DOI:
10.1039/C8RA04383C
(Paper)
RSC Adv., 2018,
8, 25008-25013
Fabrication of a scratch & heat resistant superhydrophobic SiO2 surface with self-cleaning and semi-transparent performance
Received
23rd May 2018
, Accepted 25th June 2018
First published on 11th July 2018
Abstract
Herein, we report the fabrication of a superhydrophobic surface with a new and effective silica nanocomposite. A facile synthesis was developed by spraying the as-prepared silica suspension on a glass substrate, where the SiO2 nanoparticles were composed of methylated aerogel particles wrapped by hydroxyl-terminated polydimethylsiloxane (PDMS). Three types of methylated silica aerogel nanoparticles with different surface roughness and porosities were prepared using specific precursors and methylation agents. The coating of the silica aerogels (sodium silicate and trimethylchlorosilane) wrapped in PDMS was exceptionally superhydrophobic with a superior water contact angle of 169.80 ± 3° and a sliding angle of less than 4°. The semi-transparent coating maintained its excellent water repellency at 350 °C for least 4 h and exhibited durable superhydrophobic properties for 6 months at ambient conditions. Additionally, the coating also showed good mechanical stability and remarkable self-cleaning behaviour.
1. Introduction
Superhydrophobic surfaces, with a water contact angle greater than 150° and a sliding angle (the difference between the advancing and receding contact angles) of less than 10°,1 have received increasing attention from both academic and practical fields such as self-cleaning,2,3 anti-corrosion,4,5 oil-water separation,6,7 and anti-icing.8,9 The prevalent fabrication approaches for superhydrophobic surfaces include etching,10 physical/chemical vapour deposition,11,12 electro-spinning,13 nano/microparticle assembly,14,15 the template method,16 magnetron sputtering17 and hot-pressing.18 However, problems remain for most of these methods such as high-energy consumption and the need for expensive and sophisticated instruments. Additionally, expensive poisonous agents (fluorinated components) were used to achieve superhydrophobic surfaces in many studies.19–21 Compared to fluorosilanes, PDMS not only has low surface energy but is also less toxic, cheaper, thermally stable and durable than many polymers, suggesting that PDMS may serve as both a structural precursor and a raw modifying material.22,23 Due to its low intrinsic surface energy and moldability, many kinds of nanomaterials and polymers such as ZnO,24 carbon nanowalls25 and epoxy resin26 were doped with PDMS. Due to suitable preparation process, controllable particle size and good reactivity with organic groups, SiO2 nanoparticles are the most widely applied materials in superhydrophobic surfaces.27,28 Because the aggregation of nanoparticles results in multi-scale roughness and PDMS provides good hydrophobicity with non-polar –CH3 groups, the composites containing SiO2 nanoparticles and PDMS have attracted much attention in recent decades. Few papers investigating the thermal and mechanical stabilities of durable superhydrophobic coatings on glass have been reported, which constraints wide applications of superhydrophobic coating.
Herein, silica aerogel/PDMS composites were synthesized using a typical sol–gel method and subsequent PDMS chemical modification. Interface adhesive (Qsil 216) was used to enhance the adhesion between the superhydrophobic coating and the glass substrate. The preparation was simple and scalable by spraying Qsil 216 and silica aerogel/PDMS composites in order. The semi-transparent superhydrophobic coating was found to be self-cleaning and durable with excellent thermal and mechanical stabilities.
2. Results and discussion
2.1 Surface morphology and chemical composition
Fig. 1 shows a schematic illustration of the superhydrophobic coating. MSA possessed great quantities of non-polar groups (–CH3), but it was difficult to form pure MSA coating on the glass base due to the weak cross-linking interaction. It has been recognized that PDMS with high viscosity and low surface energy has good connectivity for its end hydroxyl groups and long polymer chains, which are flexible and rotatable. Thus, P-MSA exhibited stable and controllable morphology and overcame the fragile features of MSA.
