DOI:
10.1039/C8RA03088J
(Paper)
RSC Adv., 2018,
8, 17101-17109
Direct speciation methods to quantify catalytically active species of AlCl3 in glucose isomerization†
Received
11th April 2018
, Accepted 25th April 2018
First published on 9th May 2018
Abstract
While homogeneous metal halides have been shown to catalyze glucose to fructose isomerization, direct experimental evidence in support of the catalytically active species remains elusive. Here, we integrate direct speciation methods with kinetics to provide strong evidence for the active species of AlCl3 in glucose–fructose isomerization in water. We investigate the effect of Lewis (AlCl3) and Brønsted (HCl) acids on aluminum hydrolysis and glucose conversion. We demonstrate the interplay between the acids using the Optimum Logic Inc. speciation model (OLI software). We measure aqueous aluminum species and protons through in situ and ex situ 27Al quantitative nuclear magnetic resonance (qNMR) and pH measurements, respectively, and quantify aluminum nanoparticles through a combination of inductively coupled plasma-mass spectrometry (ICP-MS), dynamic light scattering (DLS), and ultrafiltration. Direct speciation measurements correlated with the glucose isomerization rate indicate that the hydrolyzed Al(III) complex [Al(H2O)4(OH)2]1+ is the active species in glucose isomerization.
Introduction
The need to reduce greenhouse gas emissions and our dependence on fossil fuels has prompted considerable research in the production of fuels and chemicals from lignocellulosic biomass.1 Biomass is a promising renewable feedstock due to its abundance and its ability to capture CO2 from the atmosphere via photosynthesis.2 Among the top biomass-derived chemicals, 5-(hydroxymethyl)furfural (HMF) has gained significant interest as a platform chemical.2 HMF synthesis results from the hydrolysis of cellulosic biomass to glucose, glucose isomerization to fructose, and fructose dehydration to HMF.1 When the isomerization and dehydration steps occur in a single pot, dehydration drives the equilibrium-limited isomerization resulting in higher yields.3 This then requires an isomerization catalyst that is compatible with the Brønsted acid catalyst and high temperatures associated with the dehydration reaction. Currently, industrial applications use immobilized D-xylose ketoisomerase to catalyze the isomerization;4,5 however, these catalysts are expensive, operate under narrow conditions, and require highly pure glucose.6 As a result, research has turned to the development of chemo-catalytic processes to carry out glucose isomerization, starting with the pioneering work of Davis and co-workers on Sn-BEA zeolite that enable HMF production in a single pot.7,8
Metal halides are related homogeneous chemo-catalysts.3,9–12 Hu and co-workers compared the catalytic activity of AlCl3, CrCl3, and SnCl4 and found that CrCl3 exhibits the highest initial turnover frequency (TOF), while AlCl3 is the most selective catalyst to fructose.9 Mechanistic studies of glucose isomerization with AlCl3 and CrCl3 show that the isomerization proceeds through a 1,2 hydride transfer, and this step is rate-limiting according to kinetic-isotope effect measurements.3 Based on theoretical investigations,13 the catalytic species responsible for the isomerization likely exists as a bifunctional Lewis acidic/Brønsted base site, in which the Brønsted base (–OH) facilitates an initial proton transfer, activating the subsequent Lewis acid (metal center, M) catalyzed C2 → C1 hydride shift (Scheme 1).10 Direct experimental evidence in support of the catalytic species has though been lacking. This lack of understanding stems from a number of metal hydrolysis steps and self-condensation leading to oligomeric species and potentially metal nanoparticles.14–16
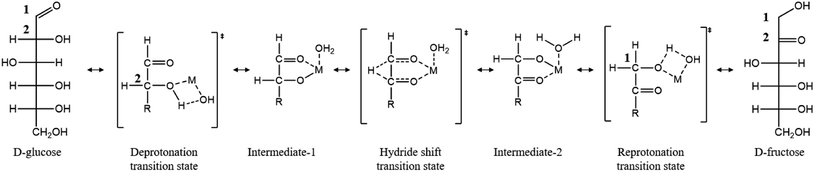 |
| Scheme 1 Proposed mechanism of glucose to fructose isomerization in water. “M” represents a metal center [e.g., Cr, Al, and Sn (in Sn BEA)]. Redrawn from ref. 3. | |
Different approaches have emerged to identify the catalytically active species. Our group combined kinetic rate measurements with a speciation thermodynamic model, called Optimum Logic Inc. Systems' Stream Analyzer software (OLI software, 2017), to suggest [Cr(H2O)5(OH)]2+ is active in CrCl3-catalyzed glucose isomerization.17 Hu and co-workers performed tandem electrospray ionization mass spectrometry (ESI-MS/MS) to propose, due to its abundance, [Al(H2O)4(OH)2]1+ is the active species when AlCl3 is used as a catalyst.9,18 Given the difference in the active species between CrCl3 and AlCl3, the fact that ESI-MS/MS may alter speciation during the measurement,19 and that observed species are often spectators rather than the active ones, the active aluminum species still remain(s) unknown.
