DOI:
10.1039/C8RA03754J
(Paper)
RSC Adv., 2018,
8, 24231-24235
Highly efficient and recyclable water-soluble fullerene-supported PdCl2 nanocatalyst in Suzuki–Miyaura cross-coupling reaction
Received
2nd May 2018
, Accepted 16th June 2018
First published on 4th July 2018
Abstract
A water-soluble fullerene-supported PdCl2 nanocatalyst [C60-TEGS/PdCl2] was prepared by coordination of water-soluble fullerene nanoparticles with palladium chloride. In pure water, the catalytic activity of nanocatalyst [C60-TEGS/PdCl2] for Suzuki–Miyaura cross-coupling reaction was investigated under different reaction conditions. The results showed that biphenyl compounds could be synthesized in high yields at room temperature using 0.01 mol% of [C60-TEGS/PdCl2] as the catalyst and K2CO3 as the base with the reaction time of 4 h. The catalyst was recycled five times, and the yield clearly did not decrease.
Introduction
The Suzuki–Miyaura cross-coupling reaction for aryl–aryl bond construction has been one of the most useful and convenient pathways to synthesize biphenyl complexes.1–6 It is applied for organic functional materials and extensively employed in the synthesis of herbicides, pharmaceuticals, and natural products as biaryl motifs.7–11 The catalyst used in the Suzuki–Miyaura cross-coupling reaction is vital for this reaction, and the search for a suitable catalyst is the latest and most challenging research area. Although nickel,12,13 copper,14,15 and other transition metal compounds catalyze the Suzuki–Miyaura cross-coupling reaction, palladium catalysts are employed widely. As is well-known, the rapid increase in studies about the phosphine ligand/palladium catalyst for this reaction is primarily due to its excellent donor capability and stability effect; however, its shortcomings, such as high toxicity and air- and moisture-sensitivities seriously restrict its actual applications. Therefore, phosphine-free ligands have become the focus of scientific researchers.
In recent years, many reports have shown that phosphine-free ligands of palladium are capable of activating aryl halide with phenylboronic acid for the Suzuki–Miyaura cross-coupling reaction. Among these, phosphine-free ligands, N-phenylurea,16 N-heterocyclic carbine,17 isoxazol-3-amine18 and other bis(thiourea) species19 exhibit high catalytic activities and stabilities. However, some problems such as lack of coordination flexibility, difficulty in synthesis, use of toxic reagent, and relatively harsh experimental conditions still need to be solved.
To resolve the above-mentioned challenges, it was necessary to find a new type of catalyst. Compared to many of the mentioned catalysts, supported catalysts used in Suzuki–Miyaura cross-coupling reaction with good coordination ability, easy synthesis, cost-effectiveness, and high thermal stability have become the core research focus of scientific researchers.20–25 A number of studies have been reported for the immobilization of palladium nanocatalysts on support materials such as polyvinyl pyrrolidone26 (PVP), amphiphilic resin,27 N,N-dihexylcarbodiimide,28 silica gel,29 and PEG-2000 (ref. 30–33) and by using them, high yield can mostly be obtained. It has been verified that coordination bonding catalytic active substances with high surface energies and high surface-to-volume ratios can improve supported catalysts' efficiency.34,35 However, there are still some problems with the supported catalyst. For example, the recyclability of PdCl2 immobilized on polyacrylamide was limited because PdII is leached, leaving only the base polymer on the surface after six runs.36
In this study, a highly efficient and recyclable water-soluble fullerene-supported PdCl2 nanocatalyst [C60-TEGs/PdCl2] was prepared by coordination of water-soluble fullerene nanoparticles with palladium chloride. The catalytic system of [C60-TEGs/PdCl2] exhibited high activity in the Suzuki–Miyaura cross-coupling reaction of aryl bromides with aryl boronic acids at room temperature in deionized water under ambient atmosphere. In this reaction, deionized water was used as a green solvent, which was in accordance with the concept of “green chemistry”. Furthermore, the [C60-TEGs/PdCl2] catalyst could be readily recovered and reused up to five consecutive runs without significant loss in its catalytic activity. As a new type of Pd nanocatalyst, the [C60-TEGs/PdCl2] catalyst was stable enough, and only small amounts of Pd were leached in a long-term static state.
