DOI:
10.1039/C8RA06978F
(Review Article)
RSC Adv., 2018,
8, 36927-36938
Structural characteristics, analytical techniques and interactions with organic contaminants of dissolved organic matter derived from crop straw: a critical review
Received
20th August 2018
, Accepted 25th October 2018
First published on 1st November 2018
Abstract
Dissolved organic matter (DOM) represents one of the most mobile and reactive organic compounds in an ecosystem and plays an important role in the fate and transport of soil organic pollutants, nutrient cycling and more importantly global climate change. Advances in environment geochemistry in the past two decades have improved our knowledge about the genesis, composition, and structure of DOM, and its effect on the environment. Application of analytical technology, for example UV-visible spectroscopy (UV-Vis), Fourier transform infrared spectroscopy (FTIR), Nuclear magnetic resonance (NMR) spectroscopy, and three-dimensional fluorescence spectroscopy (3D-EEM) have resulted in these advances. At present, crop straw, as a part of energy development strategy, is mainly used for soil amendment, fodder, fertilizer and industrial materials. Moreover, the fermentation and decomposition of straw should be also promoted for ecological agriculture. However, few studies have focused on the structural properties of DOM derived from crop straw in farmland soil. In this article, DOM derived from crop straw, which is abbreviated to “CDOM”, presents active physicochemical properties that can affect the migration and bioavailability of organic contaminants (OCs) in terrestrial ecosystems. The objectives of this review paper are: (i) to discuss the structural characteristics, analytical techniques and interactions between CDOM and OCs in farmland soil; (ii) to present a critical analysis of the impact of CDOM on the physicochemical transformation and transport of OCs in farmland soils; (iii) to provide the perspectives in future research. Therefore, the findings obtained from this study can be utilized to evaluate the relations of interactions between CDOM and OCs in agricultural soils, in order to support some suggestions for future development in agricultural waste recycling, buffering of organic pollution, and the effect on the global carbon cycle.
1 Introduction
With economic and social development, organic pollution in soils has become a common environmental issue.1 The organic contaminants (OCs), originating from sewage irrigation, animal manure, pesticide application, atmospheric deposition and waste dumping, have entered into farmland soils, and participated in different natural geochemical and biological processes.2–4 The source, composition and structure of dissolved organic matter (DOM) with the molecular weight distributed widely are very complex.5 DOM is an organic polymer mixture, and widely exists in soil, sediment, aerosol, and natural water bodies.6 DOM is conventionally defined as any organic material that passes through a given filter (0.45 μm), termed “dissolved” and the organic material that is retained on the filters is termed “particulate”.7,8 Among the organic substances in DOM, which can pass through filters, are fulvic acid, humic acid, carbohydrates, sugars, amino acids, proteins, lipids, organic acids, phenols, alcohols, acetylated amino sugars. Most of its composition is bioavailable and can be easily absorbed and utilized by soil microorganisms.9,10 DOM plays dominant role in the global circulation, speciation, toxicity, transfer and transformation of OCs.11,12 Significantly, DOM derived from crop straw, which is abbreviated to “CDOM”, presents active physicochemical properties that can affect the migration of OCs and bioavailability of OCs in terrestrial ecosystems.13
Through various interactions (van der Waals forces, hydrophobic interactions, and electron donor–acceptor), DOM binds OCs and affects the bioavailability of freely dissolved and bound OCs. DOM-sorbed pollutants normally have lower bioavailability compared to the pollutants freely dissolved in aqueous phase. Published research documented the importance of DOM in sorbing OCs and inhibiting their bioavailability. The inhibiting of bioavailability can result in a decrease biodegradation of OCs.14 Meanwhile, previous studies have shown that contaminant toxicity to target organisms is altered by the presence of DOM. Contaminants can bind to DOM and this may alter the bioavailability and subsequent toxicity of the contaminants.15 Perminova et al.16 found the toxicity of PAHs to Daphnia magna was alleviated by humic acids (HA) and fulvic acids (FA). Yang et al.17 also proved that phenanthrene would be adsorbed preferentially by aromatic carbon rich lignin than paraffinic carbon rich wax, thus lowering its bioavailability. However, several reports showed that the presence of DOM would also increase the bioavailability of OCs.18 Lin et al.19 found that DOM promoted the bioavailability of pyrene when the freely dissolved concentration of pyrene was kept constant. Also, as suggested by Cai et al.,20 DOM enhanced the bioavailability of phenanthrene by facilitating the microbial accessed to DOM-phenanthrene complex. The enhancement of polyferric aluminum sulfate (PFAS) bioaccumulation by Daphnia magna after addition of DOM at a low level was also found by Xia et al.21 Therefore, DOM is a key component of the global carbon cycle and it is considered as the most active chemical group in terrestrial and aquatic ecosystems with its contribution of natural ligands and adsorption carriers in the environment.7,22
The content of DOM (less than 0.5% of soils organic carbon (SOC)) in soil was generally relatively low, but it was one of the most active components in the soil ecological environment, it also may most rapidly reflect changes in the condition of a soil.23 As the organic fertilizer application and direct straw returning are widely used measures for farmland soil improvement in agricultural country, a mass of exogenous DOM are being taken into farmland leading to an increase in DOM content of farmland soil.24,25 According to relevant report, the content of DOM always in a dynamic process. Kalbitz et al.26 contend that content of DOM was effected by four factors: (i) properties of soil solid phases (ii) environmental factors (iii) chemical properties of soil solutions (iv) decomposition and transformation of DOM. However, the content of DOM in soil differs in one soil type from another. Also, the type of straws and pretreatment methods make difference. Nowadays, more and more OCs is being poured into farmland soil due to agricultural activities such as fertilization, sewage irrigation, and land application of sludge.27,28 DOM has a number of important ecological functions. For instance, DOM binds to a variety of heterogeneous organic substances or OCs to modify their behaviors in soils.29,30 Also, DOM mitigated the effect of OCs by reducing their free concentrations and consequently accumulation by organisms.31,32 Thus, DOM plays important roles in many chemical and biological processes in soils.33
To date, most studies have focused on the influence of DOM on toxicity of hydrophobic organic chemicals in aquatic organisms.34 However, more researches need to be done on the release of DOM from the decomposition process of crop straw and the biochemical effect of CDOM on OCs availability. The objectives of this review paper are: (1) to discuss the structural characteristics, analytical techniques and interactions between CDOM and OCs in soil; (2) to present a critical analysis of the impact of CDOM on the physicochemical transformation and transport of OCs in soils; (3) to evaluate the changes in toxicity of OCs caused by CDOM; (4) to summarize the research achievements at present and to provide the perspectives in future research. Ultimately, it improves our understanding of OCs transfer within the farmland soil system, and provide information for strategy that reduces the environmental risks of the OCs to crop production.