In this work, we integrate kinetics with more direct speciation measurements for the first time to provide evidence for the active species of AlCl3. Our judicious choice of AlCl3 is based on the fact that it is a selective catalyst in glucose isomerization and an excellent salt to be followed spectroscopically.20–24 We investigate the interplay between Lewis (AlCl3) and Brønsted (HCl) acids using the OLI software, while simultaneously developing an experimental protocol to quantify the various aluminum species. We use 27Al quantitative nuclear magnetic resonance (qNMR) and pH to measure the aqueous aluminum species and protons, respectively, and combine inductively coupled plasma-mass spectrometry (ICP-MS), dynamic light scattering (DLS), and ultrafiltration to measure the aluminum nanoparticles. Finally, we correlate the glucose isomerization rate with the aluminum species' concentrations to propose an active species.
Methods
Speciation model
AlCl3 speciation was predicted using the mixed-solvent electrolyte OLI software.25,26 The model predicts speciation by solving chemical equilibria of multicomponent systems and combining standard-state thermochemical properties of solution species with an expression for the excess Gibbs free energy. The standard-state properties are calculated using the Helgeson–Kirkham–Flowers (HKF) equation of state for aqueous ions and electrolytes at infinite dilution, while the excess Gibbs free energy model incorporates short, middle, and long-range electrostatic interactions. Further details regarding these calculations can be found in our previous work.8 The OLI model has been parameterized at conditions that differ from those typically encountered in isomerization chemistry and thus, its accuracy is unknown. We address this point in this paper by more direct measurements of aluminum speciation.
pH measurements
pH measurements were obtained using the 3300 High Temperature (HT) PERpH-X sensor (Rosemount) and the 56 Advanced Dual-Input analyzer (Rosemount). A typical measurement was conducted in a 100 mL thick-walled glass vessel (Fisher Scientific) containing catalyst solution. The catalyst solution was stirred and heated for a specified time. The pH sensor was then inserted into the vessel and pH was obtained in situ. Heated samples were then cooled to room temperature and pH was obtained ex situ. The sensor was confirmed to measure the pH of buffer solutions (Fisher Scientific) within their specified temperature range (298 to 373 K, see Fig. S1†).
27Al qNMR spectroscopy
27Al qNMR was carried out on an Avance III 400 MHz NMR spectrometer (Bruker). Spectra were measured at 104.27 MHz (pulse width: 12.50 μs, acquisition time: 0.1966 s, scans: 64, solvent: 90% H2O, 10% D2O) and processed using Mestrelab Research software (mNOVA). The samples were prepared in quartz NMR tubes (NewEra), containing 0.5 mL of reaction mixture and were studied at 303 or 363 K. For quantification purposes, an external standard of Al(H2O)63+ was prepared with 5 mM AlCl3 and 100 mM HCl (Fig. S2†).
Ultrafiltration
Ultrafiltration was performed using Vivaspin 500 concentrators (Sartorius) with a 10
000 molecular weight cut-off (MWCO). The concentrators were filled with 500 μL of either freshly prepared or heat-treated catalyst solutions and placed into the centrifuge (Eppendorf Centrifuge 5424) for 15 minutes at a spin speed of 15
000g. Samples were then recovered from the bottom of the concentrate pocket with a pipette.
ICP-MS
ICP-MS measurements on freshly prepared and heat-treated catalyst solutions were carried out using an Agilent 7500cx Series instrument (Wilmington, DE). Samples underwent ultrafiltration, followed by acid treatment prior to ICP-MS analysis. The aluminum standards (Agilent) were prepared in HNO3 (70 w/w%, Sigma Aldrich) and diluted for the ICP-MS calibration.
DLS
DLS experiments were conducted on a Brookhaven ZETAPALS instrument. DLS measurements were obtained both before and after heating the catalyst solutions. The autocorrelation functions were analyzed using the Multimodal Size Distribution algorithm of the accompanying software, where the viscosity of the solutions was assumed to equal that of water.
