H], 1029.4026 found 1030.4116.
Device fabrication and photovoltaic measurements
To fabricate the dye-sensitized solar cells device, the TiO2 electrodes were prepared following the previous reported procedure.36 In brief, the FTO glass sheet (∼7 Ω cm−2) was cut into 2.5 × 2.5 cm2. FTO glass plates were pretreated with 0.2 M HCl in an ultrasonic bath for 15 min, followed by washing with DI water under sonication for 10 min, twice. After that, the FTO substrates were sonicated with isopropanol (IPA) for 20 min and then dried by purging with N2 gas. Then, TiOx sol–gel (10 μL) was coated on the FTO plates using the rapid convection deposition technique with a deposition rate of 600 μm s−1. The TiOx layer was then gradually heated under air atmosphere at 500 °C for 1 h with a heating rate of 10 °C min−1. After that, the doctor blade technique was used to deposit a mesoporous layer of TiO2, a mixture of 0.10 g of TiO2 (P25) in 0.429 μL of IPA and 10 μL of titanium isopropoxide (TTIP). Subsequently, TiO2 paste was sintered at 500 °C for 1 h (10 °C min−1). Dye solutions of BD (0.39 mM) and N719 (0.33 mM) in acetonitrile/tert-butanol (1
:
1 v/v) have been prepared. The co-sensitized of N719 with BD have been prepared in two methods. The first method, co-deposition, solutions of N719 and BD have been mixed by varied BD concentration as 5% and 10%, respectively. The TiO2 photoanodes were immersed into dye solutions and stored at 80 °C for 4 h. The dye stained electrodes were rinsed with acetonitrile several times and dried at 80 °C for 30 min. For the second method, the stepwise deposition, the prepared TiO2 electrodes were immersed in 0.33 mmol of N719 solution in acetonitrile/tert-butanol (1
:
1 v/v) at 80 °C for 4 h and then rinsed with anhydrous acetonitrile for several times. After that, the sensitized photoanodes were dried at 80 °C for 30 minutes to remove the remaining solvent. The sensitized TiO2 was then coated with BD by dipping in 5% and 10% of BD solutions, respectively, at 80 °C for another 1 h. Finally, the sensitized films were rinsed with acetonitrile and then dried at 80 °C for 30 min. Counter electrodes were prepared by coating platinum (Pt) paste on drilled and cleaned FTO glass plates using doctor blade technique and then calcined at 450 °C for 1 h with a heating rate of 10 °C min−1. The Pt counter electrode and the dye-sensitize electrode were sandwiched together using Surlyn film as the frame. The gap between electrodes was sealed by melting the Surlyn film. Next, 10 μL of electrolyte solution consisting of iodide/triiodide, HI-30, was injected through the drilled holes on counter electrode. Finally, the holes were sealed with the Kapton tape to prevent the leakage of liquid electrolyte. The active cell area of DSCs was fixed to be 0.40 cm2. The DSC performance was characterized using the current density–voltage (J–V) plots. The photo current was measured under AM 1.5G illumination 100 mW cm−2. The light intensity was calibrated with reference monocrystalline silicon cell.
Dye loading measurement
The adsorption amount of single dye and mixed dyes have been measured following procedure report in literatures.37,38 Briefly, N719 on TiO2 layer was desorbed using 0.1 M of NaOH in EtOH/H2O (1
:
1 v/v). Then, BD was extracted using CHCl3/MeOH (1
:
1 v/v). The UV-vis spectrum of each sample solution was compared with the known concentration of N719 and BD. The dye loading was determined using Beer's law.