2 Sources and structure of DOM in farmland soil
2.1 Sources of DOM in farmland soil
It is generally known that DOM is a mixture composed of various compounds, and each component is greatly different in organic carbon content, molecular weight distribution, and functional groups.35 The structures and chemical reactivities of DOM can vary in space and time, depending in large part on the source materials and subsequent degradation, transformation, and/or mixing processes.36 External sources of DOM in farmland soil are mostly supplied from the surrounding soils which are covered with a wide array of organic source materials. These terrestrial sources are likely to contain plant debris, detritus, living organisms, algae and straw.37 Moreover, the different sources of DOM inevitably affect its distribution characteristics and structural properties, degradation process and interaction with soil components, which ultimately affected the biogeochemical processes of DOM in farmland soil.38–40 Similarly, in the latest studies, Martin et al.41 studied the sorption affinities of 4 pharmaceuticals applied in solute mixtures to soils taken from different horizons of 3 soil types. This experiment revealed that the content of organic matter would be different for various types of soil. Moreover, the sorption of OCs in soil may be affected by the different of DOM content and characteristic. Bi et al.42 reported that the DOM derived from different sources enhanced the uptake of OCs by soil compared with the control treatment. In the case of the OCs, sorption affinity of compounds decreased with soil depth, i.e. decreased with soil organic matter content. The forms of contaminants may also be affected. Badejo et al.43 found that lead isotopic composition (Pb-206/Pb-207 ratios) depends on soil organic matter content either fresh/poorly humified or humified and effect its degradation process. Thus, for more information of DOM contents, it can take correct precautions and measures to contaminants.
At present, there are vast numbers of crop straws in China, which have many types and are widely distributed.44 Research into the utilization of crop straws has increased in recent years due to its renewability, widespread availability, and its potential value that can be added by the generation of straws-based products.45 Climatically, the Western Sichuan Plain is a subtropical humid monsoon zone.46 The plain has been continuously managed, reconstructed and expanded, the land utilization rate has reached more than 60%, and has become the main agricultural production base in China.47 The Western Sichuan Plain is the main producing area of wheat-rice, canola-rice two cropping, from the principle of minimum dynamical soil, reducing soil exposed on the basis of conservation tillage and achieving the goal of covering the surface with the rice and wheat.48 In present, a series of reviews were conducted to introduce the dynamic properties of CDOM decomposition in farmland soils. Recent studies have shown that DOM from three types of straw (barley, rice, and wheat) and natural organic matter (NOM) isolates were investigated in terms of their photochemical properties and reactive oxygen species (ROS) generating abilities. Results demonstrated that the DOM derived from the aeration decomposition of barley straw yielded the best formation efficiencies of hydrogen peroxide (H2O2) and hydroxyl radicals (·OH) under solar-simulated irradiation in all organic matter samples. So, the characteristics of DOM released from the decomposing process of different straws may also differs.49 Although DOM are from the same source, the results of the present study demonstrate that chemical characteristics of fresh DOM and degraded DOM which both were extracted from the straw of maize, they are greatly diverse in components, functional group species, molecular weight distribution, etc.50,51 Hence, understanding the characteristics and role of the CDOM is important for facilitating the potential application in agricultural environments.45
2.2 Structure of CDOM in farmland soil
CDOM is one of the most active compositions in soils, which can affect the transport and decay of soil pollutants, and the availability of soil nutrients (C, N, P, S, etc.).52 CDOM comprises a huge variety of organic substances including chromatographically unidentifiable humic substances and chromatographically identifiable biomolecules such as carbohydrates, amino acids, fatty acids, etc.53,54 Humic substances (HS), such as humic and fulvic acids, are conventionally defined as heterogeneous organic materials extracted at alkaline pH and, according to the recent concept regarding soil organic matter (SOM), represent aggregates of small molecules with common properties, such as high aromaticity reflecting an excess of phenol groups.55 Non-humic substances mainly derive from animals, plants, microorganisms and their residues, in the forms of sugars, amino acids, proteins, lignin and organic acids.56 Humic acids are the major extractable component of soil humic substances and are the primary organic compounds of soil. The molecular size of humic acids range from approximately 10
000 Da to 100
000 Da, about 35% of the humic acid molecules are aromatic, while the remaining components are in the form of aliphatic molecules. Fulvic acids are a mixture of weak aliphatic and aromatic organic acids, the size of fulvic acids are smaller than humic acids, with the molecular weights range from approximately 1000 Da to 10
000 Da.57
As early as 1976, the researchers took the lead in adopting XAD resin tandem anion and cation exchange resins to divide DOM into six components: hydrophobic base (HOB), hydrophobic neutrals (HON), and hydrophobic acid (HOA), hydrophilic acid (HIA), hydrophilic base (HIB), and hydrophilic neutrals (HIN).58–60 This report intends to enrich the hydrophobic, neutral, and hydrophilic components of CDOM by XAD-8/XAD-4 resin, most traditionally based on the hydrophilicity and acidity of CDOM components. According to one study the hydrophobic fraction consists of soluble degradation products of lignin; it is enriched in structural ortho-hydroxybenzene fragments, which ensure its selective sorption and strong retention, but the hydrophilic fraction composes the major portion of labile DOM in soils.61 Thus, soil CDOM containing a great deal of lower molecular weight fractions or hydrophilic fractions is more mobile and readily biodegradable, compared to CDOM with larger molecular weight or hydrophobic fractions.