Catalytic measurements
All chemicals were purchased from Sigma Aldrich and used as received. The reactions were conducted in 10 mL glass vials (Sigma Aldrich) heated in an aluminum reactor block with controlled stirring (Fisher Scientific). Catalyst solutions of AlCl3·6H2O (Sigma Aldrich) and HCl (37 w/w%, Sigma Aldrich) were stirred and preheated at reaction temperature for 24 h. Kinetic experiments were then carried out in sealed reactor vials containing the preheated catalyst solution (2 mL) and glucose. The Al-to-reactant molar ratio was kept at 9
:
100, which corresponds to 5 mM AlCl3 and 1 wt% glucose solution. The HCl concentration varied from 3 to 44 mM when used with AlCl3 (except for the external standard mentioned above). At different time points, the vials were removed from the oil bath, quenched in ice to stop the reaction, and filtered with a 0.2 μm filter (Fisher Scientific).
Quantification of the liquid products was achieved using high-performance liquid chromatography (HPLC, Waters Alliance Instruments e2695), equipped with a refractive index (RI) detector and a photodiode array (PDA) detector. Sugars were separated using a Biorad HPX87C column at 348 K with HPLC-grade water flowing at 0.5 mL min−1 as the mobile phase. Acid byproducts and HMF were separated using a Biorad HPX87H column heated to 323 K with 5 mM sulfuric acid flowing at 0.5 mL min−1 as the mobile phase.
Results and discussion
Model-predicted AlCl3 speciation in aqueous media
In aqueous media, AlCl3 dissociates to form metal cations. These cations are solvated by water, forming complexes, such as [Al(H2O)6]3+, which can be further hydrolyzed, as shown in eqn (1).15,16,27 |
[Al(H2O)6]3+ + xH2O ↔ [Al(H2O)6−x(OH)x](3−x)+ + xH3O+
| (1) |
The main species predicted using the OLI software vs. HCl concentration include the hexa-aqua species, [Al(H2O)6]3+, the stable, crystalline solid form of aluminum hydroxide, called boehmite, AlO(OH), and the mono- and di-hydroxy species, [Al(H2O)5(OH)]2+ and [Al(H2O)4(OH)2]1+, respectively (Fig. 1 and Scheme 2). The cations are represented as Al3+, AlOH2+, and Al(OH)21+ (for simplicity).
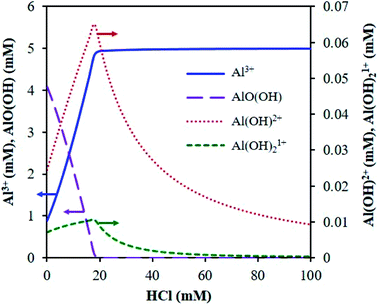 |
| Fig. 1 Distribution of AlCl3 speciation (5 mM AlCl3) at reaction temperature (413 K), calculated using the OLI software. | |
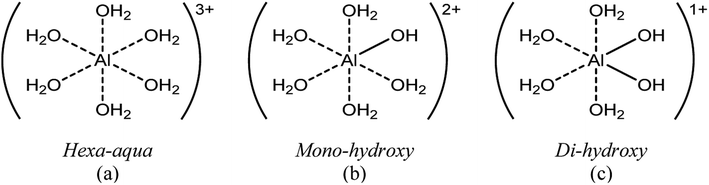 |
| Scheme 2 Al(III) ions generated from the dissolution of AlCl3 in aqueous media. | |
With increasing HCl concentration, the Al3+ concentration increases monotonically, and that of AlO(OH) decreases monotonically, whereas the concentrations of Al(OH)2+ and Al(OH)21+ exhibit volcano-like curves with peaks at intermediate HCl concentrations. At low HCl concentrations, the concentrations of Al(OH)2+ and Al(OH)21+ increase as the pH drops at the expense of the solid. The distribution of aluminum species at high HCl concentrations can be rationalized by Le Chatelier's principle, where an increase in H+ concentration shifts the thermodynamic equilibrium to the left in eqn (1), suppressing the formation of the hydrolyzed aluminum species and increasing the concentration of Al3+. The dominance of Al3+ species at high acid concentrations has been reported before.3,15 In addition, the prediction of solid boehmite is consistent with Hem et al.'s findings, which demonstrated that heating aqueous aluminum results in the formation of boehmite, whose structure was confirmed by X-ray diffraction (XRD).28 To our knowledge, the concentrations of the hydrolyzed aluminum species have not been quantified; however, Mesmer et al. determined the hydrolyzed species are most significant at relatively low concentrations of aluminum (e.g., ≤10 mM),29 as considered here. The model predicts the hydrolyzed species' concentrations increase with an increase in solution temperature, which is expected given that aluminum hydrolysis is endothermic (Fig. S3†).27 Qualitatively, the results remain similar when temperature changes.