3. Results and discussion
Synthesis
The BODIPY sensitizer was synthesized according to the synthesis protocol depicted in Scheme 1. The precursor iodo-BODIPY (1) was prepared following the procedure in our recent study.34 BODIPY (1) was coupled with 4-formylphenylboronic acid pinacol ester to yield (1,1′-biphenyl)-4-carbaldehyde-meso-BODIPY (2). Then iodination at the 2 and 6 positions of BODIPY core provided 2,6-diiodo-BODIPY (3) in a good yield of 63%. Next, compound 3 was coupled with 4-ethynyltriphenylamine using Sonogashira cross coupling reaction to provide corresponding TPA–
–BODIPY–
–TPA (4) in 36% yield. Finally, the desired BODIPY sensitizers, BD, was obtained by Knoevenagel condensation between the aldehyde moiety of 4 with cyanoacetic acid. All compounds were purified by column chromatography. The molecular structures were confirmed using NMR spectroscopy and mass spectrometry. The 1H-NMR spectrum of 4 showed corresponding aldehyde proton at 10.09 ppm, aromatic protons of triphenylamine rings at 7.30–6.97 ppm. The spectrum of BD showed vinylic proton at 8.24 ppm, indicating that aldehyde was successfully converted to cyanoacrylate acid. This also confirm with mass spectrometry (see ESI†).
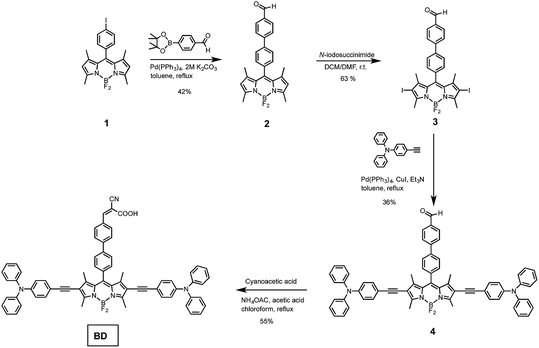 |
| Scheme 1 Synthetic route of BODIPY (BD) sensitizer. | |
Optical properties of BD
Absorption spectra of BD in solution and in solid are shown in Fig. 2. The optical parameters including absorption maxima, molar extinction coefficient and absorption onset were summarized in Table 1. The blue solution of BD dye exhibited broad absorption in the range of 300–700 nm. The two intense absorption bands at 345 and 598 nm are attributed to π–π* transition of the triphenylamine unit and the internal charge transfer (ICT) transition from the triphenylamine donor to the BODIPY unit. The molar extinction coefficient (ε) of the lower energy band was calculated to be 5.4 × 105 M−1 cm−1. This high value would benefit to photon harvesting process. The onset wavelength was at 700 nm, indicating red absorption with the optical energy bandgap of 1.77 eV. When BD was adsorbed onto a transparent TiO2 electrode, λmax was shifted from 598 nm in solution to 606 nm on TiO2. The bathochromic shift is due to greater electronic interaction between the dye and TiO2 surface.14
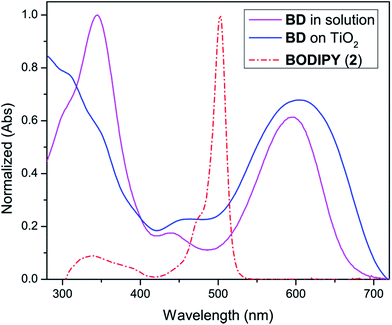 |
| Fig. 2 Normalized UV-vis of BD in dichloromethane (pink) and adsorbed on TiO2 film (blue) and the BODIPY core (3) (red). | |
Table 1 Summary of optical absorption maxima (solution and solid state), absorption coefficients, absorption onset, absorption maxima and the optical bandgaps of BD
Dye |
λmax (solution) (nm) |
εmax (×105 M−1 cm−1) |
λmax (on TiO2) (nm) |
λonset (solution) (nm) |
Eg,opt (eV) |
BD |
345, 598 |
8.7, 5.4 |
606 |
700 |
1.77 |
Electrochemical characterization of BD
To evaluate the feasibilities of electron injection and dye regeneration process, the electrochemical properties of BD including redox properties, HOMO and LUMO of BD were investigated by cyclic voltammetry. The voltammogram of BD is shown in Fig. 3 and the electrochemical data are summarized in Table 2. As seen in Fig. 3, BD exhibited the low oxidation potential of 0.53 eV due to the electron rich nature of triphenylamine moiety. This corresponds to the HOMO energy of −5.33 eV which is lower than the redox potential of triiodide redox couple (−4.90 eV), and thus facilitated electron transfer from I− to the BD in dye regeneration process. A reduction potential of BD was approximately −1.10 eV and was calculated to be −3.70 eV of LUMO energy level. The LUMO level of BD (−3.70 eV) is higher than that of the conduction band edge of TiO2 (−4.20 eV) and the difference is 0.5 eV. Therefore, excited electron from BD can be thermodynamically injected to the conduction band of TiO2.