It is widely recognized that the humic substances (HS) in soils and sediments is the principal factor controlling sorption of organic compounds. Moreover, it has been established that the organic carbon-normalized partition coefficient (Koc) is a critical factor in determining hydrophobic organic chemical distributions in most systems. The variation in quality of the HS strongly affects the transport of toxic organics (Koc).62 For example, Chefetz et al.63 showed that the polarity and aromatic component of the natural organic matter influence the binding of polycyclic aromatic hydrocarbons (PAHs). The sorption of naphthalene increased with increasing aromaticity and decreasing polarity of soil organic matter. A strong correlation was observed between Koc for pyrene and the molecular weights, molar absorptivities at 280 nm, an aromaticity of the HS. Panagiotopoulos et al.64 investigated the relations between log
Koc and absorptivity, H/O ratio, and the concentration of phenolic group of the dissolved organic matter surrogates. The (bio)degradation of soil DOM does not necessarily rely upon the molecular weights distribution. Previous studies on DOM from forest soil organic horizons showed that both the highest (>100
000) and lowest (<1000) molecular weight organic fractions can be (bio)degradable. Thus, the majority of DOM compounds in soil solution are usually shifted towards lower molecular weight (<100
000 size range) as a result of the degradation of initial litter organic matter under oxidised conditions. In soils, several organic compounds formed during the decomposition of SOM or lysis of microbial cells can sorb onto functional sites of mineral surfaces (Fe(III) and Mn(IV) oxyhydroxides and clays) or coprecipitate with newly formed secondary minerals. In particular, DOM enriched with aromatic moieties or hydrophobic carbon moieties (i.e. alkyl chains) was reported to be preferentially bound to mineral surfaces.65 Thus, CDOM can not only serve as an important source of energy for microbial growth and reproduction, but can also regulate the environmental behavior of pollutants in soil, such as their solubility, migration and transformations.42,66
2.3 Effecting factors of the content and nature of CDOM
The change of DOM contents is affected by many factors, for example, anthropogenic and natural factors and so on. Especially DOM is a balanced dynamically with complex process in farmland soil. DOC would be affected by straw mulching, organic and inorganic fertilizer, etc. Chang et al.67 had been demonstrated in their experiments. In moisture soil, green manures and soils were sampled in situ at the ploughed stage of green manures. A 56 day laboratory incubation experiment was conducted to simulate the dynamic changes of soil DOM influenced by the decomposition of green manures, the composition and ultraviolet-visible spectrum parameters of soil DOM were investigated at different incubation stages.68,69 Results showed that green manures could increase the dissolved organic carbon (DOC), the total organic acids (TOAs) and total carbohydrate (TCs) contents, and all treatments were reached a peak on the 1st day and decreased later. Meanwhile, green manures could increase the contents of DOM and its aromaticity, hydrophobic percentage, humification degree and average molecular weight, and could be increased and the stability of DOM could be enhanced accordingly.67 The content of DOM also can be affected by different pretreatment methods. Chen et al.70 understand as to how pre-treatment of a soil will affect the characteristics of DOM, since this fraction may be strongly influenced by a soil's water content. The effect of two different pre-treatments on DOM from the A-horizons of a large variety of ecosystems and regions were compared. And it analyses quantity and spectroscopic properties of soil DOM. The results show that DOM extracted from soils is not comparable. So, the content of DOM always in a dynamic process. Soil moisture is another important factor affecting on DOM. there were a lot of discussions the change of DOM contents in wetting-drying soil.71 All the above results are in correspondence with theoretic analysis that DOM contents significantly increased with greater soil moisture. Some researchers show that the concentration of DOC in soil solution was significantly increased at the initial stage of flooding. The depth of soil can also effect content of CDOM. On the vertical direction, CDOM showed a decreasing trend with the increase of soil depth.72 In addition, it contents changed with the seasons, in order of spring > summer > autumn > winter. The CDOM contents in horizontal direction, the moisture content, pH, and soil microorganism affected the humidification and mineralization processes of organic matter, which further affected the variation of DOM structure.73 The structure of CDOM affect not only the interaction of CDOM and OCs directly but also the features of soil surface, which may eventually result in the alteration of OCs activity in soil.
