DOI:
10.1039/C8RA05682J
(Paper)
RSC Adv., 2018,
8, 33459-33463
A simple and highly selective 1,2,4,5-tetrazine-based colorimetric probe for HSO3− ion recognition in food†
Received
3rd July 2018
, Accepted 21st September 2018
First published on 28th September 2018
Abstract
A series of 3,6-bis-substituted-1,2,4,5-tetrazine-based colorimetric probes has been developed in good yields that exhibits highly selective and sensitive colorimetric recognition of HSO3− in aqueous solution. The color of the solution containing colorimetric probes changed markedly from orange to colorless, upon the addition of HSO3−. Quantification of the absorption titration analysis shows that the detection limit of 3,6-bis(2-aminoethylamino)-1,2,4,5-tetrazine (2a) for HSO3− was 3.8 μM. It was noted that 3,6-bis-substituted-1,2,4,5-tetrazine-based colorimetric probes have a specific response toward HSO3− without interference from other 17 different anions and 16 common cations. A plausible mechanism was proposed by high-resolution mass spectroscopy analysis and NMR spectrometry analysis, involving the nucleophilic reaction of a bisulfite anion with the tetrazine ring and free radical rearrangement of ·SO3H. Moreover, probes 2a–2c possessed applicability for sensing bisulfite in actual food samples. Therefore, the present work established a novel strategy for investigating bisulfite in food or other products.
Introduction
Sulfur dioxide (SO2) is a pungent gas and major atmospheric pollutant that can easily be converted into its bisulfite (HSO3−) and sulfite (SO32−) derivatives under humid conditions.1,2 Owing to their ability to inhibit mildew and restrict microorganism growth, SO2 derivatives are commonly used as preservatives and antioxidants in food, beverages, and drugs such as epinephrine.3,4 Furthermore, endogenous sulfur dioxide derivatives are involved in many physiological processes.5,6 For example, bisulfite generated in mitochondria reduces blood pressure and aids blood vessel dilation.7 However, recent research has shown that a high bisulfite concentration in the body is not only correlated with the occurrence of allergic reactions,8 but can also induce respiratory responses, neurological disorders, cardiovascular disease, and lung cancer.9–12 Therefore, developing a highly sensitive and selective method for bisulfite detection is important for food safety, quality control, and human welfare.13
Currently, detection methods for HSO3− include titrimetry,14 flow injection analysis, electrochemistry,15 and spectroscopy.16 Spectroscopy, which has innate advantages such as high sensitivity, good selectivity, and the low detection limits, is the most attractive among these methods.17 Sensing mechanisms for HSO3− using spectroscopy include nucleophilic reactions with aldehydes,18 Michael-type additions,19,20 selective levulinate deprotection,21 hydrogen bond formation,22 and coordinative interactions.23
1,2,4,5-Tetrazines (or s-tetrazines) used in this paper are aromatic six-membered heterocycles containing four nitrogen atoms and bearing two electron-withdrawing amino substituents. The most prominent characteristic of s-tetrazines is their electron-deficient aromatic rings, which result from replacing four CH groups with four, more electronegative, nitrogen atoms on the prototypical aromatic ring.24 Computational results have shown that s-tetrazines can be used as binding units for anion recognition.25 Furthermore, the amino substituents cooperate with the four nitrogen atoms in the tetrazine ring to decrease the electron density of the carbon atoms at the 3 and 6 positions, making them susceptible to attack by nucleophilic reagents, such as HSO3−. Tetrazines are also colored due to a low-energy n–π* transition in the visible range, allowing them to be detected by the naked eye.26
Therefore, owing to the excellent optical and reaction properties of s-tetrazine, we developed novel 3,6-bis-substituted-1,2,4,5-tetrazine-based probes (2a–2c) for bisulfite anions. These probes can respond to HSO3− specifically and sensitively in aqueous solution and generate naked-eye detectable chromogenic and UV-Vis absorbance signaling. A corresponding mechanism is also proposed, as supported by the spectroscopic methods. Notably, the mechanism is unique and different from previously reported whole mechanisms. To our knowledge, this is the first study to use s-tetrazines as a probe for HSO3− anion recognition.
