DOI:
10.1039/C8RA06427J
(Paper)
RSC Adv., 2018,
8, 30098-30105
Preparation and investigation of novel SrCl2/DCMC-modified (via DOPA) decellularized arteries with excellent physicochemical properties and cytocompatibility for vascular scaffolds†
Received
30th July 2018
, Accepted 20th August 2018
First published on 24th August 2018
Abstract
A new method of fabricating vascular scaffolds was designed in this article by crosslinking the porcine arteries using dialdehyde carboxymethyl (DCMC) and further introducing the Sr element on the surface of modified arteries using DOPA. DCMC had been selected as an ideal crosslinking reagent for its excellent cytobiocompatibility and suitable chemical reactivity. Unfortunately, the endothelialization of biological vascular scaffolds fixed by DCMC was unsatisfactory. To overcome this deficiency, the Sr element was introduced onto arteries to improve the endothelialization of fixed arteries due to the Sr element being able to promote the expression of vascular endothelial growth factor (VEGF) being crucial for growth and proliferation of HUVECs. After modifying and crosslinking, their chemical structures, mechanical properties, stability, and cytocompatibility were examined. Our findings demonstrated that DCMC could improve the mechanical properties of animal-derived materials successfully and possess suitable biocompatibility compared with glutaraldehyde (GA). The Sr element can easily be introduced onto the surface of DCMC modified arteries by DOPA. Compared with purely DCMC-crosslinked ones, SrCl2/DCMC modification has no significant effect on the mechanical strength of fixed arteries, but a slight tendancy to improve the stability of fixed samples in D-Hanks solution. MTT assay and fluorescence tests implied that SrCl2/DCMC modification could effectively stimulate HUVECs' adhesion and proliferation, and thus promote the endothelialization process of fixed arteries. SrCl2/DCMC-modified arteries with excellent physicochemical properties and appealing HUVEC-cytocompatibility should be promising materials for fabricating vascular scaffolds.
1. Introduction
Cardiovascular diseases that result from genetics, tobacco, age, air pollution or bad life styles are one of the leading causes of death worldwide.1,2 In order to cure coronary artery diseases, cerebrovascular diseases and peripheral vascular disease, vascular prostheses are usually used to replace the damaged vessels because of the limited availability of autologous vessels. However, the quantity and quality of vascular prostheses can not meet the increasing demand for blood vessel substitutes in the clinic.3
Tissue engineering, with great prospects, is a suitable approach to address the need for vascular grafts.4 Before applying tissue-engineered vascular scaffolds as vascular grafts in vivo, rapid endothelialization of scaffolds needs to be completed in vitro, for the reason that endothelialization by HUVECs can provide a confluent, functional and antithrombogenic endothelial layer.5–7 An extracellular matrix (ECM) is very important for endothelialization. Therefore, fresh porcine arteries are chosen as base scaffold-materials to provide the important ECM where cells can attach, grow, proliferate and differentiate.8,9 However, deficiencies of living issues are still challenging. Amino groups existing on the surfaces of fresh arteries are responsible for immunological rejections or other biological reactions.10,11 Additionally, inflammatory responses caused by biodegradation of animal-derived materials require further investigation and development.12 To address this challenge, a promising crosslinking agent is strongly needed. Previous studies demonstrate that DCMC is a crosslinking agent with high efficiency due to its suitable chemical reactivity which enabled it easily react with functional groups like hydroxyl or amino groups existed on decellularized arteries under mild conditions to construct intact crosslinking structure.13,14 After fresh arteries fixation, intact reticular structure significantly improved the biomechanical properties and stability of animal-derived materials. Furthermore, cytotoxicity was decreased due to reduction of amino groups.15 Meanwhile, DCMC is a crosslinking agent with excellent biocompatible properties due to its macromolecule-structure hindering itself diffusing into cells to react with cellular components in contrast with traditional ones.16,17
Unfortunately, the effects of endothelialization on the surface of DCMC-fixed porcine arteries are not satisfactory, which might be attributed to the residual aldehydes on the surfaces and acute toxicity of polyaldehydes.14,18,19 And in our previous studies, we have proved that Sr element had the potential to promote angiogenesis for its stimulation of expression of vascular endothelial growth factor (VEGF).20–22 So, low-dose Sr is fixed onto DCMC-fixed surfaces of arteries for further modification to obtain results of rapid endothelialization.