Equilibration of AlCl3–HCl catalyst solutions
Next, we investigated the time necessary for the AlCl3–HCl catalyst solutions to reach equilibrium. Equilibration allows formation of the various aluminum species30 and thus proper comparison between the experimentally measured and predicted speciation,26 and ensures quasi-equilibrated speciation during short reaction times to enable kinetic analysis (which otherwise can be influenced by varying speciation during kinetics measurements). Different approaches have been employed in prior work to determine the time necessary for equilibrium. Frink et al. measured the pH of aluminum salt solutions as a function of time and temperature (298 K and 313 K) upon cooling (ex situ).30 Hem et al. performed measurements of the pH together with quantification of polymeric and solid aluminum.28 Since OLI predictions indicate the formation of solid aluminum, we measure pH and solids. The catalyst solutions were preheated at reaction temperature for 24 h, and the pH was obtained in situ and ex situ; not a noticeable difference between in situ and ex situ pH measurements was found (see Fig. 2 at 363 K and Fig. S4† for measurements at 303 K and 413 K). The irreversible pH behavior observed upon cooling is most likely due to the formation of stable solid aluminum species. Thereafter, we present only ex situ pH data. The solid was quantified through ultrafiltration followed by ICP-MS analysis of the permeate. The difference between the initial amount of aluminum, prior to preheating, and the concentration of aluminum in the permeate was taken as the amount of solid.
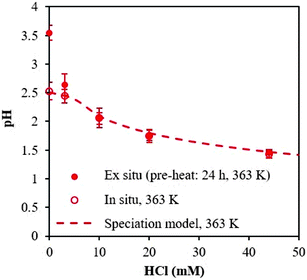 |
| Fig. 2 pH measured ex situ (closed circles) or in situ (open circles) compared to pH calculated from OLI software (line) at 363 K. Samples contained 5 mM AlCl3. Error bars correspond to 95% confidence interval. | |
Fig. 3a shows the ex situ measured pH and Fig. 3b shows the amount of solid as a function of time. The pH initially drops during the first 1 h and then remains approximately unchanged after 8 h, whereas solid formation increases with time and eventually remains constant after 24 h. Fig. 3 indicates multiple time scales whereby solids form slowly compared to equilibration of small species. Hsu proposed the solid results from an intermediate polymeric species,14 which forms within the first hour of heating, and, in combination with the formation of the hydrolyzed species, results in the formation of H+, which decreases the pH. As time increases, the polymeric species undergo nucleation to form stable solid species, such as boehmite.14 This nucleation and/or subsequent growth is slow, given the number of particles continue to increase for 24 h. DLS measurements further indicate the formation of nanoparticles and provide the average particle growth as a function of time (Fig. S5†). The average particle diameter remains constant after 24 h of heating, which is consistent with the time taken for observable solid formation using ultrafiltration/ICP-MS. We observed similar results for samples preheated at 363 K (Fig. S5†). For these samples, solids were present after being heated for 24 h or longer, and their size and amount remained constant thereafter whereas the pH equilibrated faster (Fig. S6†). Therefore, we have decided to preheat our samples for 24 h at reaction temperature for equilibration of aluminum species prior to conducting kinetic experiments.
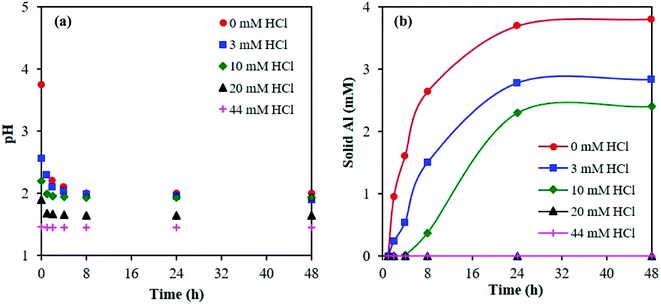 |
| Fig. 3 Determination of equilibrium from (a) pH, measured ex situ, and (b) amount of solid formed with time. Samples contained 5 mM AlCl3 and were cooled from 413 K to 303 K. | |
Direct measurements of AlCl3 speciation
Fig. 4 outlines the methodology to quantify each species. qNMR measurements were performed in situ at 363 K and ex situ at 413 K due to instrument limitations.
