DOI:
10.1039/C8RA05057K
(Paper)
RSC Adv., 2018,
8, 26928-26933
Evaluation of performance and microbial community successional patterns in an integrated OCO reactor under ZnO nanoparticle stress
Received
12th June 2018
, Accepted 19th July 2018
First published on 30th July 2018
Abstract
An integrated OCO reactor was used to investigate the performance and microbial community successional changes under long-term exposure to relatively low levels of ZnO nanoparticles (NPs). Relatively higher concentrations of ZnO NPs (1.5 mg L−1) could adversely affect the nitrogen and phosphorus removal in the reactor. The diversity and richness of the microbial communities chronically declined with an increasing concentration of ZnO NPs higher than 1.5 mg L−1. With the elevated ZnO NPs, the phyla abundances of Proteobacteria, Firmicutes and Actinobacteria decreased slightly, whereas those of Bacteroidetes and Acidobacteria increased. Bacteroidetes and Proteobacteria were the predominant phyla in each phase (with a variation in abundance), together with some common taxa responses to ZnO NP stress as revealed by Venn diagram analysis. Some genera associated with the removal of nitrogen and phosphorus, such as Acinetobacter, Stenotrophomonas and Pseudomonas, decreased significantly. The present results are significant for expanding our understanding of the functional performance and microbial community successions of activated sludge which has experienced long-term exposure to environmentally relevant concentrations of ZnO NPs.
1. Introduction
With the rapid development of nanotechnology, the application of engineered nanomaterials (ENMs) in a variety of industrial products and consumer goods has accelerated. Among ENMs, ZnO nanoparticles (ZnO NPs) together with titanium dioxide NPs and silver NPs are the most frequently used inorganic species in these industrial products and consumer goods.1 Taking into consideration that NPs might enter into domestic sewage and industrial wastewater systems, their potential effects on the environment and human health have raised increasing concerns.
ZnO NPs are widely used in paints, coatings, cosmetics, photocatalysts, therapeutics, drug delivery systems and semiconductors, due to their antimicrobial, catalytic and ultraviolet-protective properties.2 Previous studies reported the occurrence of ZnO NPs in wastewater treatment plants (WWTPs).3,4 Considering that activated sludge plays a crucial role in biological wastewater treatment, the interaction of activated sludge with NPs may compromise the performance of WWTPs. Currently, most reports have focused on the toxic effects of ZnO NPs on specific bacteria, such as Escherichia coli5 and Pseudomonas stutzeri.6 The impact of ZnO NPs on the microbial communities of the activated sludge has also been investigated recently. Based on high-throughput pyrosequencing, Wang et al. reported that the abundance of nitrite oxidizing bacteria (NOB) increased but the abundance of ammonium oxidizing bacteria (AOB) and denitrifying bacteria was relatively stable in a membrane bioreactor (MBR);7 Zhang et al. reported the toxicity effect of ZnO NPs on nitrogen removal and microbial communities in a CANON reactor.8
Although valuable findings have been documented based on current organismal studies, it is necessary to present a comprehensive understanding that microbial communities act as a whole in maintaining the key functions of a complex environment from an ecological perspective. Several studies have focused on the long-term effects of elevated ZnO NP concentrations on the microbial communities in wastewater treatment systems.8–10 In these studies, the ZnO NP concentrations used were relatively high (over 5 mg L−1), although admittedly, high concentrations of NPs are required to function in traditional toxicity assays,11 meanwhile the environmentally relevant concentration of NPs increases inevitably.9 Given that the highest concentration of ZnO NPs in WWTPs reaches 300 μg L−1,4 and 5 mg L−1 of ZnO NPs causes no inhibitory effects on the microbial growth,8 we hypothesized that relatively lower concentrations of NPs may have an unpredictable influence on the activated sludge systems over time. Research should provide insight into the long-term effects of environmentally relevant concentrations (or higher by about an order of magnitude) of ZnO NPs on the ecological function of microbial communities in activated sludge.
In the present study, activated sludge systems in an integrated OCO reactor (an anaerobic/anoxic/oxic (A2/O) process with a shape similar to the letters of the alphabet “OCO”)12 were treated with various concentrations of ZnO NPs (0.25 mg L−1, 0.8 mg L−1, 1.5 mg L−1 and 4 mg L−1) for a performance period of 80 days, and the shifts in the microbial communities were revealed using Illumina Miseq pyrosequencing of the 16S rRNA gene. The objectives of this study were as follows: (1) to evaluate the ecotoxicity of ZnO NPs on wastewater treatment systems, and (2) to illustrate the responses of microbial communities to the long-term exposure of relatively lower concentrations of ZnO NPs.