3 Analytical techniques on the properties of CDOM
In the past two decades, analytical tools for determining DOM chemistry have significantly advanced from bulk chemical characterization by elemental analysis to simple molecular-level approaches represented by e.g. UV-visible spectroscopy (UV-Vis), three-dimensional fluorescence spectroscopy (3D-EEM) and Fourier transform infrared spectroscopy (FTIR), as shown in Fig. 1.74–78 These rapid and relatively inexpensive techniques are widely used to obtain information on sources, composition and sometimes reactivity of DOM, so it is in CDOM. Also, mass spectrometry (MS) and nuclear magnetic resonance (NMR) spectroscopy can be used to provide useful information on DOM chemistry (Table 1).79–82
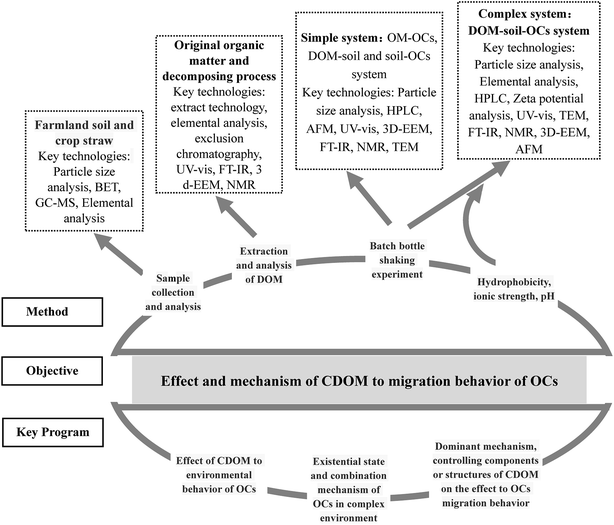 |
| Fig. 1 Technology roadmap of interactions with organic contaminants of DOM derived from crop straw. | |
Table 1 Analytical techniques for structural characteristics of DOM derived from crop straw
Analytical techniques |
Function |
Advantage |
Elemental analysis |
Element composition and content of CHNOS |
Universality and the easy to operate |
UV-Vis |
Functional groups, chemical bonds |
Don't need special handling and ease of operation |
FT-IR spectroscopies |
The form of functional groups |
High sensitivity and only needs few sample |
Three-dimensional fluorescence spectroscopy (3D-EEM) |
Fluorescence characteristic and source and structure of DOM |
High sensitivity and do not destroy samples |
Nuclear magnetic resonance (NMR) |
Element composition of C/H and provide important fingerprint information for the characterization of DOM structures |
Higher accuracy and sensitivity |
3.1 Elemental analysis
Element analysis is one of the most common methods to record DOM structures. There are some researches who have reported a characterization study of soil-derived DOM across the Three Gorges Reservoir (TGR, China), using elemental and optical analysis, infrared spectroscopy.83 With elemental analysis, basic information on the nature of DOM also can be obtained through simple molar ratios.84 For instance, C/H can indicate the degree of unsaturation and aromaticity of DOM, while O/C or (N + O)/C indicates the polarity of the DOM.85 The results show that the soil DOM from the TGR area is a mixture of “allochthonous” (i.e., plant-derived/terrigenous) and “autochthonous” (i.e., microbial) origins. The terrigenous DOM is composed primarily of phenolic and aliphatic structures from lignin and aliphatic biopolymers (i.e. cutin, suberin), respectively.83 Recent research shows that fractionation of DOM from farmland soils was conducted by using XAD-8 resin into HOA, and HON, and their structural characteristics were studied by means of elemental composition, FTIR and 1H-NMR spectroscopy.86,87 Separated by this technique, the major portion of the DOM mainly characterized HON, while the HOA fraction contained a large amount of carboxyl groups but fewer aromatic groups with a higher quantity of carbohydrates compared to that of fulvic acid (FA).88
3.2 UV-Vis and FT-IR spectroscopies
UV-Vis spectra reflect the characteristics of DOM in terms of aromatic compounds and conjugated systems. Combining DOM characterized by UV-Vis spectroscopic indices and elemental analysis is useful to distinguish DOM straw sources.89 Single spectral technology cannot comprehensively reveal structural features and sources of DOM, therefore, in some studies UV-Vis and the 3D-EEM spectroscopy were applied to investigate the structure and origin of DOM from soils.90 It can serve to provide a theoretical basis for the further study of the DOM's effect on the migration and transformation of OCs.
FT-IR spectroscopies can contribute to DOM along the soil depth profile to better understand elemental compositions.91 Though FT-IR, UV-Vis and fluorescence spectra results suggested that DOM from the upper horizons was enriched with aromatic and humic structures while that was rich in aliphatic carbon, which supported the obtained by spectroscopic characteristics of DOM from the lower horizons.42 Choi et al.92 investigated DOM in moist acidic tussock tundra to better understand elemental compositions, in this study, the soil extracts were analyzed using a 15 tesla FT-IR spectrometer in positive and negative ion modes via electrospray ionization. The results of this analysis revealed that the deeper organic soil exhibits less CHON class and more aromatic class compounds compared to the surface organic soils, thus implying that the deeper soil has decomposed more and consequently possessed the recalcitrant materials.93 Recent research, the fractional composition of DOM and the chemical nature of humic and fulvic acids were studied in lysimetric waters from forest soils of different altitudinal zones in the Sikhote Alin Range.94,95 The elemental composition, infrared absorption spectra, concentrations of acid functional groups of humic and fulvic acids were determined. The soils of high-mountain zones had stronger acidic properties of humic and fulvic acids in comparison with the soils of low-mountain zones.96 Moreover, infrared spectroscopy can provide functional information of DOM, and inform on the composition of each functional group in the dynamic change of DOM.