Results and discussion
Characterization of [C60-TEGs/PdCl2]
At room temperature, C60-TEGs and C60-TEGs/PdCl2 aqueous solutions were uniformly dispersed by ultrasonication and filtered with 0.45 μm filter membranes. Their particle sizes and distributions were measured at a wavelength of 633 nm by dynamic light scattering (DLS). The average particle sizes of C60-TEGs and C60-TEGs/PdCl2 were 42.5 nm and 61.5 nm, respectively. The TEM image of water-soluble fullerene-supported PdCl2 nanocatalyst [C60-TEGs/PdCl2] is shown in Fig. 1. The catalyst is spherical, and the particle size is from 30 to 90 nm. Compared with C60-TEGs, [C60-TEGs/PdCl2] has a larger average diameter. These results indicate changes in the particle sizes or distributions of the two samples; the palladium ion within the pentacyclic structure plays a significant role in determining the particle size.
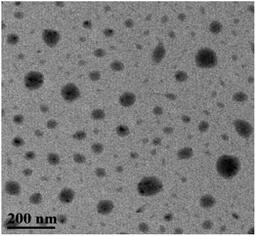 |
| Fig. 1 TEM image of water-soluble fullerene-supported PdCl2 nanocatalyst [C60-TEGs/PdCl2]. | |
The different components of Pd 3d photoelectron peaks of nanocatalyst [C60-TEGs/PdCl2] are shown in Fig. 2. The peaks at 337.02 and 342.22 eV for different chemical environments of palladium confirmed the oxidation state of Pd2+. PdCl2 showed pi-interactions with C60 double bonds, and the charge transfer occurred from Pd atoms to the interstitial C atoms of C60, which led to the decreasing electron density of Pd3d.37 However, the binding energy of Pd2+ did not change. From the above-mentioned results, it can be seen that PdCl2 is co-ordinated to TEG to form the water-soluble fullerene-supported PdCl2 nanocatalyst.
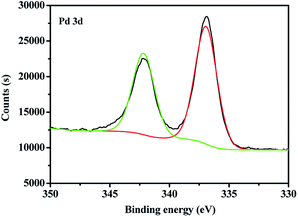 |
| Fig. 2 Pd 3d level spectra of [C60-TEGs/PdCl2]. | |
The Pd content of the catalyst was determined using inductively coupled plasma atomic emission spectroscopy (ICP-AES). Table 1 shows the quantities of PdCl2 and C60-TEGs employed in each reaction. The coordination loading rate did not sharply increase as the amount of C60-TEGs increased. Therefore, when the mass ratio of C60-TEGs and PdCl2 was 1
:
1, the optimum value was obtained.