Experimental
Materials and methods
All materials and reagents were purchased from commercial suppliers and used without further purification. 1H and 13C NMR spectra were recorded on 600 MHz Varian VNMRS Spectrometer, using D2O or DMSO-d6 as solvent. High-resolution mass spectroscopy (HRMS) was performed on a Bruker APEXIIFT-ICR mass spectrometer. Melting points were measured on a WRS-1B micro-melting point apparatus (YiCe, Shanghai). Ultraviolet-visible (UV-Vis) spectra were recorded on Metash UV-8000s spectrophotometer. The pH values were measured using a PHS-3C digital pH meter (ShengCi, Shanghai).
Preparation for UV-Vis spectral measurements
As the probes showed excellent water solubility, experiments of 2a–2c with HSO3− were conducted in aqueous solution. 4-(2-Hydroxyethyl)-1-piperazineethanesulfonic acid (HEPES) buffer (20 mM, pH 7.0) was used for UV-Vis measurements. Compounds 2a–2c were separately dissolved in ultrapure water to obtain stock solutions (1 mM). Ultrapure water was also used to prepare stock solutions (10−2 M) of NaF, NaCl, KBr, NaI, NaOH, Na2CO3, NaHCO3, Na2SO4, KHSO4, Na2SO3, NaHSO3, NaNO3, NaNO2, Na3PO4·12H2O, Na2HPO4, NaH2PO4, CH3COONa, and Na2B4O7·10H2O. Stock solutions of NaHSO3 and Na2SO3 were freshly prepared before each use. In UV-Vis selectivity experiments, test solution was prepared by adding the stock solution of 2a, 2b, or 2c (2 mL) into a 10 mL colorimetric tube, followed by 2 mL of one of the above analyte solutions, after which the test solution was made up to 10 mL using ultrapure water. For UV-Vis titration experiments, test solution was prepared by adding the stock solution of 2a, 2b, or 2c (2 mL) into a 10 mL colorimetric tube with HEPES buffer (20 mM, pH 7.0), followed by a suitable volume of analyte solution, after which the test solution was made up to 10 mL using ultrapure water.
Determination of the detection limit
The detection limits were calculated from the UV-Vis titration results. UV-Vis spectra of 2a were measured 20 times and the standard deviation of the blank measurement was determined. To obtain the slope, UV-Vis absorbance variations at 458 nm vs. HSO3− concentration were plotted, giving a linear graph. The detection limits were calculated using the equation D = 3σ/k, where σ is the standard deviation of the blank measurement, and k is the slope.
Synthesis of probes 2a–2c
Synthesis of 3,6-bis(2-aminoethylamino)-1,2,4,5-tetrazine (2a). The synthesis of 2a was achieved following a previously reported procedure.27Tetrazine 1 (0.300 g, 1.1 mmol) was added to ethylenediamine (3.5 mL). The resultant mixture was stirred at room temperature for 4 h. Excess diamine was removed under reduced pressure to afford a red precipitate. Toluene (5 mL) was added and the resultant precipitate was collected by filtration and washed with light petroleum. The crude product was purified by recrystallization from ethyl acetate to afford 2a as a red powder (0.201 g, 92%), mp 133–135 °C. 1H-NMR (600 MHz, D2O, ppm) 3.48 (4H, t, J = 6.1 Hz), 2.87 (4H, t, J = 6.1 Hz). 13C-NMR (151 MHz, D2O, ppm) 159.84, 43.07, 39.46. HRMS (ESI) m/z calculated for C6H15N8+, 199.1414; found 199.1414.