DOPA is recognized as a useful and promising adhesion molecule for its easy operation, solvent-free, non-toxic and excellent adherent properties.23 Many scientists focused their attention on DOPA and successfully modified the surfaces of metals, polymers, semiconductors and ceramics to enhance interface compatibility between different materials based on the oxidative self-polymerization of DOPA.24 Besides, after the spontaneous reaction, PDOPA layer contained catechol and amine functional groups, which made it possible to form hydrogen and chemical bond for further modification.25 Therefore, it might be an effective adhesion molecule to introduce Sr element onto the inner surface of decellularized porcine arteries fixed by DCMC.
In this study, an effective and novel method for designing vascular scaffolds was created. Namely, SrCl2 solution was selected as reagent to introduce Sr onto DCMC-fixed surfaces of arteries considering commercial concern and high reactivity while the fresh porcine arteries and DCMC were applied as original materials and crosslinking reagents, respectively. The chemical structure, mechanical properties, stability, cytocompatibility, especially endothelialization, were tested and investigated in order to evaluate the chemical and biological properties of SrCl2/DCMC-modified arteries and provide a promising strategy to promote the endothelialization of DCMC-fixed arteries in clinical application.
2. Materials and methods
2.1 Preparation and assessment of dialdehyde carboxymethyl cellulose (DCMC)
To selectively oxidize CMC, the slightly modified method described by Wang et al. was applied.26,27 Briefly, dried CMC powder (approximately 5 g) was completely dissolved in 100 ml deionized water, and the initial pH was adjusted to 3.5 with 1 M sulphuric acid. Then CMC solution was mixed with 50 ml periodate solution (0.11 g mL−1) under continuously magnetic mechanical stirring in dark at room temperature for 4 h to obtain DCMC. 10 ml of ethylene glycol as termination agent was poured into the DCMC solution and the mixture was stirred in the dark at 37 °C for 4 h to decompose the remaining periodate. Subsequently, the product was purified by precipitation with anhydrous alcohol. Furthermore, the product was dissolved in deionized water and dialyzed using dialysis tube (MWCO, 3500) to make dialysate free from periodate. Finally, the solution was lyophilized to obtain purified product.
To confirm its chemical structure and successful oxidization, the CMC and DCMC were tested by Fourier transform infrared (FTIR) spectroscopy (Nicolet 560, USA).
2.2 Decellularization, crosslinking and SrCl2-modification of porcine arteries
Fresh porcine arteries, a kind of animal-derived materials, were applied as original materials. First, excess connective tissues attached on the external surface of arteries were trimmed thoroughly and clearly. Subsequently, the arteries were immersed in PBS solution contained 0.1% trypsin and 0.02% EDTA at 37 °C for 4 h, then for further digesting, arteries were soaked in hypotonic tris-solution using 1% Triton-100 for 48 h. In order to totally get rid of cellular components, RNaseA (0.02 mg ml−1) and DNasel (0.2 mg ml−1) solution were added. After decellularization, the arteries were crosslinked in 1% DCMC solution at 37 °C for 72 h with a constant shaking.28 Considering the high cytotoxicity of residual aldehyde groups, the crosslinked arteries were soaked in 0.1 M NaBH4/PBS solution at room temperature for 4 h with continuous agitating to deoxidize these groups and then the cytocompatibility of crosslinked arteries was improved. The decellularized arteries crosslinked by 0.625% glutaraldehyde (GA) solution were served as control.
Finally, the deoxidized arteries were immersed in 10 ml DOPA solution (2 mg ml−1) for 7 h with continuous shaking. And then, after rinsing with PBS for several times, the DOPA-modified arteries were soaked in SrCl2/Tris–HCl solution at room temperature for 24 h with continuous shaking to introduce Sr2+ to the arteries. (The optimal concentration of Sr2+ was evaluated and the data was shown in ESI.†) Meanwhile, the samples were tested by energy dispersive spectroscopy (EDS) to prove the successful introduction of Sr2+.
2.3 Light microscopy
After decellularizing and crosslinking, the specimens which had been fixed in 4% formaldehyde for 24 h were sectioned. Subsequently, the specimens which had been stained with hematoxylin and eosin (H & E) and Verhoeff iron hematoxylin were observed by light microscopy (Olympus Corporation, Japan) to reveal the total framework of the tissues.