Table 1 Loading rate of PdCl2 on C60-TEGs
mC60-TEGs : mPdCl2 (mg) |
Loading rate (%) |
10 : 20 |
83.2 |
10 : 10 |
87.3 |
15 : 10 |
87.3 |
Catalytic studies
To examine the catalytic activity of the water-soluble fullerene-supported PdCl2 nanocatalyst [C60-TEGs/PdCl2], Suzuki–Miyaura cross-coupling reaction of 4-bromoacetophenone with phenylboronic acid was chosen as a model reaction. The initial investigations of 4-bromoacetophenone with phenylboronic acid catalyzed by [C60-TEGs/PdCl2] were conducted with various conditions such as the amount of the nanocatalyst [C60-TEGs/PdCl2], reaction time, and different bases. As shown in Table 2, the effect of the amount of the nanocatalyst [C60-TEGs/PdCl2] on the coupling reaction was studied. We found that lowering the amount of [C60-TEGs/PdCl2] from 0.1 mol% to 0.01 mol% did not affect the reaction performance for 2 h (entries 1–4, Table 2). When the amount of [C60-TEGs/PdCl2] decreased to 0.005 mol%, the reaction yield showed a sharp decline (entry 5, Table 2). The reaction time was also investigated, and it was found that it had a significant effect on the coupling reaction. After increasing the reaction time from 1 h to 4 h (entries 4, 6–8, Table 2), the yield improved remarkably. When the reaction time was prolonged to 6 h, the yield barely increased (entry 9, Table 2), because the substrate was completely converted to the product in 4 h. Furthermore, an investigation of the influence of the base suggested that the common and inexpensive inorganic bases can be used. It was found that K2CO3 was the best base for a high yield, whereas other bases such as Na2CO3, NaHCO3, KF, and K3PO4 gave slightly lower yields (entries 10–13, Table 1). Therefore, 0.01 mol% of the catalyst, 4 h of reaction time and 2.0 mmol of K2CO3 as the base were considered to be the most effective conditions.
Table 2 Optimization of reaction conditions at room temperaturea

|
Entry |
Catalyst (mol%) |
Base |
Time (h) |
Yieldb (%) |
Reaction conditions: 1.0 mmol of 4-bromoacetophenones, 1.2 mmol of phenylboronic acid, 2.0 mmol of base, [C60-TEGs/PdCl2] nanocatalyst, 4 mL H2O. Isolated yields. Reaction conditions: 1.0 mmol of 4-bromoacetophenones, 1.2 mmol of phenylboronic acid, 2.0 mmol of base, PdCl2, 4 mL H2O. Reaction conditions: 1.0 mmol of 4-bromoacetophenones, 1.2 mmol of phenylboronic acid, 2.0 mmol of base, physical mixture of PdCl2 and C60-TEGS, 4 mL H2O. |
1 |
0.1 |
K2CO3 |
2 |
98 |
2 |
0.05 |
K2CO3 |
2 |
98 |
3 |
0.025 |
K2CO3 |
2 |
96 |
4 |
0.01 |
K2CO3 |
2 |
95 |
5 |
0.005 |
K2CO3 |
2 |
86 |
6 |
0.01 |
K2CO3 |
1 |
91 |
7 |
0.01 |
K2CO3 |
3 |
96 |
8 |
0.01 |
K2CO3 |
4 |
98 |
9 |
0.01 |
K2CO3 |
6 |
98 |
10 |
0.01 |
Na2CO3 |
4 |
93 |
11 |
0.01 |
NaHCO3 |
4 |
90 |
12 |
0.01 |
KF |
4 |
89 |
13 |
0.01 |
K3PO4 |
4 |
96 |
14c |
0.01 |
K2CO3 |
4 |
18 |
15d |
0.01 |
K2CO3 |
4 |
56 |
For comparison, the cross-coupling reaction of 4-bromoacetophenone and phenylboronic acid was also performed using PdCl2 alone and the physical mixture of PdCl2 and C60-TEGS under the above-mentioned effective conditions. Using PdCl2 alone, only 18% yield of product was produced in the presence of 0.01 mol% of PdCl2 (entry 14, Table 2). However, in the presence of the physical mixture of PdCl2 and C60-TEGS, the yield of the product significantly increased to 56% (entry 15, Table 2). At identical conditions, the [C60-TEGs/PdCl2] nanocatalyst exhibited the highest catalytic activity. It was found that the reactivity and stability of the metal catalysts increased by the use of increasing amounts of efficacious supporting ligands.