Synthesis of 3,6-bis(3-aminopropylamino)-1,2,4,5-tetrazine (2b). Tetrazine 1 (0.300 g, 1.1 mmol) was added to 1,3-propanediamine (3.5 mL). The resultant mixture was stirred at room temperature for 4 h. Excess diamine was removed under reduced pressure to afford a red precipitate. Ether (15 mL) was added, the mixture was ultrasonicated, and the resultant red precipitate was collected by filtration and washed with ether multiple times. After further washing with light petroleum and filtration, 2b was obtained as a pure red powder (0.178 g, 71.55%). Mp 103.8–106.6 °C. 1H-NMR (600 MHz, D2O, ppm) 3.41 (4H, t, J = 6.9 Hz), 2.72 (4H, t, J = 7.1 Hz), 1.78 (4H, q, J = 7.0 Hz). 13C-NMR (151 MHz, D2O, ppm) 159.66, 38.66, 38.02, 30.85. HRMS (ESI) m/z calculated for C8H19N8+, 227.1727; found 227.1719.
Synthesis of 3,6-bis(3-aminobutylamino)-1,2,4,5-tetrazine (2c). Tetrazine 1 (0.300 g, 1.1 mmol) was added to 1,4-butanediamine (3.5 mL). The resultant mixture was stirred at 35 °C for 6 h. Excess diamine was removed under reduced pressure to afford a red precipitate. Ether (15 mL) was added, the mixture was ultrasonicated, and the resultant red precipitate was collected by filtration and washed with ether multiple times. After further purification by heating circumfluence with light petroleum and filtration, 2c was obtained as a pure red powder (0.204 g, 72.85%). Mp 113.2–115.8 °C. 1H-NMR (600 MHz, D2O, ppm) 3.40 (4H, t, J = 6.9 Hz), 2.73 (4H, t, J = 7.2 Hz), 1.70–1.64 (4H, m), 1.61–1.54 (2H, m).13C-NMR (151 MHz, DMSO-d6, ppm) 160.84, 41.90, 41.21, 31.23, 26.65. HRMS (ESI) m/z calculated for C10H23N8+, 255.2040; found 255.2032.
Synthesis of compound 3a–3c
A mixture of 2a (0.200 g, 1 mmol) and NaHSO3 (0.2289 g, 2.2 mmol) in distilled water (10 mL) was stirred at room temperature for 20 min. The solvent was then removed under reduced pressure, methanol (10 mL) was added, and the mixture was filtered. The filtrate was evaporated under reduced pressure to afford 3a as a white powder. (0.2564 g, 71.00%). 1H-NMR (600 MHz, D2O, ppm) 3.56 (4H, t, J = 5.8 Hz), 3.22 (4H, t, J = 5.7 Hz). 13C-NMR (151 MHz, D2O, ppm) 152.16, 40.01, 38.57. HRMS (ESI) m/z calculated for C6H17N8O6S2+, 361.0707; found 361.0706.
3b and 3c were synthesized in a similar way to 3a.
3b. 1H-NMR (600 MHz, D2O, ppm) 3.22 (2H, t, J = 6.6 Hz), 3.11 (2H, t, J = 6.5 Hz), 2.95–2.91 (4H, m), 1.87–1.77 (4H, m). 13C-NMR (151 MHz, D2O, ppm) 154.08, 148.77, 38.57, 38.45, 37.12, 36.68, 26.57, 25.89. HRMS (ESI) m/z calculated for C8H19N8O6S2−, 387.0880; found 387.0874. (Fig. S1–S3†).
3c. 1H-NMR (600 MHz, D2O, ppm) 3.29 (2H, t, J = 6.5), 3.18 (2H, t, J = 6.6), 3.03 (4H, m, J = 14.6, 6.5 Hz), 1.74 (4H, m, J = 15.3, 7.6 Hz), 1.71–1.64 (4H, m). 13C-NMR (151 MHz, D2O, ppm) 149.19, 41.01, 40.85, 39.09, 38.97, 25.40, 24.65, 24.20, 23.86. HRMS (ESI) m/z calculated for C10H25N8O6S2+, 417.1325; found 417.1333. (Fig. S4–S6†).
Results and discussion
Probes 2a–2c were easily prepared in good yields (Scheme 1) and the structures were characterized by 1H and 13C NMR, and high-resolution mass spectrometry (HRMS) (Fig. S7–S15†).