2.4 Biomechanical property and SrCl2-modified-stability
Biomechanical tests were performed according to previously reported procedures.29,30 Additionally, the samples were also soaked in D-Hanks solution for periods of 0 d, 1 d, 3 d, 7 d, 15 d and 30 d respectively in order to evaluate the crosslinked stability. The tested-arteries, which had already been cut along collagen fibers' orientating and shaped with 40 mm in length and 4 mm in width along collagen fibers' orientating and presented a strip shape with 40 mm in length and 4 mm in width, were finally tested on an Instron material testing machine (Instron Co., USA) at a constant extension speed of 10 mm min−1 to reach a fracture point which was recorded as ultimate tensile strength. After the linear portion of the stress/strain curve was analyzed, the E-modulus of tested materials could be calculated.
2.5 In vitro enzymatic degradation
The resistant to enzymatic degradation of fresh, DCMC-fixed, SrCl2/DCMC-fixed, and GA-fixed tissues was evaluated according to a protocol reported and applied by Li et al. Briefly, various samples were digested with 250 U ml−1 collagenase/PBS solution at 37 °C under continuous shaking for 0.5 h, 1 h, 3 h, 6 h, 12 h and 24 h. After that the tested samples were transported into 10 mM EDTA solution and immersed for 10 minutes to terminate enzymatic degradation. To obtain the accurate data, before and after enzymatic degradation, all the samples were lyophilized and weighted. The degradation rate or weight loss percentage (ΔW) was finally calculated according to the formula:
where W0 represents the initial weight of each sample and Wt represents the weight of the corresponding sample after enzymatic degradation.31
2.6 Cytocompatibility of SrCl2/DCMC-modified tissues
To accurately exhibit the HUVECs-cytocompatibility of crosslinked tissues, the direct contact cytotoxic assay of those tested arteries were evaluated. Briefly, sterilized samples (5 mm × 5 mm) were placed at the bottom in a 24-well plate. Then HUVECs suspension at a concentration of 5 × 104 cells per well was seeded onto the surface of sample (500 μL culture medium per well) and incubated it at 37 °C in humidified 5% CO2 for predetermined time (1 d, 3 d, 5 d, and 7 d). The proliferated viable cells on specimens were determined using MTT essay at those days. During this incubation period, culture medium were changed every two days.
2.7 Morphologic observation of HUVECs on various tested arteries
To detect the viability and distribution of HUVECs growing on the surface of tested arteries, fluorescein diacetate (FDA) and propidium iodide (PI) were used to doubly stain HUVECs spreading on surfaces of various sterilized samples. The procedures were performed as reported.32 Briefly, samples were first stained with FDA for 5 min at 37 °C. It stained lived cells to generate green fluorescence due to its excellent permeability to both live and dead cells. Then samples were stained with PI for another 5 min at 37 °C, which permeate into dead cells and stained dead cells to generate red fluorescence. Then, these stained samples were observed with fluorescence microscope as quickly as possible considering the risk of fluorescent quenching.
2.8 Statistical analysis
SPSS (v19.0) was used to perform statistical analysis. Experimental data was presented as means ± standard deviation (SD). Results were analyzed by one-way ANOVA with a Student's t-test. Statistical significance was set at P < 0.05.
3. Result and discussion
3.1 Preparation and assessment of DCMC
To protect natural fibers structure of CMC, the method of slightly modified oxidization using NaIO4 was selected. Periodate oxidization has been investigated and utilized because C2–C3 bond of the 1,4-glucan unit could be specifically cleaved under mild conditions.33,34 DCMC was prepared under acidic conditions (pH 3.5) due to the highly specific reaction of CMC to dialdehyde without significant side reactions such as the further oxidization of aldehyde groups or the decomposition of NaIO4 under this condition. As shown in Fig. 1, the spectrum of DCMC showing the absorption bands at 1738.06 cm−1 was the most characteristic band of C–O vibrations in aldehyde groups, which proved the successful oxidization of CMC and forming of dialdehydes structures. Meanwhile, we also noticed the presentation of a sharp peak at 890.48 cm−1 in the spectrum of DCMC which could be attributed to the hemiacetal bonds forming between residual aldehyde groups and their neighbour hydroxyl groups.