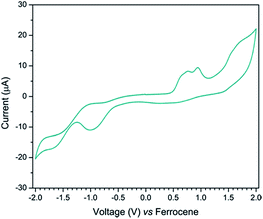 |
| Fig. 3 Cyclic voltammogram of BD. | |
Table 2 Summary of electrochemical data of BD
Dye |
Eoxa (V) |
HOMOb (eV) |
Ereda (V) |
LUMOb (eV) |
Eg,elecc (eV) |
The oxidation and reduction potentials were approximated from the oxidation and reduction peaks. The HOMO and LUMO energy levels were calculated using the following relationship; HOMO = −(Eox,onset + 4.8) eV and LUMO = −(Ered,onset + 4.8) eV.39 Eg,elec = ELUMO − EHOMO. |
BD |
+0.53 |
−5.33 |
−1.10 |
−3.70 |
1.63 |
FTIR characterization
FTIR measurements have been conducted to investigate interaction of individual dyes (BD and N719) and co-sensitized dye on TiO2 film. From Fig. 4a, BD and N719 exhibited strong peak around 1700 cm−1 that correlate to C
O stretching of the carbonyl group. The peaks at 1593 cm−1 and 1317 cm−1 represent asymmetric and symmetric vibration of carboxylate (–COO−), respectively. The carboxylic peak of BD was higher than those of carboxylate peaks, suggesting that large amount of BD was not bound to the TiO2 surface.
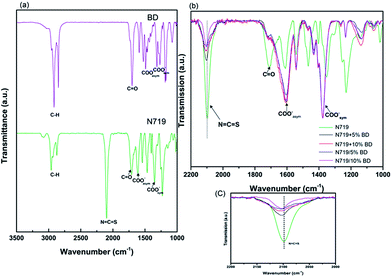 |
| Fig. 4 FTIR Spectra (a) BD and N719 (b) co-sensitized N719 + BD on TiO2 film and (c) expansion of N C S bands. | |
FTIR-spectra of different co-dyeing process were depicted in Fig. 4b, coding as N719 + 5% BD and N719 + 10% BD, were prepared via co-deposition method. While sample coding as N719/5% BD and N719/10% BD were prepared via stepwise procedure. FTIR-spectra of different co-dyeing process were depicted in Fig. 4b. The two strong bands at 1605 cm−1 and 1375 cm−1 were assigned to νCOOasym− and νCOOsym−, respectively.40 The difference (Δν) of νCOOasym− and νCOOsym− was 230 cm−1, suggesting the bidentate chelating binding of N719 to the TiO2 surface.41,42 The intensity of those two peaks were about the same, suggesting that the total dye molecules adsorbed on the TiO2 surface were similar. The peak at 2103 cm−1 was assigned to the N
C vibration mode from the NCS group of N719. Interestingly, we observed that NCS peak of co-sensitized N719 and BD was slightly shifted to 2117–2121 cm−1 and the peak intensity was decreased, as compared to N719 (Fig. 4c). This could be due to the different binding mechanism of N719 in which NCS interact to the TiO2 surface. This was also suggested from previous studies by Singh et al.42 and Johansson et al.43
Dye loading
Since N719 and BD have different molecular structure and number of anchoring group, we studied the dye loading capability and change of composition in different co-sensitization process. The adsorption amount of dyes has shown in Fig. 5. The BD cell exhibited dye loading amount of 1556.2 nmol cm−2, while the N719 cell showed considerable lower dye up-taking amount of 61 nmol cm−2. The significant high amount of BD was due to aggregation of dye on TiO2 layer as we observed free carboxylic peak in FTIR result. For co-dyeing, N719 + 5% BD and N719 + 10% BD, as the BD concentration increased, the amount of BD was increased from 65 nmol cm−2 to 140 nmol cm−2. However, the amount of N719 was fixed to 43.2 nmol cm−2. This indicated the competitive adsorption between BD and N719 on the limited sites of TiO2 surface. For the stepwise deposition, in which the photoanode was immersed in N719 solution, then dipped in BD solution, we found that the BD contents were significantly decreased to 1.8 and 8.8 nmol cm−2, respectively. The total dye loading of the stepwise staining system was about 61 nmol cm−2, that was similar to the individual N719.