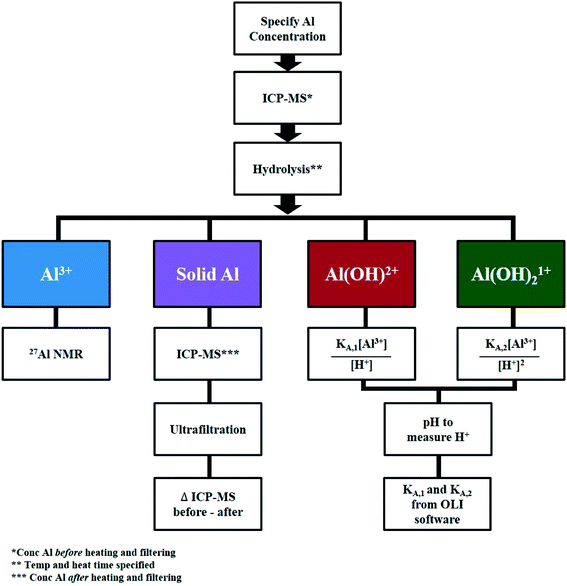 |
| Fig. 4 Methodology to quantify aluminum speciation at specified concentrations of AlCl3 and HCl, temperature, and heating time. | |
Hexa-aqua aluminum (Al3+)
We first quantified the hexa-aqua monomer, Al3+, using 27Al qNMR, at a chemical shift of ∼0 ppm.31 qNMR spectroscopy is non-destructive, quantitative, and uses a low-energy electromagnetic wave, causing an extremely small energy perturbation to the system.32–34 In addition, the quantitative accuracy of NMR analysis has reached three significant figures.32–34 Following a similar approach to that of Maki et al.,32 we measured the concentration of Al3+ by comparing the qNMR peak areas of experimental samples to an external standard, which consisted of 5 mM AlCl3 and 100 mM HCl. The concentration of HCl in the standard is high to ensure all aluminum is in the form of Al3+, according to Fig. 1. Similar to the pH measurements, it did not make a difference if the Al3+ species was quantified in situ (Fig. S7 and Table S1†) or ex situ (Fig. 5 and Table S2†); both measurements were in reasonable agreement with the speciation model (Fig. 6a). We hypothesize the unaccounted aluminum exists primarily as suspended solids, which are not detect by liquid-phase qNMR.15
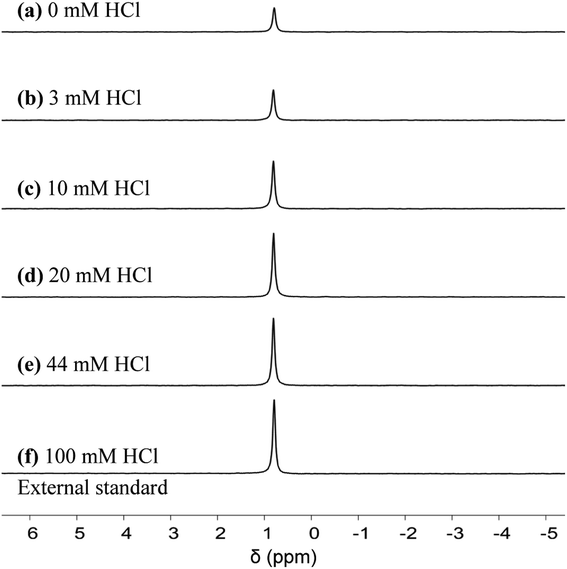 |
| Fig. 5 27Al qNMR spectra for Al3+. Experimental conditions: preheat 413 K for 24 h, 5 mM AlCl3. qNMR measurements obtained upon cooling to 303 K. | |
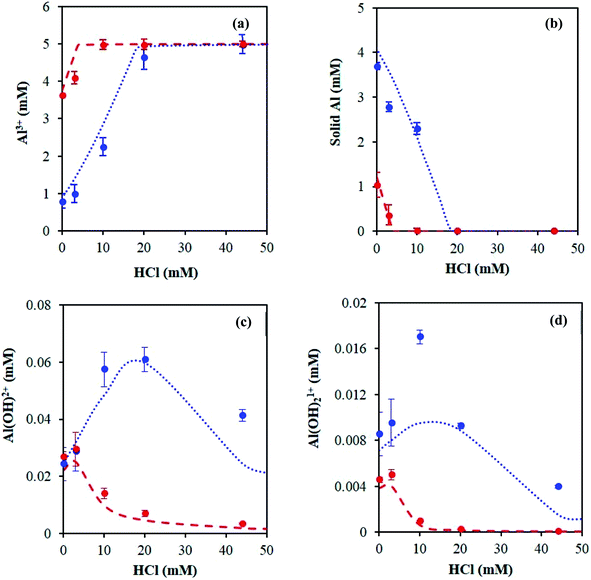 |
| Fig. 6 Aluminum speciation calculated by OLI software at 363 K (red dash lines) and 413 K (blue dotted lines) compared to experimentally inferred species (data represented by red and blue dots). (a) Al3+ (measured in situ at 363 K and ex situ upon cooling from 413 K to 303 K), (b) solid Al (measured ex situ), (c) Al(OH)2+, and (d) Al(OH)21+. Experimental samples contained 5 mM AlCl3 and were preheated at specified temperature for 24 h. | |
Solid aluminum
We next quantified the solid aluminum species. Techniques such as dialysis and ultrafiltration are commonly used to separate fine colloidal mineral aluminum and polymeric aluminum species from soluble aluminum.15 Therefore, we combined ultrafiltration with DLS and ICP-MS measurements to quantify solid aluminum species in our catalyst solutions. DLS measurements detected the presence of aluminum particles and provided the average particle diameter. This allowed for selection of an ultrafiltration membrane with a pore diameter of 5 nm, significantly less than that detected by DLS (Fig. S8†). The samples then underwent heating and ultrafiltration. ICP-MS determined the concentration of aluminum in the permeate. Our experimentally inferred data are consistent with the calculated amount of solid aluminum (Fig. 6b).