Recycling of catalyst
The recycling of water-soluble fullerene-supported PdCl2 nanocatalyst [C60-TEGs/PdCl2] is one of the crucial advantages of this study, which is a vital requirement for any practical application. The model reaction was conducted under the optimized conditions. After completion of the reaction, the catalyst was extracted two times with ether (10 mL). The organic phase was concentrated under reduced pressure, and the aqueous phase was recycled directly for the next run. After verification, it could be found that the [C60-TEGs/PdCl2] catalyst could be readily recovered and reused up to five consecutive runs without significant loss in its catalytic activity; the isolated yield was 92% (Fig. 3). The [C60-TEGs/PdCl2] catalyst was also quantitatively analysed by ICP-AES. The analysis showed that after five recycling runs of the catalyst, only 10 wt% of Pd was leached.
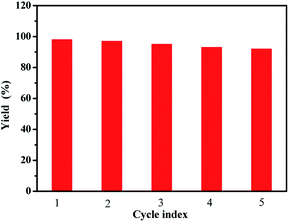 |
| Fig. 3 Recycling of [C60-TEGs/PdCl2] catalyst for the Suzuki–Miyaura coupling reaction under similar conditions. | |
Substrate expansion experiment
Encouraged by the results obtained from the coupling reaction of 4-bromoacetophenone with phenylboronic acid in the presence of [C60-TEGs/PdCl2], the scope and generality of the protocol were then expanded to various aryl halides including a range of more challenging aryl chlorides (Table 3). We tested the scope of nanocatalyst [C60-TEGs/PdCl2] in the Suzuki–Miyaura cross-coupling reaction using different para-, meta- and ortho-substituted aryl halides at low catalyst concentrations with optimal reaction conditions, as demonstrated above. When phenylboronic acid was coupled with aryl bromides containing both electron-donating and electro-withdrawing groups, the corresponding products were obtained in excellent yields (entries 1–9, Table 3). Due to the retarding effect in both the oxidative addition and transmetalation processes, the aryl halides or aryl boronic species with ortho-methyl substituents exhibited low activities. However, high yields could be achieved with prolonged reaction times (entries 7, 9 and 17, Table 3). From the results, it can be seen that some heteroaryl species were also efficiently coupled to provide the corresponding biaryl products in excellent isolated yields (entries 10 and 11, Table 3). The effect of various para-substituents on arylboronic acids toward the cross-coupling was examined (entries 12–17, Table 3). No significant difference was observed in the yields or the reaction times. 4-Chloroacetophenone was coupled with 0.5 mol% [C60-TEGs/PdCl2] to obtain 65% yield after 8 h (entry 18, Table 3). When the more challenging 4-chlorotoluene was used, a lower yield was obtained (entry 19, Table 3).
Table 3 Suzuki–Miyaura cross-coupling reaction of aryl halides with arylboronic acid catalyzed by [C60-TEGs/PdCl2] catalyst under aerobic conditionsa

|
Entry |
Aryl halide |
Aryl boronic |
Time (h) |
Yield (%)b |
Reaction conditions: aryl halide (1.0 mmol), arylboronic acid (1.2 mmol), K2CO3 (2.0 mmol), 0.01 mol% [C60-TEGs/PdCl2], H2O (4 mL), room temperature. Isolated yields. Reaction conditions: aryl chloride (1.0 mmol), phenylboronic acid (1.2 mmol), K2CO3 (2.0 mmol), 0.5 mol% [C60-TEGs/PdCl2], 4 mL EtOH/H2O (V/V, 1 : 1), 80 °C. |
1 |
 |
 |
4 |
96 |
2 |
 |
 |
4 |
95 |
3 |
 |
 |
4 |
98 |
4 |
 |
 |
4 |
97 |
5 |
 |
 |
4 |
95 |
6 |
 |
 |
4 |
98 |
7 |
 |
 |
6 |
87 |
8 |
 |
 |
6 |
91 |
9 |
 |
 |
6 |
76 |
10 |
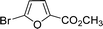 |
 |
6 |
90 |
11 |
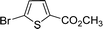 |
 |
6 |
92 |
12 |
 |
 |
4 |
97 |
13 |
 |
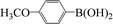 |
4 |
99 |
14 |
 |
 |
4 |
96 |
15 |
 |
 |
4 |
98 |
16 |
 |
 |
4 |
97 |
17 |
 |
 |
6 |
84 |
18c |
 |
 |
8 |
65 |
19c |
 |
 |
8 |
30 |
Experimental
Materials and methods
C60 fullerene, tetraethylene glycol (TEG), palladium chloride, phenylboronic acid, aryl halides and lithium hydroxide were purchased from Energy chemicals and were used without further purification. Other commercially available reagents were purchased from commercial resources and used without further purification.