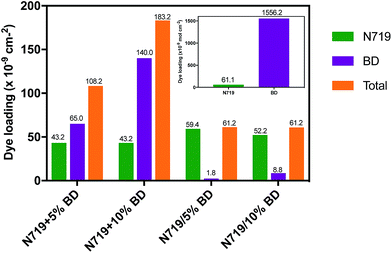 |
| Fig. 5 Dye loading of individual BD and N719 cells and co-sensitize cells using co-deposition and stepwise deposition method. | |
In addition, SEM-EDS has been conducted to investigate the assemble morphology and chemical composition of co-sensitized DSCs. SEM cross-sectional image of co-sensitized TiO2 on FTO glass and energy dispersive X-ray spectroscopy analysis (EDS) were illustrated in Fig. 6. The SEM cross sectional image of co-sensitized TiO2 on FTO glass has shown in Fig. 6a. EDS measurement has been conducted to investigate N719 and BD distribution on the TiO2 layer. The ruthenium (Ru) and fluorine (F) signals have been selected to qualitatively analyzed N719 and BD distribution in the TiO2 matrix. As seen from Fig. 6b–g, the mapping images confirmed successful sensitization of N719 and BD. The device prepared from the co-dyeing process exhibited much higher signal intensity of F signal (cyan color) than the stepwise dyeing method. This implied high BD dispersion on TiO2 layer which was consistent to the dye loading result.
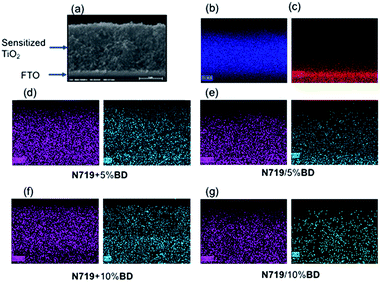 |
| Fig. 6 Cross-sectional image of sensitized TiO2 (a), EDS mapping images of (b) Ti, (c) Sn, (d) Ru and F of N719 + 5% BD (e) Ru and F of N719/5% BD (f) Ru and F of N719 + 10% BD and (g) Ru and F of N719/10% BD. | |
Photovoltaic performance
DSCs containing BD, N719 and co-sensitization of N719 and BD were fabricated. The current density versus voltage (J–V) characteristic curves were presented in Fig. 7. The photovoltaic parameters of DSCs including photocurrent density (Jsc), open circuit voltage (Voc), fill factor (FF) and power conversion efficiency (PCE) were summarized in Table 3. Photovoltaic performance based on DSC containing BD showed a Jsc of 1.26 mA cm−2, a Voc of 550 mV and an FF of 0.64, resulting in power conversion efficiency (PCE) of 0.45%. The poor performance is caused by low Jsc and Voc values. This caused by aggregation of BD on the TiO2 surface that increased charge recombination and reduced electron injection to the TiO2 electrode.44–46 The DSC containing standard N719 sensitizer exhibited the Jsc of 7.09 mA cm−2, a Voc of 640 mV and an FF of 0.67, yielding PCE of 3.09%. We noticed that this is considered low PCE as compared to some report in the literatures. This could be attributed to variation of layer thickness, active area and types of electrolyte and electrode materials.