Hydrolyzed monomers (AlOH2+ and Al(OH)21+)
The low concentration and rapid proton exchange associated with the hydrolyzed species make them difficult to observe experimentally.27 Therefore, we deduced their concentration through the fundamental equilibrium relation (Tables S3 and S4†). This required the Al3+ and H+ concentrations and the acid dissociation constants (KA,1 and KA,2). The Al3+ and H+ concentrations were obtained by qNMR and pH measurements, respectively. The acid dissociation constants were obtained from the OLI software (Tables S3 and S4†). The experimentally estimated mono- and di-hydrolyzed concentrations exhibit the same volcano-like behavior predicted by the OLI software as a function of HCl concentration, as shown in Fig. 6c and d, respectively.
Glucose conversion and catalytically active species in equilibrated catalyst solution
A series of kinetic experiments with glucose added to preheated catalyst solutions containing AlCl3 and HCl were performed. The initial rates of glucose isomerization were obtained by fitting the glucose concentration profiles at low conversions (≤15%) with a first-order rate expression, where fructose was the main product observed (Fig. S9 and S10†). Fig. 7 shows these rates as a function of HCl concentration.
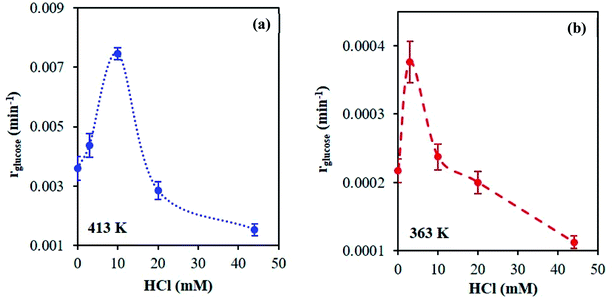 |
| Fig. 7 Effect of HCl on glucose isomerization rate in AlCl3 solutions (5 mM) that have been equilibrated at (a) 413 K and (b) 363 K for 24 h prior to kinetic study. Reaction conditions: glucose 1 wt%, Al to glucose molar ratio 9 : 100. | |
Interestingly, with increasing HCl, the glucose conversion rate exhibits a trend that is qualitatively consistent with the Al(OH)2+ and Al(OH)21+ species' concentrations, shown in Fig. 1 (see Fig. S3† for 363 K data). The slow glucose consumption at low HCl concentrations (before the maximum rate) is congruent with the formation of solid aluminum species, indicating the solid likely hinders glucose conversion. Tang et al. observed, when using solid Al(OH)3 as a catalyst, that Al(OH)3 did not effectively catalyze the reaction, and resulted in low glucose conversion (X = 7.8%) and fructose yield (YFru = 6.5%) compared to AlCl3 (X = 31.8% and YFru = 26.3%) at the same reaction conditions.9 Similarly, the Al3+ species is not likely catalytically active given the reaction rate continues to decrease as the Al3+ species concentration increases. The correlation between the estimated glucose consumption rate and the hydrolyzed species strongly indicates that one or both of these Al(OH)x(3−x)+ species may be catalytically active for glucose isomerization. Since the speciation model26 was developed at conditions different from typical sugar experiments, we used our direct speciation measurements to elucidate the aluminum species.
At 413 K, the linear scaling observed between the glucose isomerization rate and the di-hydrolyzed Al(OH)21+ concentration (Fig. 8) strongly indicates Al(OH)21+ is the catalytically active species. At 363 K, the low reaction rate, extremely small values of the rate constants and low concentrations of the aluminum species (see Table S4 and Fig. S11†) render our data inconclusive regarding the active species.