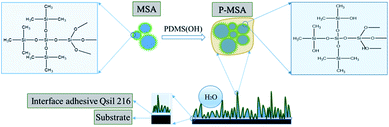 |
| Fig. 1 Schematic of the chemical composition of MSA and P-MSA and the micromorphology of the superhydrophobic coating. | |
Fig. 2 shows the presence of rough micromorphology. Comparing Fig. 2(a), (d) and (g) with Fig. 2(b), (e) and (h), respectively, it was found that the aerogel particles modified by PDMS stacked more intensely and irregularly, implying that the coatings possessed better film-formation properties than before. As seen in Fig. 3(a), (d), and (g), whether or not TMCS or HTMS was added, the particles aggregated more seriously and even became indistinguishable due to the presence of a silane coupling agent. As shown in Fig. 2(b), (e) and (h), the P-MSA-II sample exhibited the most serious aggregation and the smallest porosity. It was speculated that the residual hydroxyl groups on the surface of MSA-II were more than those on MSA-I and MSA-III because of the vigorous hydrolysis of TMCS.
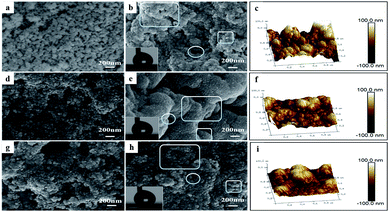 |
| Fig. 2 SEM and AFM images of (a) MSA-I, (b) and (c) P-MSA-I, (d) MSA-II, (e) and (f) P-MSA-II, (g) MSA-III, and (h) and (i) P-MSA-III coatings. | |
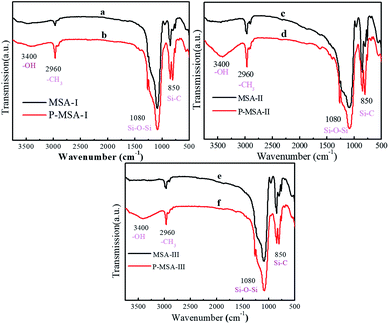 |
| Fig. 3 FTIR spectra of (a) MSA-I, (b) P-MSA-I, (c) MSA-II, (d) P-MSA-II, (e) MSA-III, and (f) P-MSA-III coatings. | |
As we know, the hierarchical micro/nanoscale structure on the surface is essential for superhydrophobic coatings besides chemical composition with low surface energy.29 This was achieved by pores, clusters of silica aerogel bulks and silica aerogel particles, which are marked in Fig. 2(b), (d) and (e), respectively. The globular entities and pores were less than 300 nm in diameter, whereas the sizes of the clusters of silica aerogel bulk were approximately more than 1 μm in the micron level. Notably, globular entities were coarse and composed of small mastoid nanoparticles. The three-dimensional micromorphologies of P-MSA-I, P-MSA-II and P-MSA-III coatings obtained using AFM are shown in Fig. 2(c), (f) and (i), respectively, and these results confirmed the existence of a hierarchical micro/nanoscale structure, corresponding to previous analysis results based on SEM images.
FTIR spectra showed the functional groups of different aerogels before and after PDMS modification (Fig. 3). The peak at 2960 cm−1 was related to the –CH3 groups, and the broad band centered at 3400 cm−1 was ascribed to O–H stretching in the Si–OH groups. The bands located at 1103, 953, and 801 cm−1 were associated with the Si–O–Si asymmetric bond stretching vibration, the Si–OH stretching vibration, and the network Si–O–Si symmetric bond stretching vibration, respectively.16,30 It was clearly observed that many hydroxyl groups remained in three nanocomposites due to the modification of PDMS. Additionally, the intensity of the peaks corresponding to the methyl group increased. PDMS, which was loaded with many methyl groups, provided good hydrophobic properties. Most importantly, PDMS provided many abundant hydroxyl groups due to which the coatings possessed good adhesion with substrates such as glass and paper.