2. Materials and methods
2.1. Experimental set-up and operation
The integrated OCO reactor with an effective volume of 240 L has been operating for more than one year, and the reactor has been described previously.12 The synthetic wastewater consisted of glucose, sucrose, soluble starch, NH4HCO3, KH2PO4, and a trace element solution (including MgCl2, CaCl2, MnSO4, CoCl2, CuSO4, FeSO4 and H3BO3) as previously reported.13 The parameters COD, TN and TP of the synthetic wastewater were adjusted to about 300 mg L−1, 37 mg L−1 and 5 mg L−1, respectively. The hydraulic retention time was 12 h (1.6 h for the anaerobic zone, 3.3 h for the anoxic zone, 4.8 h for the aerobic zone, and 2.3 h for the mixed zone and sedimentation tanks). The influences of the ZnO NPs on nutrient (nitrogen and phosphorus) removal and on the microbial community were investigated after the reactor reached a relatively steady state.
2.2. Nanoparticle and preparation of nanoparticle suspensions
ZnO nanoparticles were purchased from Shanghai Hansi Chemical Industry Co. Ltd (Shanghai, China), and the average diameter of the nanoparticles was about 30 nm. The size and shape of the nanoparticles were also characterized via transmission electron microscopy (TEM) images using an FEI Tecnai G20 microscope with a voltage of 200 kV (FEI company, USA). A nanoparticle stock suspension of 100 mg L−1 ZnO NPs was concocted by adding 0.1 g ZnO NPs to 1 L Milli-Q water, with sonication (300 W) for 20 min. Then, various concentrations of ZnO NP suspensions were prepared by diluting the stock suspension with Milli-Q water.
2.3. Batch experiments with exposure to ZnO NPs
The integrated OCO reactor was initiated with the inoculation of activated sludge from a full-scale wastewater treatment plant in Maoming, Guangdong, China. Prior to the addition of ZnO NP suspensions in the influent, the integrated OCO reactor had operated continuously for 20 d maintaining a steady operation performance. Then, ZnO NP suspensions were freshly prepared and added to the reactor with final concentrations of 0.25 mg L−1, 0.8 mg L−1, 1.5 mg L−1 and 4 mg L−1 for continuous treatment, and the duration time of each treatment was 20 d.
2.4. Analytical methods
The temperature, dissolved oxygen (DO) and pH values were measured using portable apparatus with specific probes (WTW, Germany). NH4+–N, NO2−, NO3−, the total phosphorus (TP) and the chemical oxygen demand (COD) were measured according to standard methods.14 The total nitrogen (TN) was calculated as the sum of NH4+–N, NO2− and NO3−.
2.5. Pyrosequencing and phylogenetic assignment
The total genomic DNA from each sludge sample was extracted and purified using the FastDNA® Spin Kit for Soil (MP-Bio, USA) according to the manufacturer’s instructions. The DNA concentration and quality were detected with 1% agarose gel electrophoresis and a NanoDrop Spectrophotometer. The partial 16S rRNA genes were amplified with barcoded primers 515F (5′-GTGCCAGCMGCCGCGGTAA-3′) and 909R (5′-CCCCGYCAATTCMTTTRAGT-3′), and pyrosequencing was carried out using an Illumina Miseq sequencing platform.15 More than 10
000 sequences with a 350 bp length were obtained from each sample. The aligned sequences were used for a chimera check using the Uchime algorithm.16 Taxonomic classifications were performed using the Ribosomal Database Project classifier17 with a set confidence threshold of 80%, and the operational taxonomic units (OTUs) were defined at a 3% dissimilarity cutoff. The Shannon diversity index, Chao 1 index, ACE index and Simpson index were analyzed by the QIIME software package. The similarities and differences among these communities were analyzed through Venn diagrams with shared and unique OTUs.18
3. Results and discussion
3.1. Effects of ZnO NPs on reactor performance
The morphological characterizations of the ZnO NPs are shown in Fig. 1, and the average diameter of the nanoparticles was 30 nm. The performance of the OCO reactor in each phase has been depicted in Fig. 2. During the whole experiment, the influent concentrations of ammonia and phosphorus were constantly kept around 37 mg L−1 and 5 mg L−1, respectively. The concentration of the ZnO NPs was increased step-wise during each phase except in phase P0 (which acted as the control).