The Pd content of the [C60-TEGs/PdCl2] catalyst was determined using inductively coupled plasma atomic emission spectroscopy (ICP-AES, Shimadzu ICPE-9000). The size of the nanoparticles of [C60-TEGs/PdCl2] was measured with dynamic laser scattering (DLS, Malvern, Nano ZS90). The morphological examination of [C60-TEGs/PdCl2] was performed by transmission electron microscopy (TEM, JEOL-2100F). The different components of Pd 3d photoelectron peaks of nanocatalyst [C60-TEGs/PdCl2] were determined by X-ray photoelectron spectroscopy (XPS, Kratos AXIS Ultra). Proton nuclear magnetic resonance (NMR) spectroscopy was performed using a Varian 400 MHz spectrometer at room temperature with CDCl3 as a solvent. Chemical shifts for 1H NMR and 13C NMR spectra were given in parts per million from the peak for internal tetramethylsilane.
Preparation of water-soluble fullerene-supported PdCl2 nanocatalyst [C60-TEGs/PdCl2]
Water-soluble fullerene nanoparticles of C60-TEGs were synthesized according to previously reported methods.38–40 The water-soluble fullerene-supported PdCl2 nanocatalyst [C60-TEGs/PdCl2] was prepared by coordination of C60-TEGs with palladium chloride (Scheme 1). Briefly, C60-TEGs were dissolved in 10 mL deionized water; PdCl2 was added, and the mixture was stirred for 20 h. The precipitate was removed by centrifugation. Next, the solution was purified by dialysis against deionized water using dialysis tubing with a molecular weight cut-off of 3.5 kDa for 48 h to remove unbonded PdCl2 and then lyophilized to obtain [C60-TEGs/PdCl2] powders. The powders were dissolved again in deionized water before being used.
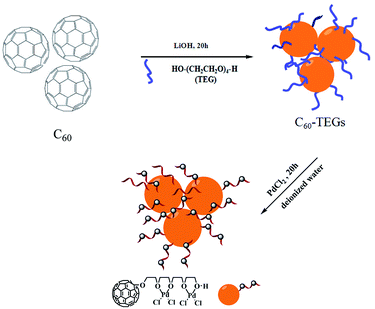 |
| Scheme 1 The synthesis of water-soluble fullerene-supported PdCl2 nanocatalyst [C60-TEGs/PdCl2]. | |
Suzuki–Miyaura cross-coupling reaction catalyzed by [C60-TEGs/PdCl2]
A 25 mL flask was charged with aryl halide (1.0 mmol), aryl boronic acid (1.2 mmol), K2CO3 (2.0 mmol), catalyst (0.01 mol% Pd), and deionized water (4 mL). The reaction mixture was stirred at room temperature under air for a certain time. The progress of the reaction was monitored by thin layer chromatography (TLC). After completion of the reaction, it was extracted with ether (10 mL). The organic phase was concentrated under reduced pressure and chromatography over silica gel using petroleum ether/ethyl acetate (V/V, 10
:
1) to get the desired coupling products. All the purified products were known compounds and were characterized by comparing their 1H and 13C NMR spectra with those found in literature.41
Conclusions
In conclusion, the novel water-soluble fullerene-supported PdCl2 nanocatalyst [C60-TEGs/PdCl2] was synthesized. The catalytic system of [C60-TEGs/PdCl2] provided a facile and aerial condition for the Suzuki–Miyaura cross coupling reaction of aryl bromides with aryl boronic acids in deionized water with excellent yields of desired products. It is important that [C60-TEGs/PdCl2] could be readily recovered and reused up to five consecutive runs by simple extraction without significant loss in its catalytic activity. In additon, the catalytic system of [C60-TEGs/PdCl2] showed great tolerance for a broad range of functional groups on the aryl bromides or chlorides. The high catalytic activity, broad substrate scope, reusability and environmental protection of [C60-TEGs/PdCl2] make it a desirable compound from the environmental and industrial viewpoints.