2.2 Static and dynamic wetting properties of three coatings
As shown in the lower-left corner of Fig. 2(b), (e), and (h), it is clear that the water droplets adopted a perfect ball shape, which implied excellent superhydrophobicities of the corresponding coatings. Furthermore, the hydrophobicities of the three P-MSA coatings are quantitatively shown in Table 1 including advancing angle, receding angle and contact angle. The water contact angles of the three samples were higher than 150°. The results were consistent with the droplet shapes in Fig. 2(b), (e), and (h). It was speculated that the superhydrophobic property resulted from the synergistic effect of MSAs and PDMS. On the one hand, nanoparticles tended to agglomerate due to high surface area and surface energy. The inter-particle forces within the agglomerates originated from van der Waals, capillary, and electrostatic forces.31 MSAs, made up of aggregated nanoparticles, provided a multi-scale hierarchical structure with granular bulges and pores, which could trap sufficient air pockets on the rough interstices between liquid and solid.16 Therefore, the water drop could remain on the layer of air with minimum solid fraction in contact. On the other hand, the guest phase (MSAs) was uniformly and completely coated by the host matrix (PDMS) (Fig. 2), which gave rise to the enhancement of hydrophobic methyl groups (Fig. 3). The contact angle hysteresis (θCAH = θAdv − θRec) was less than 10° among the three samples and thus, the droplets could easily slide away from the coating. This resulted in the non-continuous solid/liquid/gas three-phase contact line. The spherical droplets on the surface of the superhydrophobic coatings showed Cassie–Baxter's state with almost no sticking towards the surface.32 Furthermore, these superhydrophobic coatings were intact under normal circumstances for a period of more than 6 months.
Table 1 The naming, precursor and methylation agent of methylated silica aerogels (MSAs)
Silica aerogels |
Precursor |
Methylation agent |
MSA-I |
Sodium silicate |
TMCS |
MSA-II |
TEOS |
TMCS |
MAS-III |
TEOS |
HMDS |
2.3 Self-cleaning properties
Alternative surfaces that clean themselves by water flow are known as self-cleaning surfaces. Especially on superhydrophobic surfaces, the accumulated dust particles can be easily washed off by rolling water drops.33,34 The self-cleaning property of the superhydrophobic P-MSA-I coating was verified, as shown in Fig. 4. The P-MSA-I coating was contaminated at first by dust particles, as shown in Fig. 4(a). A hanging droplet of nearly 15 μl was produced from a syringe and slowly dragged on the dust-accumulated area. The droplets effectively collected the dust particles while rolling on the surface rapidly due to the extremely low sliding angle.
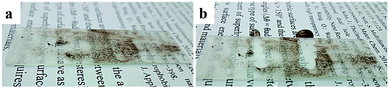 |
| Fig. 4 Optical images of (a) the heavily dusty surface and (b) the self-cleaned surface of the superhydrophobic P-MSA-I coating. | |
2.4 Abrasion resistance stability
Objective comparison of the abrasion resistance of superhydrophobic surfaces has been hampered by the lack of a single, standardized test method.35 There are many methods to analyse the mechanical properties of superhydrophobic coatings including the pencil hardness test, the sandpaper test, the scotch tape test, and the water drop/jet impact test.36–38 The methodology illustrated in Fig. 5 was applied. Sand paper (800 mesh) served as an abrasive surface, and the P-MSA-I coating was tested facing this abrasive material. While a pressure (∼2178 Pa) was applied vertically to the glass, the glass slide was moved in one horizontal direction. The advancing contact angle, receding contact angle and hysteresis changes of the coating were then measured after abrasion (10 cm in abrasion length), as shown in Fig. 6.