 |
| Fig. 1 High-resolution TEM image of the ZnO nanoparticles. | |
 |
| Fig. 2 Reactor performance on nitrogen removal in each phase with various concentrations of ZnO NPs. | |
For nitrogen removal, no nitrite accumulation was observed throughout the dosing phases, indicating that the oxidation of nitrite remained unaffected. The nitrogen removal rate (NRR) reached an average of 0.055 kg m−3 d−1 (Table 1) in P0 with effluent nitrate at 7.55 mg L−1 and a low ammonia level. Then in phase P1, the ZnO NP stock suspension was directly added to the reactor for a concentration of 0.25 mg L−1 in the system. The NRR increased and reached an average of 0.060 kg m−3 d−1 (Table 1), and this result was mainly attributed to the additional removal of nitrate, as Zn2+ ions can be released from the ZnO NPs (0.13 mg L−1 Zn2+ ions within 1 mg L−1 ZnO NPs in wastewater),19 and low Zn2+ concentrations provide essential micronutrients for vital cofactors of metalloproteinases and certain enzymes thereby enhancing the bioactivities of nitrifiers and denitrifiers.20 Subsequently, the ZnO NP concentration was further increased to 0.8 mg L−1 in phase P2, and the NRR decreased significantly to an average of 0.049 kg m−3 d−1 (p < 0.05); both the ammonia and nitrate in the effluent presented an increasing trend. Then in phase P3, the ZnO NP concentration was further increased to 1.5 mg L−1 and the NRR exhibited a small decrease (0.047 kg m−3 d−1). This indicated that nitrifiers were significantly suppressed. Finally, in phase P4, as the ZnO NP concentration was increased to 4 mg L−1, the NRR remained almost unchanged (0.047 kg m−3 d−1), meanwhile with a decline in the effluent nitrate and a remarkable increase in the effluent ammonia, the ammonia removal rate had dramatically decreased to 41.48% by the last day. The decrease in the ammonia removal rate may be due to a long-lasting inhibition of nitrification, which was strengthened by the accumulative effect of the ZnO NPs. These results coincided with previous studies which proved that ammonia removal is inhibited by inhibition of the respiration of nitrifying bacteria21 or enzyme activity.22
Table 1 Operational conditions and nutrient removal performances (average data of each phase)
Phase |
Period (d) |
ZnO NPs (mg L−1) |
Inf. NH4+ (mg L−1) |
NRR (kg m−3 d−1) |
ΔNO3−/ΔNH4+ |
MLVSS (mg L−1) |
P0 |
0–20 |
0 |
35.5 |
0.055 |
0.217 |
2016 |
P1 |
21–40 |
0.25 |
35.2 |
0.060 |
0.141 |
2163 |
P2 |
41–60 |
0.8 |
39.3 |
0.049 |
0.316 |
1807 |
P3 |
61–80 |
1.5 |
37.5 |
0.047 |
0.285 |
1579 |
P4 |
81–100 |
4.0 |
36.8 |
0.047 |
0.127 |
1261 |
For phosphorus removal, TP removal rate was kept above 90% in phases P0, P1 and P2 (Fig. 3). This implies the negligible influence of the ZnO NPs on TP removal below a concentration of 0.8 mg L−1. When the concentration of ZnO NPs increased to 1.5 mg L−1 or higher, TP removal dramatically decreased. These results illustrate that the growth and activity of polyphosphate accumulating organisms (PAOs) are inhibited through the anaerobic release and aerobic uptake of phosphorus, thereby phosphorus removal clearly drops.23,24 However, COD removal was unaffected during the whole experimental period (data not shown). A previous study also reported that COD removal was not impacted adversely until the concentration of ZnO NPs reached as high as 68 mg L−1.25
 |
| Fig. 3 Reactor performance on phosphorus removal in each phase with various concentrations of ZnO NPs. | |
3.2. Responses of the microbial community successional patterns
3.2.1 Effects of ZnO NPs on microbial community diversities. To further understand the effect of ZnO NPs on the bacterial community diversity and richness in the reactor during long-term exposure, high-throughput sequencing was performed using an Illumina Miseq system. The Good’s coverage values of five samples were about 0.9, indicating that the bacterial communities could well represent the total sequences of the activated sludge samples. Based on a 97% similarity of sequences, the retrieved sequences were clustered into 1320, 1270, 1160, 1135 and 1363 operational taxonomic units (OTUs) at ZnO NP concentrations of 0, 0.25, 0.8, 1.5 and 4 mg L−1, respectively (Table 2).