3.3 Three-dimensional fluorescence spectroscopy (3D-EEM)
3D-EEM can show the distribution of DOM in unsaturated structure and different types of fluorescent materials humic and fulvic acids fluorescence are mainly attributed to carboxyl, phenolic hydroxyl or carbonyl groups.97 EEM spectroscopy coupled with parallel factor (PARAFAC) and principal components analysis (PCA) was a useful method to characterize fluorescent components of DOM, by specific indices, such as the humification index (HIX). For instance, a study of organic materials and their sources in upstream and downstream waters of dissolved organic carbon (DOC) was performed by EEM spectroscopy, PARAFAC analysis and photo-microbial experiments.98,99 The upstream DOM was mostly composed of one component that had a fulvic acid-like substance whereas downstream DOM was composed of two components with mixtures of tryptophan-like and fulvic acid-like substances.100
Also 3D-EEM can be used to analyze the interaction between DOM and OCs. By using the 3D-EEM technology, together with the fluorescence regional integration (FRI) quantitative method, the long-term effects of pesticide residues under low concentration of natural DOM were analyzed. These results suggest that the distribution of different types of pesticide residue in the soil was influenced by different components at different levels of significance. Moreover, the humification degree of soil organic matter was an effect of DOM on the pesticide residues in the soil.101
3.4 Nuclear magnetic resonance (NMR)
13C-NMR spectroscopy can provide important fingerprint information for the characterization of DOM structures, such as the relative content of various types of carbon (aliphatic, aromatic, carboxyl, carbonyl, amine, etc.).7,102 Researchers have studied the direct conversion of DOM from the aromatic compounds and unsaturated structures to carboxylation and hydroxylation products by using a flow-based design NMR spectroscopy and direct sunlight interface.103 The phenols, carbonyls and carboxylic acids can enhance the interaction of DOM and organic contaminants, which further regulated the migration and transfer processes of contaminants in farmland soils.104 For instance, a recent study was conducted to understand the influence of dibutyl phthalate (DBP) adsorption kinetics and isotherms in soils under different concentrations of extraneous DOM, by using FTIR and NMR. The NMR indicated that the intra-molecular and intermolecular hydrogen bond interactions of carboxylic acids, aromatic CC and CO in amides were involved in DBP adsorption in soils. Therefore, the addition of DOM may increase adsorption of DBP in soils.101 Also, NMR spectroscopy can be used to evaluate the aromatic compounds and their degradation products in samples influenced by OCs and its biodegradation.105 It can make us to enable a comprehensive level understanding of the DOM with respect to in composition and structure. The role of natural DOM as potential cosubstrate and detoxification reactant may improve future remediation strategies.
Each of the above indicators, confirm and complement each other. The integration of multivariate information can be more useful for accurately understanding the nature of crop straw and its decomposition process DOM. This can not only correlate the changes in the composition of soil organic matter, but help to understand the source of straw provides the basis for the mechanism of interaction between DOM and OCs.
4 Interaction between CDOM-soil-OCs
4.1 Benefits of DOM on soils
The availability of crop straw in agriculture is very important for the sustainability of biomass supply. Returning crop straws to fields can improve the soil quality by increasing the soil CDOM content, enhancing the microbial activities as well as their enzymes production, and improving the soil texture.24 Due to the restitution of crop residues and the application of organic fertilizer during the cultivation process, the exogenous CDOM can augment its concentrations in agricultural soil.106–108 SOM content and composition affect both soil structure and adsorption properties; so, water retention may be affected by changes in soil organic matter.107 Thus, CDOM is widespread in natural environments where it has an important role in determining the balance and long-term accumulation of element content (carbon, nitrogen, and phosphorus) in soil.109 Incorporation of organic matter had been put into practice as soil amendment in protect the soil from solar energy to reduce evaporation and aid in moisture retention to decrease soil water loss. Several studies conclude that soil moisture, rather than temperature, has the most significant influence on soil organic matter. Moreover, soil organic matter had been applied as an important factor to predict soil water retention characteristics. Rusan et al.110 were confirmed the function organic matter incorporation into soil continuously in improving soil water retention and plant growth environment. Conversely, soil capacity as well as the crop productivity directly depends on soil degradation and pollution, which determine effecting of soil organic matter and biological diversity.