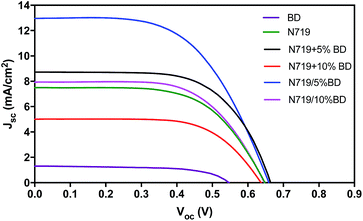 |
| Fig. 7 J–V characteristics of DSCs containing BD, N719 and co-sensitized N719 + BD solar cells. | |
Table 3 Photovoltaic performance of DSCs based on BD, N719 and co-sensitized N719 with BDa
Dyes |
Sensitization methods |
Amount of N719 (×10−9 mol cm−2) |
Amount of BD (×10−9 mol cm−2) |
Jsc (mA cm−2) |
Voc (V) |
FF |
PCE (%) |
Light source: 100 mW cm−2, AM 1.5G simulated solar light; working area: 0.4 cm2. |
BD |
|
|
1556.2 |
1.26 |
0.55 |
0.64 |
0.45 |
N719 |
|
61.1 |
|
7.09 |
0.64 |
0.67 |
3.09 |
N719 + 5% BD |
Co-deposition |
43.2 |
65.0 |
8.71 |
0.67 |
0.66 |
3.85 |
N719 + 10% BD |
43.2 |
140 |
5.02 |
0.64 |
0.66 |
2.10 |
N719/5% BD |
Stepwise deposition |
59.4 |
1.8 |
12.98 |
0.66 |
0.60 |
5.14 |
N719/10% BD |
52.2 |
8.8 |
7.95 |
0.63 |
0.63 |
3.24 |
Next, we prepared co-sensitized N719 and BD DSCs using co-deposition method. The PCE of N719 + 5% BD and N719 + 10% BD were 3.85% and 2.01%, respectively (Table 3). The efficiency of N719 + 5% BD was raised by 24.6% in comparison with that of N719 (PCE = 3.09%). The enhanced efficiency was due to the increase of Jsc and Voc. However, we found that when increase concentration of BD to 10% (N719 + 10% BD), the Jsc was declined to 5.02 mA cm−2, resulting in PCE of 2.01%. The decreased efficiency could be correlate to higher amount of BD that accumulate on the TiO2 layer. This aggregation could trap free electrons travelling to the conduction band of TiO2. Interestingly, for stepwise sensitization DSCs, all devices have higher PCE compared to those of co-deposition DSCs as depicted in Fig. 5. PCEs of 5.14% and 3.24% were obtained from N719/5% BD and N719/10% BD, respectively. In addition, the trend of PCE was similar to the co-deposition DSCs in which N719/5% BD > N719/10% BD. The PCE of N719/5% BD exhibited a Jsc of 12.98 mA cm−2, a Voc of 660 mV and an FF of 0.6, corresponding to overall the best efficiency of 5.14%. This showed an improvement of 66% compared to the device sensitized with N719 and enhancement of 33% compared to the N719 + 5% BD using co-deposition method. The increased PCE is corresponding to much higher (Jsc) from 7.09 mA cm−2 of N719 cell to 12.98 mA cm−2.