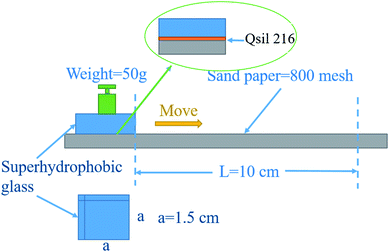 |
| Fig. 5 Schematic of the abrasion test employed to evaluate the robustness on the superhydrophobic coating. | |
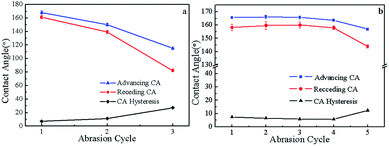 |
| Fig. 6 The effect of the sand paper abrasion cycle on the advancing contact angle, receding contact angle and contact angle hysteresis in (a) the absence of Qsil 216 and (b) the presence of Qsil 216. | |
The pure P-MSA-I coating showed poor mechanical stability, and the superhydrophobic surface was damaged completely after two abrasion cycles. However, the P-MSA-I/Qisl 216 surface remained superhydrophobic after going through at least four abrasion cycles. Even so, the contact angle hysteresis was less than 10°, which indicated that a small loading of Qsil 216 on the glass has better durability against mechanical force. PDMS, a soft polymer, could be easily scratched away from the surface. Thus, the optimum material should be obtained between the inorganic phase basement and the polymer to achieve improved mechanical stability of the coating, which in our case was the coating prepared by the addition of Qsil 216 on the surface of the basement.
It is well-known that glass is a dense silicate material with extremely poor conductivity; the chemical composition and surface texture are different from those of metal and fabric. As a result, many polymers cannot be integrated with glass substrates by electrostatic interaction or molecular diffusion. Due to the presence of many activated functional groups, such as –Si–OH groups, in the curing process, firm adhesion can occur because of strong intermolecular forces and chemical bonds between the adhesive and glass. Additionally, P-MSAs can adhere well on the surface of Qsil 216, indicating that the adhesive has lower surface tension than PDMS, which is more propitious to wetting the surface of glass.
2.5 Optical transparency
As shown in Fig. 7, pure glass transmission was about 85% in the visible range from 350 to 770 nm. When P-MSA-I was coated on the glass, the transmission decreased to 72%. Even when the P-MSA-I/Qsil 216 composite was coated, the transmission decreased to 60%, which was 70.95% of the pure glass transmission. The semi-transparent superhydrophobic glass met most of the requirements for practical applications.
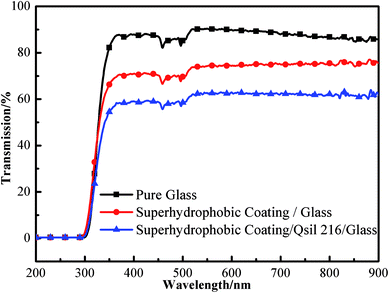 |
| Fig. 7 Optical transmission spectra of pure glass, the glass coated by P-MSA-I and the glass coated by P-MSA-I/Qsil 216 composite. | |
2.6 Thermal stability
The thermal stability was tested at the gradient of 50 °C from 150 °C to 400 °C, and the tests were carried out at a rate of 10 °C min−1 under air atmosphere for 4 h under ultimate temperature. From Fig. 8, it can be observed that the coating maintained superhydrophobic features up to 350 °C. At 400 °C, the local area of the P-MSA-I coating became hydrophilic. It was speculated that the continuous high-temperature conditions resulted in the loss of weight and therefore caused irreversible damage to the microstructure. The effects of temperature on the advancing contact angle, the receding contact angle and the contact angle hysteresis are shown in Fig. 8. The coating possessed excellent superhydrophobic features (θAdv, θRec ≥ 155°) and remarkable self-cleaning properties (θCAH ≤ 10) (Fig. 9).
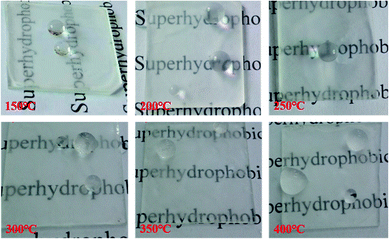 |
| Fig. 8 Optical images of water droplets on the glass coated by the P-MSA-I/Qsil 216 composite after high temperature calcination for 4 h. | |
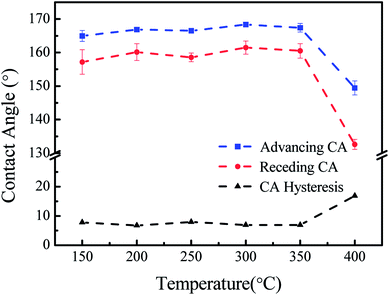 |
| Fig. 9 The effects of temperature on the advancing contact angle, the receding contact angle and the contact angle hysteresis. | |
3. Experimental
3.1 Materials
The compounds used were hydroxyl-terminated polydimethylsiloxane (PDMS, AR, M = 1000, Guangzhou Juchengzhaoye Organalsilicon Material Co. China), tetraethylorthosilicate (TEOS, AR, Tianjin Kermel Chemical Reagents Co. China), and Qsil 216 (ACC-SILICONS). Other reagents were supplied by Xilong Chemical Co., China and used as received including dimethylfumarate (DMF, AR), toluene (AR), isopropyl alcohol (AR), acetone (AR), sodium silicate (AR), trimethylchlorosilane (TMCS, AR), hexane (AR), hexamethyldisilazane (HMDS, AR), NH3·H2O (1 mol ml−1) and hydrochloric acid (HCl, 1 mol ml−1).