4.2 Interaction mechanism of CDOM-soil-OCs
At present, some researches on the interactions of DOM-OCs are mainly concentrated in the environmental medium of humic substances DOM as soil, lakes, rivers, wastewater, etc.111–114 With regard to the mechanism of DOM binding between OCs in farmland soil, DOM is considered to be one of the most important components due to its active physicochemical properties. There have been many theories and empirical studies in this field.115 So far there are many mechanisms for the combination of DOM and OCs, and the same would be true of CDOM. It mainly includes hydrogen bonding, ligand exchange, hydrophobic particle distribution, covalent bonding, chelation, π bond interaction, and electron transfer.116–118 In the process of combining OCs with CDOM components and a variety of mechanisms coexist, often associated with the soil environment and the property of organic compounds.119
The contents of organic matter in different crops were different so that the contribution of the decomposition process to CDOM of soil must be different.120 Wei et al.121 studied that crop straw decomposition process can be divided into three stages: (1) leaching release where the soluble components in the crop straws are rapidly leached into the soil by irrigation and rain erosion; (2) the degradation of N and P materials is easy to be degraded; (3) organic matter degradation with mainly polysaccharides, lignin, dense kink of lipids and so on as which are the main released matters, also the persistent cellulose, hemicellulose and macromolecular protein were decomposed. In the case of crop straw after leaching, the elemental distribution and chemical properties of the CDOM released by the decay process are still in a dynamic state.122 As the properties of soil component are closely related to the environmental behavior of OCs, the CDOM will have an important effect on the migration behavior of OCs in farmland soil, as shown in Fig. 2(a).
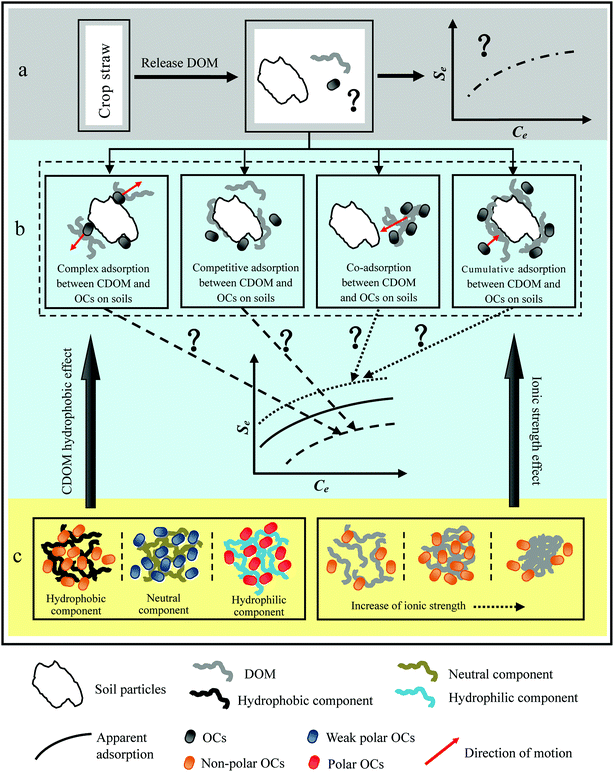 |
| Fig. 2 The process mechanism of interactions between CDOM and OCs. Note: Se and Ce are solid-phase and liquid-phase concentrations, respectively. | |
The migration process of OCs in farmland soil is likely to be involved in the process and mechanisms of CDOM, such as the one shown in the Fig. 2. CDOM contains a large number of oxygen functional groups, of which hydroxyl, can combine with groups on organic molecules to form H bonds.123 The H-bonding plays an important role in the adsorption of several non-ionic polar pesticides, such as 2,4-D, 2,4,5-T and dicamba, H-bonding also can be formed between –COOH, –COOR groups and organic matter, so as to control the OCs in the soil.124,125 As shown in Fig. 2(b), recent studies further reported that CDOM can form complexes with OCs or promote OCs migration in the soil by competing with the adsorption sites of soil particles.126,127 It can also enhance the adsorption capacity of OCs by co-adsorption or cumulative adsorption.128 The so-called co-adsorption is OCs being preferentially adsorbed to one or more components of the CDOM leading to the formation of complexes and CDOM at the same time adsorbed to the soil particles. And cumulative adsorption means that one or more components of CDOM are preferentially adsorbed by soil particles and the adsorption capacity of soil particles to certain OCs is enhanced with the increase of DOM adsorption capacity.129 Some researchers proposed the hydrophobic, neutral and hydrophilic components in CDOM by XAD-8/XAD-4 resin extraction and separation to study their effects on the migration behavior of OCs in soils, as shown in Fig. 2(c).130,131 These results indicated that the higher proportion of hydrophobic alkyl carbon and aromatic carbon in plant residues, the less biodegradable, and the higher proportion of hydrophilic polysaccharides, the more easily degraded, usually completed in a few months.132
Nowadays, an extensive effort has been devoted to understanding sorptive interactions between HOCs and natural DOM, particularly, the structure–activity correlations. Many studies reported that sorption of apolar HOCs (e.g., polycyclic aromatic hydrocarbons/PAHs, halogenated compounds, etc.) to DOM positively correlated to its aromaticity, which was attributed to the facilitated van der Waals (dispersion) and/or p–p interactions in sorption.133,134 The strong binding of apolar HOCs to DOM is often attributed to the abundance of aromatic moieties, which have relatively high electronic polarizability and hence facilitate van der Waals (dispersion) interactions with HOC molecules.135 Furthermore, the aromatic moieties of DOM can induce additional p–p coupling/stacking interactions with the π–π electron-bearing HOCs (such as PAHs). Instead, the aliphatic content of DOM was suggested to play a more important role in sorption of apolar HOCs. Additionally, the sorption affinity of apolar HOCs was often found to be inversely proportional to the polarity of DOM, suggesting the dominant role of hydrophobic partition. Regarding the sorption of polar organic pollutants, the presence of HA may have different effects as compared to HOCs due to that the two types of organic pollutants have different sorption mechanisms.136,137 Guo et al.138 studied that DOM impact on polar organic pollutants would be more pronounced at low DOM concentration values due to competition of DOM and polar O-containing pollutants for mineral surface sites. The process may be less significant at higher DOM concentration values because mineral surfaces become less selective towards oxygenated components. Therefore, non-polar OCs are more susceptible to approach low-polarity CDOM, while strongly polar OCs have stronger bonds with polar aromatic CDOM.139 When the hydrophobic component is dominant, CDOM may promote desorption of non-polar OCs from farmland soils. Also in hydrophobic microenvironment CDOM may increase the adsorption of non-polar OCs, while the hydrophilic components may accelerate the release of soil particle surface polar OCs.140