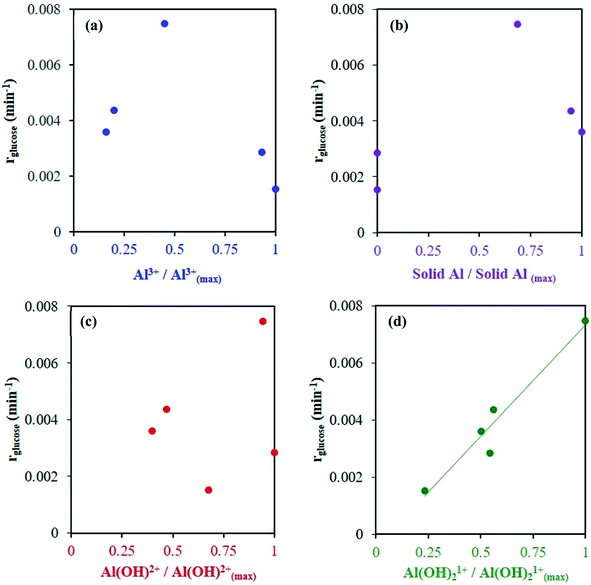 |
| Fig. 8 Glucose conversion as a function of measured Al species' concentrations, normalized to the maximum observed species' concentration (see Table S3† for observed species' concentrations). Catalyst solutions were preheated at 413 K for 24 h prior to kinetic study. Reaction conditions: glucose 1 wt%, Al to glucose molar ratio of 9 : 100, 413 K. | |
Conclusions
We have coupled kinetic studies with direct speciation measurements to elucidate the active species of AlCl3 in glucose to fructose isomerization. Experiments were designed based on insights obtained from modeling the aluminum hydrolysis using the OLI software. We established an experimental protocol to quantify the various aluminum species that employs 27Al qNMR for the hexa-aqua aluminum monomer, ultrafiltration and ICP-MS for the solid, and pH measurements combined with equilibrium relations for the mono- and di-hydroxy aluminum species. We found that speciation reaches equilibrium after heating the catalyst solutions for long times. Linear scaling between the glucose isomerization rate and the speciation measurements at sufficiently high temperatures indicates that the hydrolyzed Al(III) complex [Al(H2O)4(OH)2]1+ is the active species in glucose isomerization. This finding supports the hypothesis of Hu and co-workers, who observed [Al(H2O)4(OH)2]1+ using ESI-MS/MS.9 Furthermore, our findings support the idea that the catalytic species responsible for the isomerization exists as a bifunctional Lewis acidic/Brønsted base site.3 The approach developed here can be applied to study the speciation of other metal halides. For metals not observed by qNMR, such as CrCl3, other spectroscopic techniques, such as ultraviolet visible light spectrometry (UV-Vis), could possibly be employed to study the dominant hexa-aqua species.
Conflicts of interest
There are no conflicts to declare.
Acknowledgements
We acknowledge support from the National Science Foundation under Award No. 1434456. The authors would like to thank Caroline Golt (UD) for performing the ICP-MS measurements, Dr Shi Bai (UD) and Dr Elizabeth McCord (UD) for their assistance with the qNMR method, and John Ruano-Salguero (UD), Prof. Raul Lobo (UD), and Dr Basudeb Saha (UD) for useful discussions.
References
- R. J. van Putten, J. C. van der Waal, E. de Jong, C. B. Rasrendra, H. J. Heeres and J. G. de Vries, Chem. Rev., 2013, 113, 1499–1597 CrossRef CAS PubMed.
- A. Corma, S. Iborra and A. Velty, Chem. Rev., 2007, 107, 2411–2502 CrossRef CAS PubMed.
- V. Choudhary, A. B. Pinar, R. F. Lobo, D. G. Vlachos and S. I. Sandler, ChemSusChem, 2013, 6, 2369–2376 CrossRef CAS PubMed.
- T. D. Fenn, D. Ringe and G. A. Petsko, Biochemistry, 2004, 43, 6464–6474 CrossRef CAS PubMed.
- R. DiCosimo, J. McAuliffe, A. J. Poulose and G. Bohlmann, Chem. Soc. Rev., 2013, 42, 6437–6474 RSC.
- S. H. Bhosale, M. B. Rao and V. V. Deshpande, Microbiol. Rev., 1996, 60, 280–300 CAS.
- M. Moliner, Y. Roman-Leshkov and M. E. Davis, Proc. Natl. Acad. Sci. U. S. A., 2010, 107, 6164–6168 CrossRef CAS PubMed.