To gain further insight into the attribution of BD and the co-sensitization methods on light harvesting and photocurrent generation, UV-vis absorption spectroscopy of sensitized TiO2 film and IPCE measurements have been conducted. It can be seen from Fig. 8a that co-adsorption of BD and N719 films have red-shifted absorption and could compensate the absorption of N719, at the range of 500–700 nm. Next, we performed IPCE experiment using 300 W Xe lamp with low-speed chopper to provide chromatic lights from 300–800 nm and measuring Jsc. From Fig. 8b, IPCE spectrum of BD was covered in the range of 300–550 nm with the lowest IPCE value of 10%. For the mixed dye system, the IPCE spectra were covered in broader range from 300–700 nm. The co-deposition devices exhibited the maximum value of IPCE as high as 37.8% and 26.91% for N719 + 5% BD and N719 + 10% BD, respectively. On the other hand, the stepwise sensitized devices, N719/5% BD and N719/10% BD, exhibited higher IPCE of 40.5% and 34.4% at 500 nm. The trend and increasing of IPCE values are in good agreement with Jsc results. It is worth noting that although stepwise dyeing cells contain less BD amount, it can be able to enhance the IPCE value. Although we do not fully understand the reason for greater Jsc values of stepwise co-sensitization, we assume that this may be due to cascade electron transfer from BD to N719 to titania since BD has higher LUMO energy level (−3.7 eV) than that of N719 (−3.9 eV).47,48
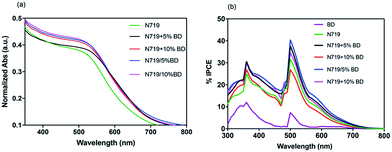 |
| Fig. 8 (a) UV-vis absorption spectra of dye adsorbed on TiO2 films and (b) IPCE spectra BD, N719 and co-sensitized N719 + BD solar cells. | |
4. Conclusions
In summary, BD was synthesized and used as co-adsorbent with N719 for DSC application. The influence of co-sensitization procedures, co-deposition and stepwise deposition, on DSC performance have been investigated. We found that small amount of BD sensitizer in co-deposition and stepwise deposition bear a positive influence on device efficiency. The stepwise deposition significantly increase Jsc values, resulting in higher PCEs. This is due to the improvement of IPCE and spectral response region at 500–700 nm. The best cell was achieved from N719/5% BD with PCE of 5.14%.
Conflicts of interest
There are no conflicts of interest to declare.
Acknowledgements
This work was supported by the National Nanotechnology Center (NANOTEC), NSTDA, Ministry of Science and Technology, through its program of Center of Excellence Network, the Thailand Research Fund (Grant no. TRG5880211) and the “KMUTT 55th Anniversary Commemorative Fund”. We would like to thank Dr Pisist Kumnorkaew at the National Nanotechnology Center, Thailand for IPCE instrument.
References
- A. Hagfeldt, G. Boschloo, L. C. Sun, L. Kloo and H. Pettersson, Chem. Rev., 2010, 110, 6595–6663 CrossRef CAS PubMed.
- F. Bella, C. Gerbaldi, C. Barolo and M. Gratzel, Chem. Soc. Rev., 2015, 44, 3431–3473 RSC.
- A. Shah, P. Torres, R. Tscharner, N. Wyrsch and H. Keppner, Science, 1999, 285, 692–698 CrossRef CAS PubMed.
- M. Gratzel, J. Photochem. Photobiol., C, 2003, 4, 145–153 CrossRef CAS.
- J. H. Yum, E. Baranoff, S. Wenger, M. K. Nazeeruddin and M. Gratzel, Energy Environ. Sci., 2011, 4, 842–857 CAS.
- B. E. Hardin, H. J. Snaith and M. D. McGehee, Nat. Photonics, 2012, 6, 162–169 CrossRef CAS.
- M. Gratzel, Acc. Chem. Res., 2009, 42, 1788–1798 CrossRef CAS PubMed.
- J. N. Clifford, E. Martinez-Ferrero, A. Viterisi and E. Palomares, Chem. Soc. Rev., 2011, 40, 1635–1646 RSC.
- Y. Z. Wu and W. H. Zhu, Chem. Soc. Rev., 2013, 42, 2039–2058 RSC.
- C. P. Lee, R. Y. Y. Lin, L. Y. Lin, C. T. Li, T. C. Chu, S. S. Sun, J. T. Lin and K. C. Ho, RSC Adv., 2015, 5, 23810–23825 RSC.
- J. Y. Wang, K. Liu, L. C. Ma and X. W. Zhan, Chem. Rev., 2016, 116, 14675–14725 CrossRef CAS PubMed.