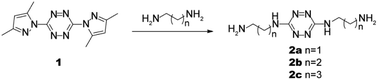 |
| Scheme 1 Design and synthesis of probes 2a–c. | |
UV-Vis absorption spectra of detecting HSO3−
UV-Vis titration spectra experiments for HSO3− in aqueous solution (HEPES buffer: 20 mM, pH7.0) were conducted using solutions containing different HSO3− concentrations. As shown in Fig. 1a, with increasing sulfite concentration, the absorbance at 458 nm decreased gradually. As expected, when the HSO3− concentration reached 2.0 equiv., the ΔAbs(A0 − A) reached a maximum and then plateaued (Fig. 1b). These experimental results coincided with the mechanistic analysis that a 1
:
2 adduct was generated. Furthermore, the orange solution changed to colorless, allowing the colorimetric detection of HSO3− by the naked eye.
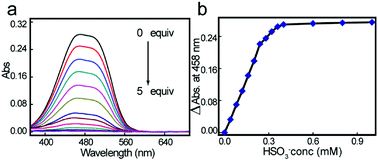 |
| Fig. 1 UV-Vis titration spectra (a) and ΔAbs (A0 − A) vs. bisulfite concentration plot (b) of 2a (2.0 × 10−4 M) toward 0 to 5 equiv of HSO3− in HEPES buffer (pH 7.0, 20 mM, 100% ultrapure water). | |
The selective response of probe 2a to HSO3−
The selectivity of 2a for HSO3− over other anions was investigated in aqueous solution at ambient temperature. As shown in Fig. 2a, free probe 2a showed a UV-Vis absorption centered at 458 nm. When 10 equiv. of 18 different representative anions (F−, Cl−, Br−, I−, OH−, CO32−, HCO3−, SO42−, HSO4−, SO32−, HSO3−, NO3−, NO2−, PO43−, HPO42−, H2PO4−, CH3COO−, and B4O72−) were added to the aqueous solution, only HSO3− induced a significant color change from orange to colorless (Fig. 2c), while others triggered only minor changes. Upon HSO3− addition, the absorption centered at 458 nm as diminished, meaning that colorimetric detection of HSO3− could be observed by the naked eye. Probes 2b and 2c showed similar sensing phenomena for HSO3− (Fig. S16 and S17†). But with the increasing length of chains, the selective efficiency is reduced obviously, response time is much longer and the reverse reactions between 2b and 3b, 2c and 3c happened easier, which leads to decreasing of the linearity of absorbance efficiency and concentration (Fig. S18 and S19†). And also the solubility in water decreases. Furthermore, competition experiments confirmed the good HSO3− selectivity. As shown in Fig. 2b, in the presence of the competitive species mentioned above, the signaling of 2a toward HSO3− was not affected. Additionally, 2a showed a negligible response to 16 different representative cations (Fig. S20†), suggesting that cations will not interfere with the probe 2a. These results implied that the novel probes (2a–2c) had excellent selectivity for HSO3− over other anions in aqueous environment.
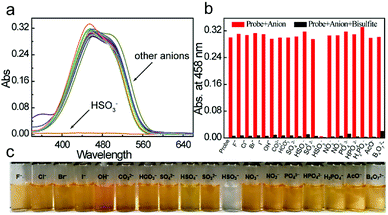 |
| Fig. 2 (a) UV-Vis spectra of probe 2a in the presence of common anions. [2a] = 2.0 × 10−4 M, [An−] = 2.0 × 10−3 M. λmax = 458 nm. (b) Competition experiments of the 2a–sulfite system and (c) color change photograph in the presence of coexisting anions. [2a] = 2.0 × 10−4 M. [Bisulfite] = 2.0 × 10−3 M. [An−] = 2.0 × 10−3 M. All spectra were measured in 100% ultrapure water. | |
pH dependent
We also explored the effect of pH on HSO3− determination. The maximum absorbance obtained at 458 nm for free probe 2a and probe 2a–HSO3− at different pH values is shown in Fig. 3. The absorbance of free 2a was constant at pH 5.5–9.5, indicating that it was stable. After adding 4.0 equiv. of HSO3−, the absorbance decreased, with a maximum value in the pH range 5.5–7.5. As both alkaline and acidic solutions would block HSO3− formation, we chose a neutral pH for further studies.