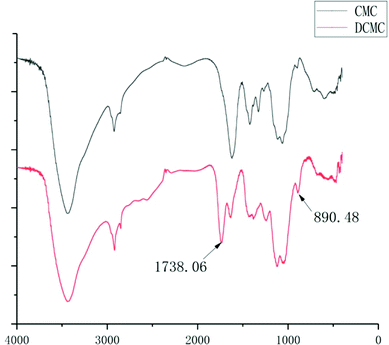 |
| Fig. 1 FTIR spectra for CMC and DCMC. | |
3.2 Decellularization, DCMC-crosslinking and SrCl2-modification of arteries
Cellular components may trigger many problems such as immunological recognition or other biological reactions. In this study, the aim of decellularization was to diminish cells and cellular components existed in vessel and thus to reduce the antigenicity of tissues. The decellularization can help graft-tissues avoid becoming immune target in vivo implant. The results demonstrated that, after decellularization procedure, there were no residual cellular components in the vessel-tissues. After fixation, aldehydes on DCMC could react with amino groups in arteries to form the cross-linking bridge structure (Schiff base). This, on the one hand could consume free aldehyde groups to eliminate its cytotoxicity, on the other hand could reduce the amount of free amino groups to reduce the antigenicity derived from free amino groups. However, the Schiff base reaction in water solution is reversible, therefore residual aldehydes and free amino groups would constantly appear in graft-tissues and influence the following application in cell seeding and in vivo implant. To address this problem, we treated the DCMC-crosslinked arteries with 0.1 M NaBH4/PBS solution to turn unstable Schiff base structure to stable imine bond and deoxidize aldehyde to stable hydroxyl respectively. This procedure is a crucial step for further improving crosslinking performance of DCMC and cytocompatibility of DCMC-crosslinked arteries.35,36 Subsequently, the DOPA-modified arteries were soaked in SrCl2/Tris–HCl solution, Sr2+ was adhered on the surface of tissues due to hydrogen bonds formation between Sr2+ and unique catechol functional groups of DOPA. The results of EDS shown in Fig. 2 proved that Sr element is successfully introduced onto the surface of DCMC-DOPA modified arteries due to excellent adhesion feature of DOPA.
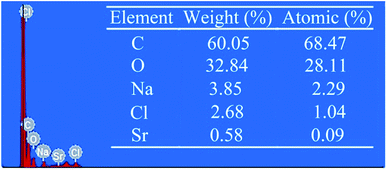 |
| Fig. 2 The EDS analysis of SrCl2/DCMC-fixed tissues. | |
3.3 Biomechanical properties and SrCl2/DCMC-modification-stability
The biomechanical properties of animal-derived materials are usually poor. Crosslinking these materials with crosslinking reagent may improve their biomechanical properties.37 The mechanical properties of the decellularized, the DCMC-crosslinked, and SrCl2/DCMC-fixed samples were tested. GA-fixed arteries were selected as the control group. The values in ultimate tensile stress and E-modulus for samples immersed in D-Hanks solution for 0 d, 1 d, 3 d, 7 d, 15 d and 30 d were shown in Fig. 3.
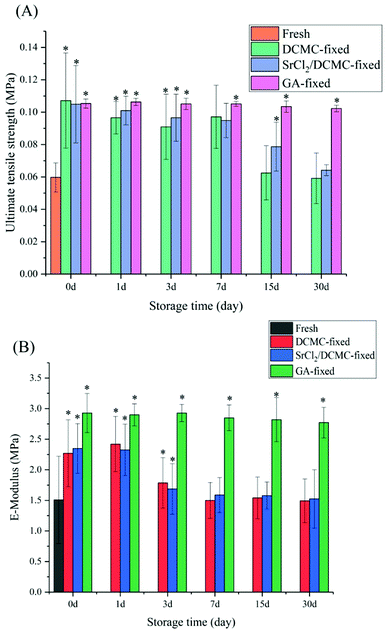 |
| Fig. 3 (A) Ultimate tensile strength and (B) E-modulus of fresh, DCMC-fixed, SrCl2/DCMC-fixed and GA-fixed arteries soaking in D-Hanks solution for different period (0 d, 1 d, 3 d, 7 d, 15 d and 30 d, respectively). | |
When all samples were not immersed in D-Hanks solution, the results of day 0 revealed that DCMC significantly improved the mechanical properties of arteries, and the biomechanical property of SrCl2/DCMC-fixed arteries were almost the same as the counterpart of DCMC-fixed arteries. The reason of the improvement of biomechanical properties of crosslinked tissues caused by DCMC was that multiple functional groups of DCMC could react with amino groups existed within arteries leading to the forming of intramolecular or intermolecular crosslinking structure. After further modification, the similarity of biomechanical properties of DCMC-fixed and SrCl2/DCMC-fixed samples proved that introduction of Sr element did not affect their mechanical properties. In summary, the strategy of introducing Sr element to the DCMC-fixed arteries was novel and effective, which endowed the materials with suitable mechanical properties and potentiality to improve endothelialization of graft-tissues by Sr element.