- D. P. Hagberg, J. H. Yum, H. Lee, F. De Angelis, T. Marinado, K. M. Karlsson, R. Humphry-Baker, L. C. Sun, A. Hagfeldt, M. Gratzel and M. K. Nazeeruddin, J. Am. Chem. Soc., 2008, 130, 6259–6266 CrossRef CAS PubMed.
- J. F. Huang, J. M. Liu, L. L. Tan, Y. F. Chen, Y. Shen, L. M. Xiao, D. B. Kuang and C. Y. Su, Dyes Pigm., 2015, 114, 18–23 CrossRef CAS.
- A. Burke, L. Schmidt-Mende, S. Ito and M. Gratzel, Chem. Commun., 2007, 234–236 RSC.
- C. J. Qin, Y. Numata, S. F. Zhang, X. D. Yang, A. Islam, K. Zhang, H. Chen and L. Y. Han, Adv. Funct. Mater., 2014, 24, 3059–3066 CrossRef CAS.
- S. P. Singh and T. Gayathri, Eur. J. Org. Chem., 2014, 4689–4707 CrossRef CAS.
- C. J. Jiao, N. N. Zu, K. W. Huang, P. Wang and J. S. Wu, Org. Lett., 2011, 13, 3652–3655 CrossRef CAS PubMed.
- E. M. Barea, J. Ortiz, F. J. Paya, F. Fernandez-Lazaro, F. Fabregat-Santiago, A. Sastre-Santos and J. Bisquert, Energy Environ. Sci., 2010, 3, 1985–1994 CAS.
- M. D. Ye, X. R. Wen, M. Y. Wang, J. Iocozzia, N. Zhang, C. J. Lin and Z. Q. Lin, Mater. Today, 2015, 18, 155–162 CrossRef CAS.
- C. J. Qin, Y. Numata, S. F. Zhang, A. Islam, X. D. Yang, K. Sodeyama, Y. Tateyama and L. Y. Han, Adv. Funct. Mater., 2013, 23, 3782–3789 CrossRef CAS.
- S. Chang, H. D. Wang, L. T. L. Lee, S. Z. Zheng, Q. Li, K. Y. Wong, W. K. Wong, X. J. Zhu, W. Y. Wong, X. D. Xiao and T. Chen, J. Mater. Chem. C, 2014, 2, 3521–3526 RSC.
- L. H. Dong, Z. W. Zheng, Y. F. Wang, X. Li, J. L. Hua and A. G. Hu, J. Mater. Chem. A, 2015, 3, 11607–11614 CAS.
- A. Bessette and G. S. Hanan, Chem. Soc. Rev., 2014, 43, 3342–3405 RSC.
- M. Mao, J. B. Wang, Z. F. Xiao, S. Y. Dai and Q. H. Song, Dyes Pigm., 2012, 94, 224–232 CrossRef CAS.
- S. Erten-Ela, M. D. Yilmaz, B. Icli, Y. Dede, S. Icli and E. U. Akkaya, Org. Lett., 2008, 10, 3299–3302 CrossRef CAS PubMed.
- H. Cheema, R. Younts, B. Gautam, K. Gundogdu and A. El-Shafei, Mater. Chem. Phys., 2016, 184, 57–63 CrossRef CAS.
- I. Gonzalez-Valls, A. Mirloup, T. Le Bahers, N. Keller, T. Cottineau, P. Sautet and V. Keller, RSC Adv., 2016, 6, 91529–91540 RSC.
- N. Kaneza, J. T. Zhang, H. Y. Liu, P. S. Archana, Z. C. Shan, M. Vasiliu, S. H. Polansky, D. A. Dixon, R. E. Adams, R. H. Schmehl, A. Gupta and S. L. Pan, J. Phys. Chem. C, 2016, 120, 9068–9080 CAS.
- M. E. El-Khouly, S. Fukuzumi and F. D'Souza, ChemPhysChem, 2014, 15, 30–47 CrossRef CAS PubMed.