3.2 Preparation of methylated silica aerogel (MSA)
Ten g TEOS was hydrolysed and polymerized in a solution of 22.08 g ethanol, 2.59 g water and 0.3 ml HCl for 4 h at 85 °C. Then, 9.8 ml NH3·H2O and 1.58 g DMF were dripped into the above-mentioned solution and gelled for 8 h at 35 °C. The gel was aged further to strengthen the gel network and resist collapse during ambient drying by a two-pot method. A 50 ml EtOH/H2O solution (4V%
:
1V%) was added into the above-mentioned solution and kept for 12 h and then, the 50 ml EtOH/TEOS solution (4V%
:
1V%) was added and kept for 24 h. The as-prepared silicon aerogel was named as SA.
To obtain methylated silicon aerogel, methylation agents including TMCS/hexane (15V%
:
85V%) or HMDS/hexane (15V%
:
85V%) solutions were added in SA with strong stirring for 12 h. Subsequently, solvents were rinsed by 50 ml ethanol and hexane (4V%
:
1V%) 4 times at 50 °C and then thoroughly dried from 60 to 160 °C at a rate of 20 °C every 4 h; the obtained aerogels were named as “MSA-II” and “MAS-III”.
Additionally, for the MSA-I sample, silicon sol was obtained by the ion exchange method using sodium silicate as the silicon source, and the subsequent procedures were similar to those of the others.
3.3 Preparation of methylated silica Aerogel/PDMS composite (P-MSA)
MSA samples were smashed by ball mill grinding for 10 min at room temperature. One g MSA was modified by 0.6 g PDMS in 100 ml of toluene with stirring for 48 h. P-MSA was centrifuged with acetone and dried at 100 °C for 6 h (Table 2).
Table 2 WCA and sliding angle of P-MSA coatings
Coatings |
θCA |
θAdv |
θRec |
P-MSA-I |
160.81 ± 4° |
169.80 ± 3° |
166.18 ± 3° |
P-MSA-II |
163.28 ± 5° |
165.80 ± 3° |
155.96 ± 3° |
P-MSA-III |
165.75 ± 3° |
169.36 ± 3° |
162.37 ± 3° |
3.4 Composite coatings
The solutions of Qsil 216 silicon part-A (1 g) and hexane (50 ml) were stirred completely. Then, 0.1 g Qsil 216 silicon part-B was added. The solution was sprayed on the substrate and dried at 80 °C for 30 min. The P-MSA suspension, which was prepared by isopropyl alcohol with a concentration of 10 mg ml−1, was sprayed onto the Qsil 216 layer and then, the coating was dried at 80 °C for 2 h.
3.5 Characterization of the P-MSA coatings
The water contact and sliding angles were measured by an instrument (JC2000C, China) at room temperature. The roughness and morphology of the coating surface were characterized by atomic force microscopy (AFM, Dimension Icon, Germany) and scanning electron microscopy (SEM, SUPRA 55, Germany). The components of the materials were analysed by Fourier transform infrared spectroscopy (FTIR, Bruker TENSOR27, Germany).