The data measured in 1 d, 3 d, 7 d, 15 d and 30 d showed the SrCl2/DCMC-modification-stability of samples in various groups. At first, the value of ultimate tensile stress and E-modulus all decreased slightly with increasing in degradation time. However, from the 15th to the 30th day, there is a marked drop in the biomechanical properties for DCMC-fixed and SrCl2/DCMC-fixed group due to the degradation of DCMC, but they were still superior than decellularized fresh samples. Moreover, we may draw a conclusion that Sr element can partially improve the stability of the modified-materials due to that the biomechanical properties of samples in SrCl2/DCMC-modified group was better than the DCMC-modified ones after soaking in D-Hanks solution for several days.
3.4 In vitro enzymatic degradation
Fig. 4 and 5 presents the photomicrograph of the total framework of decellularized and crosslinked specimens stained with H&E and Verhoeff iron hematoxylin before and after process of biodegradation (immersing in collagenase I solution for 12 h). After the enzymatic degradation process, the ultrastructure of decellularized fresh sample were chaotic and their natural structures were ruined entirely. The reason is that enzymes could easily transport into ECM and digest structures of collagen fibers without the effect of steric hindrance before crosslinking.11,38 In contrast, the microstructure of DCMC-fixed tissues remained their initial intactness and the array/orientation of fibers were still normal and regular due to their remarkable resistance to enzymatic hydrolytic degradation. The effect of steric hindrance resulted from intramolecularly or intermolecularly crosslinking structure caused by DCMC-fixation could hinder enzymatic penetration into fixed-tissues and improve the resistance to enzymatic hydrolytic degradation of samples. Moreover, the regularity and density of fibers after modification of Sr element were similar to morphology of DCMC-fixed tissues. This suggested introduction of Sr element did not influence the structure of extracellular matrices.
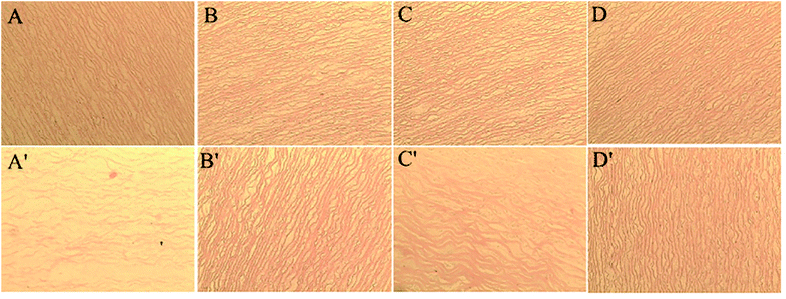 |
| Fig. 4 Photomicrographs (H&E staining, 200 × magnification) of the (A) fresh specimen, (B) DCMC-fixed specimen, (C) SrCl2/DCMC-fixed specimen and (D) GA-fixed specimen. (A′–D′) for corresponding samples after process of biodegradation. | |
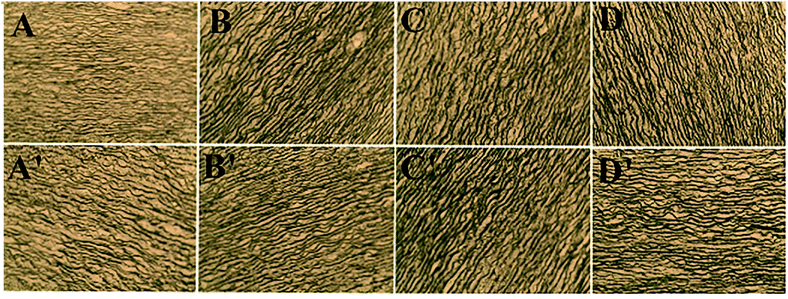 |
| Fig. 5 Photomicrographs (Verhoeff iron hematoxylin staining, 200 × magnification) of the (A) fresh specimen, (B) DCMC-fixed specimen, (C) SrCl2/DCMC-fixed specimen and (D) GA-fixed specimen. (A′–D′) for corresponding samples after process of biodegradation. | |
Fig. 6 showed the weight loss of all tested samples during degradation process at predetermined time in vitro. The weight loss of samples in GA-fixed group was very low during hydrolytic process, while the relative weight loss of samples in other experimental groups all increased significantly with the degradable time proceeding. As mentioned and certified, animal-derived biological tissues are easily degraded by various proteolytic enzymes and present high loss of weight.39 The weight loss of DCMC-fixed tissues was significantly lower than decellularized fresh samples, which suggested that DCMC was a useful crosslinking reagent to improve the mechanical property and stability of animal-derived biological tissues. The weight loss of DCMC-fixed tissues were almost the same as the SrCl2/DCMC-modified ones, which indicated the introduction of Sr element preserved the resistance to enzymatic degradation of samples.