- A. Loudet and K. Burgess, Chem. Rev., 2007, 107, 4891–4932 CrossRef CAS PubMed.
- H. Lu, J. Mack, Y. C. Yang and Z. Shen, Chem. Soc. Rev., 2014, 43, 4778–4823 RSC.
- F. D'Souza, A. N. Amin, M. E. El-Khouly, N. K. Subbaiyan, M. E. Zandler and S. Fukuzumi, J. Am. Chem. Soc., 2012, 134, 654–664 CrossRef PubMed.
- M. E. El-Khouly, A. N. Amin, M. E. Zandler, S. Fukuzumi and F. D'Souza, Chem.–Eur. J., 2012, 18, 5239–5247 CrossRef CAS PubMed.
- S. Wanwong, P. Surawatanawong, S. Khumsubdee, S. Kanchanakungwankul and J. Wootthikanokkhan, Heteroat. Chem., 2016, 27, 306–315 CrossRef CAS.
- J. Zhang, F. T. Lu, S. B. Qi, Y. M. Zhao, K. P. Wang, B. Zhang and Y. Q. Feng, Dyes Pigm., 2016, 128, 296–303 CrossRef CAS.
- K. Yuwawech, J. Wootthikanokkhan, S. Wanwong and S. Tanpichai, J. Appl. Polym. Sci., 2017, 130, 45010 CrossRef.
- E. Dell'Orto, L. Raimondo, A. Sassella and A. Abbotto, J. Mater. Chem., 2012, 22, 11364–11369 RSC.
- G. H. Rao, A. Venkateswararao, L. Giribabu, L. Han, I. Bedja, R. K. Gupta, A. Islam and S. P. Singh, Phys. Chem. Chem. Phys., 2016, 18, 14279–14285 RSC.
- C. M. Cardona, W. Li, A. E. Kaifer, D. Stockdale and G. C. Bazan, Adv. Mater., 2011, 23, 2367–2371 CrossRef CAS PubMed.
- M. K. Nazeeruddin, R. Humphry-Baker, P. Liska and M. Gratzel, J. Phys. Chem. B, 2003, 107, 8981–8987 CrossRef CAS.
- G. B. Deacon and R. J. Phillips, Coord. Chem. Rev., 1980, 33, 227–250 CrossRef CAS.
- J. Singh, A. Gusain, V. Saxena, A. K. Chauhan, P. Veerender, S. P. Koiry, P. Jha, A. Jain, D. K. Aswal and S. K. Gupta, J. Phys. Chem. C, 2013, 117, 21096–21104 CAS.
- E. M. J. Johansson, M. Hedlund, H. Siegbahn and H. Rensmo, J. Phys. Chem. B, 2005, 109, 22256–22263 CrossRef CAS PubMed.
- M. Mao and Q. H. Song, Chem. Rec., 2016, 16, 719–733 CrossRef CAS PubMed.
- A. Mishra, M. K. R. Fischer and P. Bauerle, Angew. Chem., Int. Ed., 2009, 48, 2474–2499 CrossRef CAS PubMed.
- H. P. Lu, C. Y. Tsai, W. N. Yen, C. P. Hsieh, C. W. Lee, C. Y. Yeh and E. W. G. Diau, J. Phys. Chem. C, 2009, 113, 20990–20997 CAS.
- O. O. Ogunsolu, I. A. Murphy, J. C. Wang, A. Das and K. Hanson, ACS Appl. Mater. Interfaces, 2016, 8, 28633–28640 CAS.
- L. G. Wei, Y. L. Yang, R. Q. Fan, Y. Na, P. Wang, Y. W. Dong, B. Yang and W. W. Cao, Dalton Trans., 2014, 43, 11361–11370 RSC.
Footnote |
† Electronic supplementary information (ESI) available. See DOI: 10.1039/c8ra00862k |
|
This journal is © The Royal Society of Chemistry 2018 |
Click here to see how this site uses Cookies. View our privacy policy here.