In the DOM-soil-OCs complex systems, the conformation of DOM is affected by its chemical and structural properties (e.g., aromaticity/aliphaticity, polarity, and molecular weight) as well as solution chemistry conditions such as pH.141 Low pH and high cationic intensity will reduce the repulsion between DOM molecules or molecules, promote the agglomeration of DOM molecules and change the hydrophobic nature of the system, but this effect is not monotonous.82,142,143 DOM particles contain abundant carboxyl and phenolic groups, which induce strong electrostatic repulsion when deprotonated at high pH, consequently leading to expanded, loose molecular configuration. On the other hand, at low pH the facilitated protonation of the oxygen-containing functional groups would reduce the electrostatic repulsion, and hence result in more compact DOM molecules with smaller apparent particle sizes. Similar observations on deceased particle sizes of DOM and other polyelectrolytes in response to pH decreases were reported in previous studies.144 As a result, the binding affinity of phenanthrene to DOM increased with decreasing pH due to the facilitated formation of compact pesudomicellar structures. A change in pH and ionic strength will greatly affect the interactions of DOM and polar or ionizable organic compounds.145 High cations such as Al3+, Fe3+, Ca2+, Mg2+, or toxic trace metal ions such as Cu2+, Mn2+, Pb2+, Cr3+, and Cd2+ may affect CDOM, monovalent ions such as Na+ and K+ can also affect CDOM.146 The presence of cations can increase the hydrophobic micro-environment of the CDOM molecules and enhance the complexation ability of non-polar OCs.147 A further increase in cations will make CDOM the number of cross-linked molecules increase, the structural tightening and hydrophobic microenvironment decrease and reduce the adsorption of non-polar OCs in soil as shown in Fig. 2(c). In addition, cations can also form complexes with certain OCs, such as amino groups in sulfamethoxazole; sulfonic acid oxygen and other groups are cationic complexation of the active site, and thereby change the existence of OCs and its behavior.148,149 Therefore, the extent and direction of influence of CDOM on the environmental behavior of OCs depends on its own properties, characteristics, and media conditions of interaction.
4.3 Effect of CDOM on solubility, transportation and degradation of OCs
CDOM can affect the mobility and solubility of soil pollutants. Kim et al., octanol–water partition coefficients (Kow), water solubility, and dissolved humic matter (DHM)–water partition coefficients (Koc) for 95 organic compounds were referred for estimating leachability of dioxins in the presence of DHM. The Koc and Kow values of dioxins were depended on chlorine content substituted. DHM in leachate influences on the solubility of dioxins, and thus the actual solubility or leachability of dioxins increase extraordinarily as increasing DHM and chlorine content. It means that dioxins abundant wastes (fly ash) should not be codisposed with organic abundant wastes (sewage sludge, food waste or bottom ash, etc.) to minimize the leachability of dioxins. Moreover, the presence of DOM reduces the harm of dioxins to soil.150 CDOM plays an important role in the transport of organic pollutants in soil. Wu et al.151 investigated the mechanism of DOM on adsorption behavior of PAEs in two different soil types. DBP (dibutyl phthalate) was used as a model compound due to its high toxicity and common PAEs contaminant in soils. To understand the influence of DBP adsorption kinetics and isotherms in soils under different concentrations of DOM, batch experiments were performed in combination with characterization using fourier transform infrared spectroscopy (FTIR) and nuclear magnetic resonance (NMR). The results examined the effect of DOM on adsorption of DBP in soils was related to the concentrations of DOM and soil type.151 The addition of DOM at low concentrations better enhanced the effect than at high concentrations. This pattern was more dominant in the red soil and less so in the black soil. The adsorption of DBP in soils was dominated by physical adsorption irrespective of DOM hydrophobic partition played a dominant role in DBP adsorption in soils, regardless of the presence of DOM. Addition of DOM promoted adsorption of DBP in soils mainly through partition. The adsorption mechanism of DBP in the two soil types was affected by addition of DOM differently.152 The intra-molecular and intermolecular hydrogen bond interactions of aromatic CC and CO in amides were involved in DBP adsorption in red soil. Therefore, DOM played a vital role in effecting transport of DBP in soils.153
It can be seen that the environmental geochemical behavior of OC is controlled to a certain extent by CDOM in farmland soils. Meanwhile it is considered that the environmental behavior of OCs in soils should be studied, not only to consider the adsorption of OCs on soils, but also because it is necessary to pay attention to the effects of CDOM and decay processes on OCs migration under conservation tillage. So it is an unavoidable problem for the appropriate environmental risk assessment and quality control of farmland ecosystems.