Table 2 Sequencing results of the sludge sample in different phases
Sample |
Sequence numbers |
OTU number |
Shannon index |
Chao1 index |
ACE index |
Simpson index |
P0 |
49 534 |
1320 |
10.30 |
6421.45 |
7117.18 |
0.997 |
P1 |
44 043 |
1270 |
10.09 |
6322.17 |
7250.18 |
0.997 |
P2 |
31 183 |
1160 |
9.62 |
6379.32 |
7452.33 |
0.995 |
P3 |
33 788 |
1135 |
9.33 |
5723.52 |
6530.43 |
0.994 |
P4 |
36 773 |
1363 |
9.84 |
5407.57 |
6428.06 |
0.997 |
The Shannon index, Simpson index, ACE index and Chao 1 index are commonly used for indicating the microbial diversity and richness.26 Compared with 0 mg L−1 ZnO NPs, the Shannon, Chao 1 and ACE indexes showed significant changes at 1.5 and 4 mg L−1 ZnO NPs (Table 2), suggesting that the presence of the ZnO NPs affected the microbial diversity and richness of the activated sludge in the OCO reactor.
3.2.2 Successional changes to the microbial community under ZnO NP stress. As the microbial diversity and richness of the activated sludge were impacted by the ZnO NPs, the microbial community patterns at the phylum level for each phase are further illustrated in Fig. 4. The prominent phyla of the five sludge samples consisted of Proteobacteria (38.21–43.44%), Bacteroidetes (27.47–43.36%), Firmicutes (4.96–10.00%), Chloroflexi (2.95–5.30%), Acidobacteria (2.20–6.75%), Actinobacteria (1.60–2.59%), Cyanobacteria (0.61–1.67%) and TM7 (0.17–1.74%) at various ZnO NP concentrations. As the concentrations of the ZnO NPs increased from 0 mg L−1 to 4 mg L−1, the variation in the microbial phyla abundance showed that the proportions of Proteobacteria, Firmicutes and Actinobacteria decreased slightly, whereas those of Bacteroidetes and Acidobacteria increased. Previous studies reported that Proteobacteria and Bacteroidetes were the predominant phyla in most full-scale WWTPs,27,28 together with the subdominant phyla Firmicutes and Acidobacteria.29 Specifically, Proteobacteria could play a crucial role in the removal of organic waste and nutrients.30,31 Chloroflexi are also common filamentous bacteria in the biological treatment processes of wastewater.32 At the genus level, Acinetobacter, Stenotrophomonas, Pseudomonas and Bacteroides decreased with increasing ZnO NP concentrations (Fig. 5). Previous studies showed that members of the genus Acinetobacter were typical bacteria present in sewage33 and versatile in the biodegradation of various pollutants,34 and Stenotrophomonas and Pseudomonas were classified as organophosphorus-degrading bacteria.35 On the other hand, Dechloromonas and Sediminibacterium firstly increased with increasing ZnO NP concentrations up to 1.5 mg L−1 (P0–P3), and decreased at the ZnO NP concentration of 4 mg L−1 (P4). Previous reports illustrated that Dechloromonas were denitrifiers36 or capable of reducing nitrate or nitrite to nitrogen gas under autotrophic conditions.37 Dechloromonas also exhibited high tolerance to ZnO NPs in an SBR38 and an increasing tendency with elevated ZnO NP concentrations.39
 |
| Fig. 4 Phylum-level distribution of microbial communities in the reactor. | |
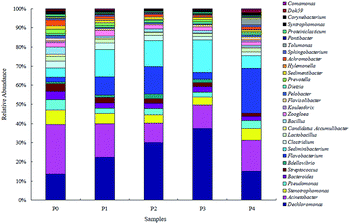 |
| Fig. 5 Taxonomic classification of sequences at bacterial genus level in the dominated classes. | |
Microbial similarities and differences of activated sludge samples were analyzed by a Venn diagram (Fig. 6). The total of observed OTUs in all five communities was 3074, but only 283 OTUs (9.2%) of the total OTUs were shared among them (Fig. 6). The shared OTUs in the five communities indicated that some microbes existed in the activated sludge during the whole operational period. Furthermore, the majority of the shared OTUs at the phyla level were Proteobacteria (35.01%) and Bacteroidetes (30.46%), followed by Firmicutes (10.79%), Chloroflexi (6.71%) and Acidobacteria (2.64%). The number of OTUs that were unique to each community were counted at 316 (P0), 283 (P1), 211 (P2), 262 (P3) and 484 (P4), and together they accounted for 90.8% of the total number of observed OTUs, suggesting that ZnO NPs altered the microbial community compositions with long-term exposure.