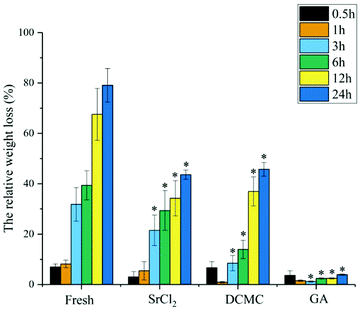 |
| Fig. 6 The relative weight loss of fresh, DCMC-fixed, SrCl2/DCMC-fixed and GA-fixed tissues in vitro at different time points. | |
3.5 Cytocompatibility and endothelialization in vitro
As mentioned above, HUVECs played a vital role in rapid endothelialization and preventing vascular-graft from formation of thrombus, so HUVECs were a kind of crucial cells in performance-evaluation of vascular scaffolds. Fig. 7 showed that the proliferation of HUVECs seeded on various tested samples.6
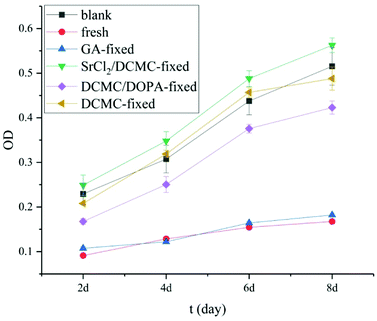 |
| Fig. 7 Proliferation of HUVECs seeded on the surface of various fixed arteries (2 d, 4 d, 6 d and 8 d, respectively). | |
As shown in Fig. 7, the OD values for the HUVECs cultured on the surfaces of the samples of GA-fixed group and fresh group decreased remarkably, which indicated their high cytotoxicity. They can not be used as materials to fabricate vascular scaffolds. In contrast, the OD values for DCMC-modified group were superior to the OD values of fresh and GA-fixed group at any selected time, which suggested that DCMC was an effective crosslinker with good cycocompatibility in the fixation of animal-derived tissues. As for the reason, we speculated that on the one hand the molecular weight of DCMC is much larger (than glutaraldehyde) enough to hinder itself diffusing into cells to react with cellular component; on the other hand, DCMC was derived from naturally occurring polysaccharide which was proved to be biocompatible. The OD values for DCMC/NaBH4 group were lower than blank control at the 2nd and 8th day, which might be attributed to toxicity of polyaldehydes and residual aldehyde groups due to degradation of DCMC, respectively.18,19 The results of samples in DCMC/DOPA-modified group indicated that the proliferation of cells on modified arteries was affected by the DOPA modification. DOPA showed a certain degree of inhibition in the proliferation of HUVECs.40 The OD values for SrCl2/DCMC-modified groups were the highest among all groups, even higher than that of blank control, which demonstrated that SrCl2/DCMC-modification could obviously promote the HUVECs-cycocompatibility of SrCl2/DCMC-modified arteries due to the low-dose Sr released continuously from this novel biomaterial. This result was encouraging for the rapid endothelialization of SrCl2/DCMC-modified arteries.
Fluorescent tests were conducted to detect the viability and distribution of HUVECs growing on the surface of tested arteries by double-staining method using FDA/PI. As seen in Fig. 8, the adhesion, growth and proliferation of HUVECs on fresh samples were very unsatisfied due to the immunological rejection. The number and viability of HUVECs grown on SrCl2/DCMC-modified arteries was better than all other groups. Meanwhile, the amount of dead cells on the surfaces of SrCl2/DCMC-modified arteries was the least among all tested ones. Additionally, the shape of HUVECs seeded on the surfaces of fresh and GA-fixed group was small and rounded, while the shape of cells cultured on the surface of SrCl2/DCMC-modified group was normal and regular, which proved that introduction of Sr element was a neat and promising method to meet the requirements of adhesion, growth and spreading of HUVECs. Our results supported that SrCl2/DCMC-modified arteries could be used as a potential vascular scaffold with promoting endothelialization due to its appealing HUVECs-cytocompatibility.