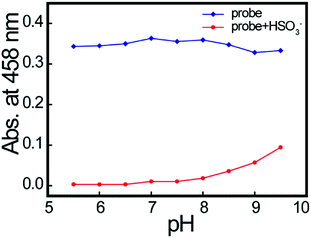 |
| Fig. 3 Effect of test solution pH on 2a (2.0 × 10−4 M) in the absence (blue line) or presence (red line) of bisulfite (4 equiv.). | |
The detection limit of probe 2a for HSO3−
According to concentration-dependent signaling behavior, the absorbance at 458 nm decreased linearly (0.9947) when the concentration of HSO3− ranged from 0 to 20 μM (Fig. 4a and b). The detection limit for HSO3− was estimated as 3.8 μM.
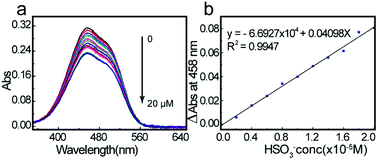 |
| Fig. 4 UV-Vis spectra (a) and ΔAbs (A0 − A) vs. bisulfite concentration plot for detection limit determination (b) of probe 2a in the presence of HSO3− at concentrations ranging from 0 to 20 μM. [2a] = 2.0 × 10−4 M. In HEPES buffer (pH 7.0, 20 mM, 100% ultrapure water). | |
Proposed mechanism
The change in chromogenic signaling showed that the π-conjugated structure of s-tetrazine was disrupted, with a new compound (3a) formed, which was obtained by performing the reaction on a large scale.
Clean 1H and 13C NMR, and HRMS, spectra of 3a were obtained (Fig. S21–S23†). As shown in Fig. 5a, the proton signals at 2.87 and 3.48 ppm were assigned to the methylene protons Ha (Hc) and Hb (Hd), respectively, in 2a. Upon addition of HSO3− to a D2O solution of 2a, all proton peaks shifted downfield. Resonance signals of Ha and Hc at around 2.87 ppm shifted to 3.22 ppm (
δ = 0.35 ppm) and resonance signals of Hb and Hd at about 3.48 ppm shifted to 3.56 ppm (
δ = 0.08 ppm). Furthermore, as shown in Fig. 5b, the chemical shifts of carbon atoms at the 3 and 6-positions shifted from 159.84 to 152.16 (δ = 7.67 ppm), while that of Ca (Cc) shifted from 39.46 to 38.57 (δ = 0.89 ppm) and Cb (Cd) shifted from 43.07 to 40.01 (δ = 3.06 ppm). All changes in the 1H NMR spectra were attributed to the formation of O⋯H hydrogen bonds between the sulfonic acid and methylene groups bonded to the carbon atoms in the tetrazine ring. In contrast, the collapse of the tetrazine conjugate system in compound 3a reduced the inductive effect of nitrogen atoms on tetrazine, leading to 13C NMR signals shifting upfield. The parent ion peak at m/z 361.0706 ([M + H]+) also strongly supported the formation of 3a.
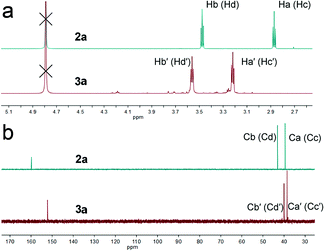 |
| Fig. 5 (a) 1H and (b) 13C NMR spectra of 2a in D2O and 2a–NaHSO3 in D2O. | |
When free radical inhibitor, 2,2,6,6-tetramethylpiperidine-1-oxyl (Tempo) was added in the reaction, the color changed from orange to colorless as normal. It manifests that the mechanism is not free radical type at first. Based on the experimental fact and spectral data, a mechanism was proposed (Scheme 2). Two tautomeric forms of HSO3− exist in dynamic equilibrium, one with a proton attached to sulfur (HSO3−) and the other protonated at oxygen (HOSO2−). The latter has two equivalent resonance structures. The carbon atoms in the tetrazine ring connected to three nitrogen atoms were electron deficient and easily attacked by nucleophile HSO3−. Formation of a sulfur–carbon bond leads to the collapse of the tetrazine conjugate system and formation of intermediate I. But the steric hindrance at 3,6-positions makes the I unstable to split into ·SO3H and free radicals II, followed by formation of more stable free radicals III. At last compound 3a was obtained by formation of C–S bond.