Conflicts of interest
There are no conflicts to declare.
Acknowledgements
This work was supported by the National Natural Science Foundation of China (No. 21764016 and No. 21664014), the Natural Science Foundation of Jiangxi Province (No. 20161BAB215197) and the Foundation of Jiangxi Provincial Education Department (No. GJJ151031 and GJJ170901).
Notes and references
- J. Almond-Thynne, D. C. Blakemore, D. C. Pryde and A. C. Spivey, Chem. Sci., 2017, 8, 40 RSC
. - B. Tahmasbi and A. Ghorbani-Choghamarani, Catal. Lett., 2017, 147, 649 CrossRef
. - L. M. Kumar and B. R. Bhat, J. Organomet. Chem., 2017, 827, 41 CrossRef
. - F. R. Fortea-Pérez, M. Julve, E. V. Dikarev, A. S. Filatov and S. E. Stiriba, Inorg. Chim. Acta, 2018, 471, 788 CrossRef
. - T. B. Halima, W. Zhang, I. Yalaoui, X. Hong, Y. F. Yang, K. N. Houk and S. G. Newman, J. Am. Chem. Soc., 2017, 139, 1311 CrossRef PubMed
. - M. O. Sydnes, Catalysts, 2017, 7, 35 CrossRef
. - M. R. Netherton and G. C. Fu, Org. Lett., 2001, 3, 4295 CrossRef PubMed
. - J. P. Stambuli, R. Kuwano and J. F. Hartwig, Angew. Chem., Int. Ed., 2002, 41, 4746 CrossRef PubMed
. - P. J. Hajduk, M. Bures, J. Praestgaard and S. W. Fesik, J. Med. Chem., 2000, 43, 3443 CrossRef PubMed
. - V. Polshettiwar, A. Decottignies, C. Len and A. Fihri, ChemSusChem, 2010, 3, 502 CrossRef PubMed
. - P. Huo, J. B. Li, W. Y. Liu, X. H. He and G. Q. Mei, Chem. Lett., 2016, 45, 454 CrossRef
. - M. Tobisu, T. Shimasaki and N. Chatani, Angew. Chem., Int. Ed., 2008, 47, 4866 CrossRef PubMed
. - T. Zell and U. Radius, Z. Anorg. Allg. Chem., 2011, 637, 1858 CrossRef
. - J. H. Li and D. P. Wang, Eur. J. Org. Chem., 2006, 1, 2063 CrossRef
. - K. Hojoh, Y. Shido, H. Ohmiya and M. Sawamura, Angew. Chem., Int. Ed., 2014, 53, 4954 CrossRef PubMed
. - X. Cui, Y. Zhou, N. Wang, L. Liu and Q. X. Guo, Tetrahedron Lett., 2007, 48, 163 CrossRef
. - R. Garrido, P. S. Hernández-Montes, Á. Gordillo, P. Gómez-Sal, C. López-Mardomingo and E. de Jesús, Organometallics, 2015, 34, 1855 CrossRef
. - N. Bumagin, V. Potkin, S. Petkevich, A. Lyakhov, D. Rudakov, M. Livantsov and N. Golantsov, Synthesis, 2011, 44, 151 Search PubMed
. - W. Chen, R. Li, B. Han, B. J. Li, Y. C. Chen, Y. Wu, L. S. Ding and D. Yang, Eur. J. Org. Chem., 2006, 1, 1177 CrossRef