 |
| Fig. 6 Venn diagrams based on high-throughput sequencing of the microbial community at the P1–P4 stages (OTU at 3% difference). The shared OTUs were analyzed at the phylum level. | |
4. Conclusions
The long-term exposure of low-level ZnO NPs caused adverse impacts on the removal of nitrogen and phosphorus in an integrated OCO reactor. ZnO NPs resulted in the chronic decline of microbial diversity and richness. The shifts in the microbial communities revealed that Bacteroidetes and Proteobacteria were the predominant phyla, and even some common taxa existing in the activated sludge all the time experienced dramatic variations in the composition of the microbial species. The present results are significant in understanding the functional performance and microbial community successions of activated sludge under much lower ZnO NP stress.
Conflicts of interest
There are no conflicts to declare.
Acknowledgements
This research was supported by the National Natural Science Foundation of China (21777034), the China Scholarship Council (201708440543), the Natural Science Foundation of Guangdong Province, China (2014A030307019, 2015A030313801), the Science and Technology Planning Project of Guangdong Province, China (2014A020216046), the Special Innovation Project of Guangdong Colleges and Universities, China (2017KTSCX129), the Young Creative Talents Project of Department of Education of Guangdong Province (2015KQNCX102) and the Science and Technology Planning Project of Maoming (2014044).
References
- Y. Yang, C. Zhang and Z. Hu, Environ. Sci.: Processes Impacts, 2013, 15, 39–48 RSC.
- A. Nel, T. Xia, L. Mädler and N. Li, Science, 2006, 311, 622–627 CrossRef PubMed.
- F. Gottschalk, T. Sonderer, R. W. Scholz and B. Nowack, Environ. Sci. Technol., 2009, 43, 9216–9222 CrossRef PubMed.
- T. Y. Sun, F. Gottschalk, K. Hungerbühler and B. Nowack, Environ. Pollut., 2014, 185, 69–76 CrossRef PubMed.
- M. Li, L. Zhu and D. Lin, Environ. Sci. Technol., 2011, 45, 1977–1983 CrossRef PubMed.
- Q. Chen, T. Li, M. Gui, S. Liu, M. Zheng and J. Ni, Bioresour. Technol., 2017, 239, 21–27 CrossRef PubMed.
- Z. Wang, F. Huang, X. Mei, Q. Wang, H. Song, C. Zhu and Z. Wu, J. Membr. Sci., 2014, 471, 258–264 CrossRef.
- X. Zhang, N. Zhang, H. Fu, T. Chen, S. Liu, S. Zheng and J. Zhang, Bioresour. Technol., 2017, 243, 93–99 CrossRef PubMed.
- M. Tan, G. Qiu and Y.-P. Ting, Bioresour. Technol., 2015, 185, 125–133 CrossRef PubMed.
- N.-Q. Puay, G. Qiu and Y.-P. Ting, J. Cleaner Prod., 2015, 88, 139–145 CrossRef.
- J. W. Metch, N. D. Burrows, C. J. Murphy, A. Pruden and P. J. Vikesland, Nat. Nanotechnol., 2018, 13, 253–259 CrossRef PubMed.
- D. Li, Y. Mao, Z. Liu, X. Yin, C. Lang and Y. Liu, Environ. Technol., 2014, 35, 2628–2633 CrossRef PubMed.
- Z. Liu, H. Zhou, D. Li, X. Yin, Y. Mao and W. Chen, Technol. Water Treat., 2017, 43, 104–107 Search PubMed.
- APHA, Standard Methods for the Examination of Water and Wastewater, American Public Health Association/American Water Works Association/Water Environment Federation, Washington, DC, 2005 Search PubMed.
- J. G. Caporaso, J. Kuczynski, J. Stombaugh, K. Bittinger, F. D. Bushman, E. K. Costello, N. Fierer, A. G. Pena, J. K. Goodrich, J. I. Gordon, G. A. Huttley, S. T. Kelley, D. Knights, J. E. Koenig, R. E. Ley, C. A. Lozupone, D. McDonald, B. D. Muegge, M. Pirrung, J. Reeder, J. R. Sevinsky, P. J. Tumbaugh, W. A. Walters, J. Widmann, T. Yatsunenko, J. Zaneveld and R. Knight, Nat. Methods, 2010, 7, 335–336 CrossRef PubMed.