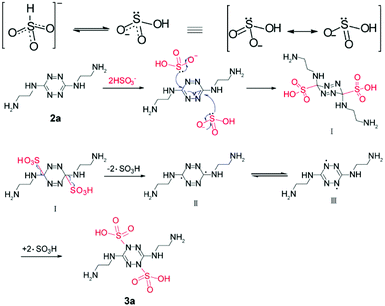 |
| Scheme 2 Proposed formation mechanism of 3a. | |
Detection of HSO3− in realistic food samples
Encouraged by these results, we also explored whether probe 2a shows a response for HSO3− in realistic food samples. We detected HSO3− in dry white wine, corn starch, canned fruit and jasmine tea drinks, which were purchased from a local supermarket. As shown in Table 1, 2a was able to determine HSO3− concentration in these samples with good recovery, ranging from 92.8% to 107.3%. The levels of HSO3− were calculated to be 38.56, 2.49, 3.21 and 2.13 mg kg−1, respectively. Moreover, titration method was also used to determine the concentration of HSO3− (Table S1†). However, the determination values in corn starch, canned fruit and jasmine tea drinks by iodine titration method were much higher than the values in this method, due to the reducing substances and only the results of dry white wine agreed well with our method. Thus, our method has advantages over traditional titration method for complex samples. Probe 2a could be well applied in the detection of HSO3− in food products.
Table 1 Determination of HSO3− in various food samples
Sample |
HSO3− level |
Added |
Found |
Recovery |
(μM) |
(μM) |
(μM) |
(%) |
Dry white wine |
14.27 |
3.00 |
16.02 |
92.8% |
Corn starch |
11.07 |
3.00 |
15.10 |
107.3% |
Canned fruit |
15.85 |
3.00 |
19.05 |
101.1% |
Jasmine tea drinks |
15.76 |
3.00 |
18.93 |
100.9% |
Conclusions
In summary, we have developed novel 3,6-bis-substituted-1,2,4,5-tetrazine-based probes (2a–2c). The probes exhibited a colorimetric response to HSO3− with excellent selectivity and good sensitivity in neutral aqueous solution at ambient temperature. Thus, probes 2a–2c possessed applicability for sensing bisulfite in actual food samples. A plausible mechanism was proposed, involving the nucleophilic reaction of a bisulfite anion with the tetrazine ring, followed by rearrangement of ·SO3H.
Conflicts of interest
There are no conflicts to declare.
Acknowledgements
This work was supported by the General Plan of Beijing Municipal Education Commission [grant number KM201710028007]; and the Beijing Natural Science Foundation [grant numbers 2173060].
References
- A. V. Leontiev and D. M. Rudkevich, J. Am. Chem. Soc., 2005, 127, 14126–14127 CrossRef CAS PubMed.
- S. Wang, X. Cao, T. Gao, X. Wang, H. Zou and W. Zeng, Mikrochim. Acta, 2018, 185, 218 CrossRef PubMed.
- L. Zhu, J. Xu, Z. Sun, B. Fu, C. Qin, L. Zeng and X. Hu, Chem. Commun., 2015, 51, 1154–1156 RSC.
- J. Wang, Y. Hao, H. Wang, S. Yang, H. Tian, B. Sun and Y. Liu, J. Agric. Food Chem., 2017, 65, 2883–2887 CrossRef CAS PubMed.
- T. Yu, G. Yin, T. Niu, P. Yin, H. Li, Y. Zhang, H. Chen, Y. Zeng and S. Yao, Talanta, 2018, 176, 1–7 CrossRef CAS PubMed.
- D. P. Li, Z. Y. Wang, X. J. Cao, J. Cui, X. Wang, H. Z. Cui, J. Y. Miao and B. X. Zhao, Chem. Commun., 2016, 52, 2760–2763 RSC.