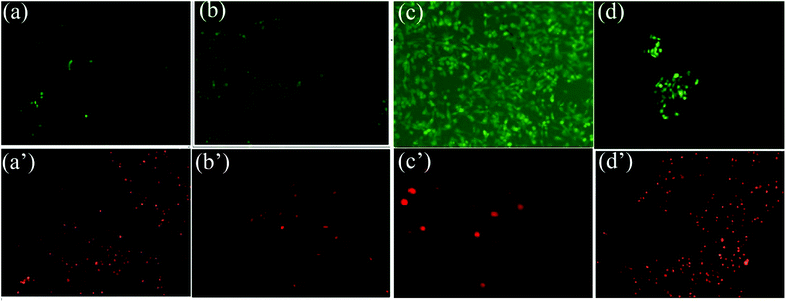 |
| Fig. 8 FDA/PI fluorescent photos of HUVECs cultured on the surface of various fixed samples. (a–d) FDA stained fluorescent photos: (a) fresh sample, (b) DCMC-fixed sample, (c) SrCl2/DCMC-fixed sample, (d) GA-fixed sample; (a′–d′) for corresponding samples of PI stained. | |
4. Conclusions
In summary, DCMC-fixed arteries with introduction of Sr element by DOPA were proposed to act as a promising vascular scaffold. Our findings demonstrated that: first, DCMC could improve mechanical property of animal-derived materials successfully and possess suitable biocompatibility compared with glutaraldehyde due to its polymeric structure and steric hindrance; second, Sr element can easily be introduced onto the surface of DCMC modified arteries by DOPA under mild condition. The introduction of Sr element did not affect their structure and mechanical properties; third, SrCl2/DCMC-modification could obviously promote the HUVECs-cycocompatibility of SrCl2/DCMC-modified arteries and SrCl2/DCMC-modified arteries possessed very good endothelial cell compatibility and had potential to promote endothelialization.
Conflicts of interest
There are no conflicts to declare.
Acknowledgements
The authors would like to thank the National Key Research and Development Program of China (No. 2016YFC1100900, No. 2016YFC1100901, No. 2016YFC1100903, No. 2016YFC1100904).
References
- S. Yusuf, S. Reddy, S. Ounpuu and S. Anand, Circulation, 2001, 104, 2746–2753 CrossRef PubMed.
- S. Yusuf, S. Reddy and S. Anand, Circulation, 2001, 104, 2855–2864 CrossRef PubMed.
- A. Khademhosseini and R. Langer, Nat. Protoc., 2016, 11, 1775–1781 CrossRef PubMed.
- R. Langer, Science, 1993, 260, 920–926 CrossRef PubMed.
- G. E. Davis and D. R. Senger, Circ. Res., 2005, 97, 1093 CrossRef PubMed.
- G. H. Gibbons, Am. J. Cardiol., 1997, 79, 3–8 CrossRef PubMed.
- M. R. Williamson, R. Black and C. Kielty, Biomaterials, 2006, 27, 3608–3616 CrossRef PubMed.
- M. S. Wietecha, W. L. Cerny and L. A. Dipietro, Mechanisms of Vessel Regression: Toward an Understanding of the Resolution of Angiogenesis, Springer Berlin Heidelberg, 2012 Search PubMed.
- X. Yang, J. Liu, Y. Xu, Z. Gu, Y. Xu, L. Li and X. Yu, RSC Adv., 2016, 6, 24527–24535 RSC.
- X. X. Yu, F. Liu, Y. T. Xu and C. X. Wan, J. Mater. Sci.: Mater. Med., 2010, 21, 777 CrossRef PubMed.
- Y. Xu, L. Li, H. Wang, X. Yu, Z. Gu, C. Huang and H. Peng, Carbohydr. Polym., 2013, 92, 448–454 CrossRef PubMed.
- F. Shahabipour, M. Banach, T. P. Johnston, M. Pirro and A. Sahebkar, Int. J. Cardiol., 2017, 228, 319–326 CrossRef PubMed.
- D. Li, Y. Ye, D. Li, X. Li and C. Mu, Carbohydr. Polym., 2016, 137, 508 CrossRef PubMed.
- X. Jiang, Z. Yang, Y. Peng, B. Han, Z. Li, X. Li and W. Liu, Carbohydr. Polym., 2016, 137, 632 CrossRef PubMed.
- C. Mu, J. Guo, X. Li, W. Lin and D. Li, Food Hydrocolloids, 2012, 27, 22–29 CrossRef.