- E. Nikolla, Y. Roman-Leshkov, M. Moliner and M. E. Davis, ACS Catal., 2011, 1, 408–410 CrossRef CAS.
- J. Q. Tang, X. W. Guo, L. F. Zhu and C. W. Hu, ACS Catal., 2015, 5, 5097–5103 CrossRef CAS.
- T. D. Swift, H. Nguyen, A. Anderko, V. Nikolakis and D. G. Vlachos, Green Chem., 2015, 17, 4725–4735 RSC.
- Y. J. Pagan-Torres, T. F. Wang, J. M. R. Gallo, B. H. Shanks and J. A. Dumesic, ACS Catal., 2012, 2, 930–934 CrossRef CAS.
- V. Choudhary, S. I. Sandler and D. G. Vlachos, ACS Catal., 2012, 2, 2022–2028 CrossRef CAS.
- R. Bermejo-Deval, R. S. Assary, E. Nikolla, M. Moliner, Y. Roman-Leshkov, S. J. Hwang, A. Palsdottir, D. Silverman, R. F. Lobo, L. A. Curtiss and M. E. Davis, Proc. Natl. Acad. Sci. U. S. A., 2012, 109, 9727–9732 CrossRef CAS PubMed.
- P. H. Hsu, Clays Clay Miner., 1988, 36, 25–30 CAS.
- G. Sposito, The Environmental Chemistry of Aluminum, CRC Press Inc., Boca Raton, FL, 1989 Search PubMed.
- C. F. Baes, The Hydrolysis of Cations, Krieger Publishing Company, Malabar, FL, 1976 Search PubMed.
- V. Choudhary, S. H. Mushrif, C. Ho, A. Anderko, V. Nikolakis, N. S. Marinkovic, A. I. Frenkel, S. I. Sandler and D. G. Vlachos, J. Am. Chem. Soc., 2013, 135, 3997–4006 CrossRef CAS PubMed.
- J. Q. Tang, L. F. Zhu, X. Fu, J. H. Dai, X. W. Guo and C. W. Hu, ACS Catal., 2017, 7, 256–266 CrossRef CAS.
- I. I. Stewart, Spectrochim. Acta, Part B, 1999, 54, 1649–1695 CrossRef.
- P. M. Bertsch, R. I. Barnhisel, G. W. Thomas, W. J. Layton and S. L. Smith, Anal. Chem., 1986, 58, 2583–2585 CrossRef CAS.
- M. A. Curtin, L. R. Ingalls, A. Campbell and M. James-Pederson, J. Chem. Educ., 2008, 85, 291–293 CrossRef CAS.
- B. C. Faust, W. B. Labiosa, K. H. Dai, J. S. Macfall, B. A. Browne, A. A. Ribeiro and D. D. Righter, Geochim. Cosmochim. Acta, 1995, 59, 2651–2661 CrossRef CAS.
- K. Shafran, O. Deschaume and C. C. Perry, Adv. Eng. Mater., 2004, 6, 836–839 CrossRef CAS.
- A. Singhal and K. D. Keefer, J. Mater. Res., 1994, 9, 1973–1983 CrossRef CAS.
- P. M. Wang and A. Anderko, Fluid Phase Equilib., 2001, 186, 103–122 CrossRef CAS.
- P. M. Wang, A. Anderko and R. D. Young, Fluid Phase Equilib., 2002, 203, 141–176 CrossRef CAS.
- C. F. Baes and R. E. Mesmer, Am. J. Sci., 1981, 281, 935–962 CrossRef CAS.
- J. D. Hem, Adv. Chem. Ser., 1968, 98–114 CrossRef CAS.
- R. E. Mesmer and C. F. Baes, Inorg. Chem., 1971, 10, 2290–2296 CrossRef CAS.
- C. R. Frink and M. Peech, Inorg. Chem., 1963, 2, 473–478 CrossRef CAS.
- J. W. Akitt, Multinuclear NMR, Springer, US, New York, 1987 Search PubMed.
- H. Maki, G. Sakata and M. Mizuhata, Analyst, 2017, 142, 1790–1799 RSC.
- F. Malz and H. Jancke, J. Pharm. Biomed. Anal., 2005, 38, 813–823 CrossRef CAS PubMed.
- G. F. Pauli, B. U. Jaki and D. C. Lankin, J. Nat. Prod., 2005, 68, 133–149 CrossRef CAS PubMed.
Footnote |
† Electronic supplementary information (ESI) available. See DOI: 10.1039/c8ra03088j |
|
This journal is © The Royal Society of Chemistry 2018 |
Click here to see how this site uses Cookies. View our privacy policy here.