. - X. Jiang, J. Sclafani, K. Prasad, O. Repič and T. J. Blacklock, Org. Process Res. Dev., 2007, 11, 769 CrossRef
. - A. Modak, J. Mondal, M. Sasidharan and A. Bhaumik, Green Chem., 2011, 13, 1317 RSC
. - S. Y. Ding, J. Gao, Q. Wang, Y. Zhang, W. G. Song, C. Y. Su and W. Wang, J. Am. Chem. Soc., 2011, 133, 19816 CrossRef PubMed
. - Y. Wang, J. Zhang, W. Zhang and M. Zhang, J. Org. Chem., 2009, 74, 1923 CrossRef PubMed
. - N. Mejías, R. Pleixats, A. Shafir, M. Medio-Simón and G. Asensio, Eur. J. Org. Chem., 2010, 26, 5090 CrossRef
. - J. D. Patil, S. N. Korade, S. A. Patil, D. S. Gaikwada and D. M. Pore, RSC Adv., 2015, 5, 79061 RSC
. - Y. Li, X. M. Hong, D. M. Collard and M. A. El-Sayed, Org. Lett., 2000, 2, 2385 CrossRef PubMed
. - Y. Uozumi and Y. Nakai, Org. Lett., 2002, 4, 2997 CrossRef PubMed
. - Y. Liu, C. Khemtong and J. Hu, Chem. Commun., 2004, 398 RSC
. - L. Wan and C. Cai, Catal. Commun., 2012, 24, 105 CrossRef
. - T. M. Razler, Y. Hsiao, F. Qian, R. Fu, R. K. Khan and W. Doubleday, J. Org. Chem., 2008, 74, 1381 CrossRef PubMed
. - L. Wu, B. L. Li, Y. Y. Huang, H. F. Zhou, Y. M. He and Q. H. Fan, Org. Lett., 2006, 8, 3605 CrossRef PubMed
. - P. Zhang, Z. Weng, J. Guo and C. Wang, Chem. Mater., 2011, 23, 5243 CrossRef
. - L. Wu, Z. W. Li, F. Zhang, Y. M. He and Q. H. Fan, Adv. Synth. Catal., 2008, 350, 846 CrossRef
. - Z. Chen, Y. Liang, D. S. Jia, Z. M. Cui and W. G. Song, Chin. J. Catal., 2017, 38, 651 CrossRef
. - N. N. Gu, Y. S. Liu, P. Liu, X. W. Ma and Y. Liu, Synth. Commun., 2016, 46, 154 CrossRef
. - G. Alvarenga, C. P. Ruas, J. R. M. Vicenti, F. A. Duarte, M. A. Gelesky and G. R. Rosa, J. Braz. Chem. Soc., 2016, 27, 787 Search PubMed
. - J. Goclon, K. Winkler and J. T. Margraf, RSC Adv., 2017, 7, 2202 RSC
. - J. Jeong, J. Jung, M. Choi, J. W. Kim, S. J. Chung, S. Lim, H. Lee and B. H. Chung, Adv. Mater., 2012, 24, 1999 CrossRef PubMed
. - W. Y. Liu, J. C. Wei and Y. W. Chen, New J. Chem., 2014, 38, 6223 RSC
. - W. Y. Liu, J. C. Wei, Y. W. Chen, P. Huo and Y. Wei, ACS Appl. Mater. Interfaces, 2013, 5, 680 CrossRef PubMed
. - P. Huo, J. B. Li, W. Y. Liu and G. Q. Mei, Chin. J. Catal., 2017, 35, 363–367 Search PubMed
.
|
This journal is © The Royal Society of Chemistry 2018 |
Click here to see how this site uses Cookies. View our privacy policy here.