- X. Wang, P. Tang, Y. Xu, X. Yang and X. Yu, Int. J. Biol. Macromol., 2016, 93, 1583–1590 CrossRef PubMed.
- X. Wang, Y. Wang, L. Li, Z. Gu and X. Yu, Carbohydr. Polym., 2015, 115, 54–61 CrossRef PubMed.
- X. Li, Y. Weng, X. Kong, B. Zhang, M. Li, K. Diao, Z. Zhang, X. Wang and H. Chen, J. Mater. Sci.: Mater. Med., 2012, 23, 2857–2865 CrossRef PubMed.
- B. Balakrishnan and A. Jayakrishnan, Biomaterials, 2005, 26, 3941–3951 CrossRef PubMed.
- H. Xie, Z. Gu, Y. He, J. Xu, C. Xu, L. Li and Q. Ye, J. Mater. Chem. B, 2018, 6, 2332–2339 RSC.
- X. Wang, Y. Wang, L. Li, Z. Gu, H. Xie and X. Yu, Ceram. Int., 2014, 40, 6999–7005 CrossRef.
- S. Li, L. Li, C. Guo, H. Qin and X. Yu, Int. J. Biol. Macromol., 2017, 104, 969–978 CrossRef PubMed.
- C. Luo, Z. Zou, B. Luo, W. Wen, H. Li, M. Liu and C. Zhou, Appl. Surf. Sci., 2016, 369, 82–91 CrossRef.
- X. Wang, Z. Gu, B. Jiang, L. Li and X. Yu, Biomater. Sci., 2016, 4, 678–688 RSC.
- C. C. Lin and S. J. Fu, Mater. Sci. Eng., C, 2016, 58, 254–263 CrossRef PubMed.
- X. Wang, S. Zhang and B. Ju, Tenside, Surfactants, Deterg., 2013, 50, 270–276 CrossRef.
- H. Li, B. Wu, C. Mu and W. Lin, Carbohydr. Polym., 2011, 84, 881–886 CrossRef.
- Y. Xu, L. Li, X. Yu, Z. Gu and X. Zhang, Carbohydr. Polym., 2012, 87, 1589–1595 CrossRef.
- C. Shuai, P. Feng, P. Wu, Y. Liu, X. Liu, D. Lai, C. Gao and S. Peng, Chem. Eng. J., 2017, 313, 487–497 CrossRef.
- P. Feng, P. Wu, C. Gao, Y. Yang, W. Guo, W. Yang and C. Shuai, Adv. Sci., 2018, 5, 1700817 CrossRef PubMed.
- Y. Xu, C. Huang, L. Li, X. Yu, X. Wang, H. Peng, Z. Gu and Y. Wang, Carbohydr. Polym., 2013, 95, 148–154 CrossRef PubMed.
- K. H. Jones and J. A. Senft, J. Histochem. Cytochem., 1985, 33, 77–79 CrossRef PubMed.
- I. M. N. Void and B. E. Christensen, Carbohydr. Res., 2005, 340, 679–684 CrossRef PubMed.
- T. Zheng, X. Yu and S. Pilla, Carbohydr. Polym., 2017, 157, 1333–1340 CrossRef PubMed.
- S. Hirano, M. Ishigami and Y. Ohe, J. Appl. Polym. Sci., 2010, 117, 682–690 CrossRef.
- C. Lee, S. H. Kim, S. H. Choi and Y. J. Kim, Eur. J. Cardiothorac. Surg., 2011, 39, 381–387 CrossRef PubMed.
- H. W. Sung, R. N. Huang, L. L. Huang, C. C. Tsai and C. T. Chiu, J. Biomed. Mater. Res., 2015, 42, 560–567 CrossRef.
- Z. Gu, X. Zhang, X. Yu, L. Li, Y. Xu and Y. Chen, Mater. Sci. Eng., C, 2011, 31, 1593–1601 CrossRef.
- H. J. Wei, H. C. Liang, M. H. Lee, Y. C. Huang, Y. Chang and H. W. Sung, Biomaterials, 2005, 26, 1905–1913 CrossRef PubMed.
- S. P. Berger, M. Hunger, B. A. Yard, P. Schnuelle and F. J. Van Der Woude, Kidney Int., 2000, 58, 2314–2319 CrossRef PubMed.
Footnote |
† Electronic supplementary information (ESI) available. See DOI: 10.1039/c8ra06427j |
|
This journal is © The Royal Society of Chemistry 2018 |
Click here to see how this site uses Cookies. View our privacy policy here.