4. Conclusions
A facile and scalable method to prepare superhydrophobic coatings was reported in detail. The semi-transparent coatings exhibited excellent durability, self-cleaning, and superhydrophobicity as well as excellent thermal and mechanical stabilities. The advancing water contact angle and sliding angle were 169.80 ± 3° and 4°, respectively, and these were remarkable results. The coating maintained water repellency under 350 °C for at least 4 h and possessed durable superhydrophobicity for 6 months at ambient conditions. Additionally, it remained superhydrophobic after at least four rounds of abrasion with sandpaper under more than 2000 Pa. This novel coating with self-cleaning, antifouling, low-drag, and anti-smudge properties can be used in applications where superhydrophobicity is important.
Conflicts of interest
There are no conflicts to declare.
Acknowledgements
This work is supported by the Daqing Oilfield Postdoctorate Working Station. The authors also appreciate the financial support of “the Fundamental Research Funds for the Central Universities” (Grant No. HIT. NSRIF. 2017026), the Program of Heilongjiang Province (Project No. GC13A105), the National Natural Science Foundation of China (Grant No. 51302052), and the National Science Fund for Distinguished Young Scholars of China (Grant No. 51325201).
Notes and references
- T. M. Schutzius, I. S. Bayer, M. K. Tiwari and C. M. Megaridis, Ind. Eng. Chem. Res., 2011, 50, 11117–11123 CrossRef.
- L. Feng, Z. Yan, X. Qiang, Y. Liu and Y. Wang, Appl. Phys. A, 2016, 122, 165 CrossRef.
- X. Du, J. S. Li, L. X. Li and P. A. Levkin, J. Mater. Chem. A, 2012, 1, 1026–1029 RSC.
- X. Du, X. Li and J. He, ACS Appl. Mater. Interfaces, 2010, 2, 2365–2372 CrossRef PubMed.
- M. Fang, Z. Tang, H. Lu and S. Nutt, J. Mater. Chem., 2011, 22, 109–114 RSC.
- H. Jin, M. Kettunen, A. Laiho, H. Pynnönen, J. Paltakari, A. Marmur, O. Ikkala and R. H. Ras, Langmuir, 2011, 27, 1930–1934 CrossRef PubMed.
- W. Zhang, Z. Shi, F. Zhang, X. Liu, J. Jin and L. Jiang, Adv. Mater., 2013, 25, 2071–2076 CrossRef PubMed.
- S. Hoshian, V. Jokinen, V. Somerkivi, A. R. Lokanathan and S. Franssila, ACS Appl. Mater. Interfaces, 2015, 7, 941 CrossRef PubMed.
- J. Cheng, Y. Zhang, Q. Wang and T. Wang, J. Appl. Polym. Sci., 2013, 129, 2959–2965 CrossRef.
- P. Wang, H. Han, J. Li, X. Fan, H. Ding and J. Wang, Appl. Phys. A, 2016, 122, 53 CrossRef.
- H. Ogihara, J. Xie, J. Okagaki and T. Saji, Langmuir, 2012, 28, 4605–4608 CrossRef PubMed.
- G. R. J. Artus and S. Seeger, Ind. Eng. Chem. Res., 2012, 51, 2631–2636 CrossRef.
- S. H. Park, S. M. Lee, H. S. Lim, J. T. Han, D. R. Lee, H. S. Shin, Y. Jeong, J. Kim and J. H. Cho, ACS Appl. Mater. Interfaces, 2010, 2, 658 CrossRef PubMed.
- K. C. Chang, H. I. Lu, C. W. Peng, M. C. Lai, S. C. Hsu, M. H. Hsu, Y. K. Tsai, C. H. Chang, W. I. Hung and Y. Wei, ACS Appl. Mater. Interfaces, 2013, 5, 1460–1467 CrossRef PubMed.
- D. Ebert and B. Bhushan, Langmuir, 2012, 28, 11391 CrossRef PubMed.
- X. Du and J. He, ACS Appl. Mater. Interfaces, 2011, 3, 1269–1276 CrossRef PubMed.
- M. Petr, J. Hanuš, O. Kylián, J. Kratochvíl, P. Solař, D. Slavínská and H. Biederman, Mater. Lett., 2016, 167, 30–33 CrossRef.