- R. C. Edgar, B. J. Haas, J. C. Clemente, C. Quince and R. Knight, Bioinformatics, 2011, 27, 2194–2200 CrossRef PubMed.
- Q. Wang, G. M. Garrity, J. M. Tiedje and J. R. Cole, Appl. Environ. Microbiol., 2007, 73, 5261–5267 CrossRef PubMed.
- L. Lu, D. Xing and N. Ren, Water Res., 2012, 46, 2425–2434 CrossRef PubMed.
- D. Q. Zhang, C. Y. Eng, D. C. Stuckey and Y. Zhou, Chemosphere, 2017, 171, 446–459 CrossRef PubMed.
- A. Daverey, Y.-C. Chen, S. Sung and J.-G. Lin, Bioresour. Technol., 2014, 165, 105–110 CrossRef PubMed.
- L. Hou, J. Xia, K. Li, J. Chen, X. Wu and X. Li, Water Sci. Technol., 2013, 67, 254–260 CrossRef PubMed.
- S. T. Wang, S. P. Li, W. Q. Wang and H. You, RSC Adv., 2015, 5, 67335–67342 RSC.
- E. Lombi, E. Donner, E. Tavakkoli, T. W. Turney, R. Naidu, B. W. Miller and K. G. Scheckel, Environ. Sci. Technol., 2012, 46, 9089–9096 CrossRef PubMed.
- G. Liu, D. Wang, J. Wang and C. Mendoza, Sci. Total Environ., 2011, 409, 2852–2857 CrossRef PubMed.
- F. Huang, Z. Wang, X. Mei and Z. Wu, Technol. Water Treat., 2013, 39, 14–18 Search PubMed.
- K. Meli, I. Kamika, J. Keshri and M. N. B. Momba, Sci. Rep., 2016, 6, 39176 CrossRef PubMed.
- M. Hu, X. Wang, X. Wen and Y. Xia, Bioresour. Technol., 2012, 117, 72–79 CrossRef PubMed.
- D. Shu, Y. He, H. Yue and Q. Wang, Bioresour. Technol., 2015, 186, 163–172 CrossRef PubMed.
- S. Xia, L. Duan, Y. Song, J. Li, Y. M. Piceno, G. L. Andersen, L. Alvarez-Cohen, I. Moreno-Andrade, C.-L. Huang and S. W. Hermanowicz, Environ. Sci. Technol., 2010, 44, 7391–7396 CrossRef PubMed.
- C. O. Jeon, D. S. Lee and J. M. Park, Water Res., 2003, 37, 2195–2205 CrossRef PubMed.
- Y. Yang, J. Quensen, J. Mathieu, Q. Wang, J. Wang, M. Li, J. M. Tiedje and P. J. J. Alvarez, Water Res., 2014, 48, 317–325 CrossRef PubMed.
- L. Björnsson, P. Hugenholtz, G. W. Tyson and L. L. Blackall, Microbiology, 2002, 148, 2309–2318 CrossRef PubMed.
- J. Snaidr, R. Amann, I. Huber, W. Ludwig and K. H. Schleifer, Appl. Environ. Microbiol., 1997, 63, 2884–2896 Search PubMed.
- S. i. Ishii, J. Koki, H. Unno and K. Hori, Appl. Environ. Microbiol., 2004, 70, 5026–5029 CrossRef PubMed.
- B. K. Singh, Nat. Rev. Microbiol., 2009, 7, 156–164 CrossRef PubMed.
- K. Heylen, B. Vanparys, L. Wittebolle, W. Verstraete, N. Boon and P. De Vos, Appl. Environ. Microbiol., 2006, 72, 2637–2643 CrossRef PubMed.
- J. Ma, Z. Wang, D. He, Y. Li and Z. Wu, Water Res., 2015, 78, 98–110 CrossRef PubMed.
- Z. Liu, H. Zhou, J. Liu, X. Yin, Y. Mao, Z. Liu, Z. Li and W. Xie, RSC Adv., 2016, 6, 110108–110111 RSC.
- S. Wang, M. Gao, Z. She, D. Zheng, C. Jin, L. Guo, Y. Zhao, Z. Li and X. Wang, Bioresour. Technol., 2016, 216, 428–436 CrossRef PubMed.
|
This journal is © The Royal Society of Chemistry 2018 |
Click here to see how this site uses Cookies. View our privacy policy here.