- J. Xu, J. Pan, X. Jiang, C. Qin, L. Zeng, H. Zhang and J. F. Zhang, Biosens. Bioelectron., 2016, 77, 725–732 CrossRef CAS PubMed.
- X. Ma, C. X. Liu, Q. L. Shan, G. H. Wei, D. B. Wei and Y. G. Du, Sens. Actuators, B, 2013, 188, 1196–1200 CrossRef CAS.
- W. Zhang, T. Liu, F. Huo, P. Ning, X. Meng and C. Yin, Anal. Chem., 2017, 89, 8079–8083 CrossRef CAS PubMed.
- S. Samanta, P. Dey, A. Ramesh and G. Das, Chem. Commun., 2016, 52, 10381–10384 RSC.
- Y. Liu, K. Li, M. Y. Wu, Y. H. Liu, Y. M. Xie and X. Q. Yu, Chem. Commun., 2015, 51, 10236–10239 RSC.
- Y. Q. Sun, J. Liu, J. Zhang, T. Yang and W. Guo, Chem. Commun., 2013, 49, 2637–2639 RSC.
- M. G. Choi, J. Hwang, S. Eor and S. K. Chang, Org. Lett., 2010, 12, 5624–5627 CrossRef CAS PubMed.
- D. Lowinsohn and M. Bertotti, Food Addit. Contam., 2001, 18, 773–777 CrossRef CAS PubMed.
- S. S. Hassan, M. S. Hamza and A. H. Mohamed, Anal. Chim. Acta, 2006, 570, 232–239 CrossRef CAS PubMed.
- H. Li, X. Zhou, J. Fan, S. Long, J. Du, J. Wang and X. Peng, Sens. Actuators, B, 2018, 254, 709–718 CrossRef CAS.
- D. P. Li, Z. Y. Wang, H. Su, J. Y. Miao and B. X. Zhao, Chem. Commun., 2017, 53, 577–580 RSC.
- X. H. Cheng, H. Z. Jia, J. Feng, J. G. Qin and Z. Li, Sens. Actuators, B, 2013, 184, 274–280 CrossRef CAS.
- J. Luo, G. J. Song, X. J. Xing, S. L. Shen, Y. Q. Ge and X. Q. Cao, New J. Chem., 2017, 41, 3986–3990 RSC.
- S. Xu, R. Tang, Z. Wang, Y. Zhou and R. Yan, Spectrochim. Acta, Part A, 2015, 149, 208–215 CrossRef CAS PubMed.
- Y. Sun, Y. Li, X. Ma and L. Duan, RSC Adv., 2016, 6, 79830–79835 RSC.
- L. Wang, W. X. Li, W. J. Zhi, D. D. Ye, Y. Wang, L. Ni and X. Bao, Dyes Pigm., 2017, 147, 357–363 CrossRef CAS.
- Y. Q. Sun, P. Wang, J. Liu, J. Zhang and W. Guo, Analyst, 2012, 137, 3430–3433 RSC.
- Y. Sun, C. Zhong, R. Gong, H. Mu and E. Fu, J. Org. Chem., 2009, 74, 7943–7946 CrossRef CAS PubMed.
- Y. Zhao, Y. Li, Z. Qin, R. Jiang, H. Liu and Y. Li, Dalton Trans., 2012, 41, 13338–13342 RSC.
- Y. H. Gong, M. Fabien, M. R. Rachel, B. Sophie, L. Galmiche, J. Tang, P. Audebert and G. Clavier, Eur. J. Org. Chem., 2009, 2009, 6121–6128 CrossRef.
- C. Glidewell, P. Lightfoot, B. J. L. Royles and D. M. Smith, J. Chem. Soc., Perkin Trans. 2, 1997, 0, 1167–1174 RSC.
Footnote |
† Electronic supplementary information (ESI) available. See DOI: 10.1039/c8ra05682j |
|
This journal is © The Royal Society of Chemistry 2018 |
Click here to see how this site uses Cookies. View our privacy policy here.