- S. Gao, J. Huang, S. Li, H. Liu, F. Li, Y. Li, G. Chen and Y. Lai, Mater. Des., 2017, 128, 1–8 CrossRef.
- K. Ellinas, S. P. Pujari, D. A. Dragatogiannis, C. A. Charitidis, A. Tserepi, H. Zuilhof and E. Gogolides, ACS Appl. Mater. Interfaces, 2014, 6, 6510 CrossRef PubMed.
- S. M. Kang, S. M. Kim, H. N. Kim, M. K. Kwak, D. H. Tahk and K. Y. Suh, Soft Matter, 2012, 8, 8563–8568 RSC.
- Z. Pan, H. Shahsavan, W. Zhang, F. K. Yang and B. Zhao, Appl. Surf. Sci., 2015, 324, 612–620 CrossRef.
- X. Liu, Y. Xu, K. Ben, Z. Chen, Y. Wang and Z. Guan, Appl. Surf. Sci., 2015, 339, 94–101 CrossRef.
- X. Liu, Y. Wang, Z. Chen, K. Ben and Z. Guan, Appl. Surf. Sci., 2016, 360, 789–797 CrossRef.
- G. Ren, Y. Song, X. Li, B. Wang, Y. Zhou, Y. Wang, B. Ge and X. Zhu, J. Colloid Interface Sci., 2018, 57–62 CrossRef PubMed.
- L. Zhang, C. H. Xue, M. Cao, M. M. Zhang, M. Li and J. Z. Ma, Chem. Eng. J., 2017, 320, 244–252 CrossRef.
- Y. Zhang, F. Ren and Y. Liu, Appl. Surf. Sci., 2018, 436, 405–410 CrossRef.
- W. Ding, Z. Zhang, Y. Li and C. Xu, ACS Appl. Mater. Interfaces, 2014, 5, 10014–10021 Search PubMed.
- L. Xu, R. G. Karunakaran, J. Guo and S. Yang, ACS Appl. Mater. Interfaces, 2012, 4, 1118 CrossRef PubMed.
- A. Lafuma and D. Quéré, Nat. Mater., 2003, 2, 457–460 CrossRef PubMed.
- Q. F. Xu, J. N. Wang and K. D. Sanderson, ACS Nano, 2010, 4, 2201–2209 CrossRef PubMed.
- Y. Raichman, M. Kazakevich, E. Rabkin and Y. Tsur, Adv. Mater., 2006, 18, 2028–2030 CrossRef.
- S. Liu, S. S. Latthe, H. Yang, B. Liu and R. Xing, Ceram. Int., 2015, 41, 11719–11725 CrossRef.
- S. Pitchaimuthu, S. S. Latthe, C. Ravidhas, C. A. Jennifer, K. D. David, R. Venkatesh, A. Devadoss, C. Terashima, K. Nakata and A. Fujishima, Crystengcomm, 2015, 17, 2624–2628 RSC.
- Y. Lu, S. Sathasivam, J. Song, C. R. Crick, C. J. Carmalt and I. P. Parkin, Science, 2015, 347, 1132–1135 CrossRef PubMed.
- T. Verho, C. Bower, P. Andrew, S. Franssila, O. Ikkala and R. H. Ras, Adv. Mater., 2011, 23, 673–678 CrossRef PubMed.
- Y. Xiu, Y. Liu, D. W. Hess and C. P. Wong, Nanotechnology, 2010, 21, 155705 CrossRef PubMed.
- S. Liu, X. Liu, S. S. Latthe, G. Li, S. An, S. S. Yoon, B. Liu and R. Xing, Appl. Surf. Sci., 2015, 351, 897–903 CrossRef.
- S. S. Latthe, C. Terashima, K. Nakata, M. Sakai and A. Fujishima, J. Mater. Chem. A, 2014, 2, 5548–5553 RSC.
|
This journal is © The Royal Society of Chemistry 2018 |
Click here to see how this site uses Cookies. View our privacy policy here.