5 Future outlook
CDOM plays an important role in global carbon cycling and contaminant transport. The following future outlook can be drawn on the basis of the summary:
(1) CDOM can be divided into two main types: humic substances and non-humic substances. Most studies of CDOM-OCs based on the results of humic acid. However, research on the nature of CDOM is far from complete and the mechanisms of interactions between CDOM and OCs needs further study.
(2) Due to the structure of DOM is extremely complex, the information and structural properties that each analytical technique can respond to are different. Therefore, future studies are worth being undertaken to explore how this can be combined with a variety of advanced techniques further. This may result in more information for layer molecular structure of CDOM in soil.
(3) CDOM can directly or indirectly interactions with OCs by a series of reactions such as ion-exchange, adsorption–desorption, and complexation reactions in soil. The development of the environmental behavior of CDOM can gain more reasonable access to the characteristics of CDOM for control agro contaminants' pollution on soils and environmental restoration. Such investigations may be helpful for further studies aimed at risk assessment of OCs associated with application of CDOM to soils.
Conflicts of interest
There are no conflicts to declare.
Acknowledgements
This work was supported by the National Natural Science Foundation of China (Grant no. 41403081), the Key Research and Development Program of Sichuan Province of China (Grant no. 2017SZ0178), the National Key R&D Program of China (Grant no. 2016YFC0502204) and Scientific Research Fund of Sichuan Provincial Education Department (Grant no. 18ZA0500).
References
- Y. An, Z. Zhou, W. M. Qiao, W. Pan and Z. H. Chen, RSC Adv., 2017, 7, 42305–42311 RSC.
- R. Cantarero, P. Richter, S. Brown, L. Ascar and I. Ahumada, Environ. Sci. Pollut. Res., 2017, 24(14), 12847–12859 CrossRef CAS PubMed.
- X. P. Liao, C. X. Zhang, L. L. Yao, J. L. Li, M. Liu, L. Xu and M. Evalde, Sci. Total Environ., 2014, 473–474, 530–536 CrossRef CAS PubMed.
- Y. Zhang, S. Hu, H. Zhang, G. Shen, Z. Yuan and W. Zhang, Sci. Total Environ., 2017, 607–608, 1348–1356 CrossRef CAS PubMed.
- W. He, Z. L. Bai, Y. L. Li, X. Z. Kong, W. X. Liu, C. Yang, B. Yang and F. L. Xu, Sci. China: Earth Sci., 2016, 59, 746–759 CrossRef.
- H. Hu, Y. G. Wu, N. Yi, S. Zhang, Y. J. Zhang and X. Xin, Environ. Sci. Pollut. Res., 2017, 24, 21750–21760 CrossRef PubMed.
- X. X. Qu, L. Xie, Y. Lin, Y. C. Bai, Y. R. Zhu, F. Z. Xie, J. P. Giesy and F. C. Wu, Environ. Sci. Pollut. Res., 2013, 20, 7413–7423 CrossRef CAS PubMed.
- F. C. Wu and B. S. Xing, Natural organic matter and its significance in the environment, Science Press, Beijing, 2014 Search PubMed.
- J. Balch and C. Guéguen, Chemosphere, 2015, 119, 498–503 CrossRef CAS PubMed.
- Y. N. Liu, D. C. O. Thornton, T. S. Bianchi, W. A. Arnold, M. R. Shields, J. Chen and S. A. Yvon-Lewis, Environ. Sci. Technol., 2015, 49(6), 3366–3374 CrossRef CAS PubMed.
- I. Michael-Kordatou, C. Michael, X. Duan, X. He, D. D. Dionysiou, M. A. Mills and D. Fatta-Kassinos, Water Res., 2015, 77, 213–248 CrossRef CAS PubMed.
- X. Zhu, J. Z. Beiyuan, A. Y. T. Lau, S. S. Chen, D. C. W. Tsang, N. J. D. Graham, D. H. Lin, J. T. Sun, Y. H. Pan, X. Yang and X. D. Li, Sci. Total Environ., 2018, 619, 1153–1162 CrossRef PubMed.
- Z. F. Liu, Y. T. Han, M. Jing and J. W. Chen, J. Soils Sediments, 2017, 17(3), 771–779 CrossRef CAS.
- X. Y. Ren, G. M. Zeng, L. Tang, J. J. Wang, J. Wan, Y. N. Liu, J. F. Yu, H. Yi, S. J. Ye and R. Deng, Sci. Total Environ., 2018, 610–611, 1154–1163 CrossRef CAS PubMed.
- B. Glomstad, D. Altin, L. Sørensen, J. Liu, B. M. Jenssen and A. M. Booth, Environ. Sci. Technol., 2016, 50(5), 2660 CrossRef CAS PubMed.
- I. V. Perminova, N. Y. Grechishcheva, D. V. Kovalevskii, A. V. Kudryavtsev, V. S. Petrosyan and D. N. Matorin, Environ. Sci. Technol., 2001, 35(19), 3841–3848 CrossRef CAS PubMed.
- C. Yang, Z. Shen, G. Yu and J. Wang, Bioresour. Technol., 2008, 99(14), 6240–6245 CrossRef CAS PubMed.
- X. Y. Ren, G. M. Zeng, L. Tang, J. J. Wang, J. Wan, J. J. Wang, Y. C. Deng, Y. N. Liu and B. Peng, Waste Manag., 2018, 72, 138–149 CrossRef CAS PubMed.
- H. Lin, X. H. Xia, S. Q. Bi, X. M. Jiang, H. T. Wang, Y. W. Zhai and W. Wen, Environ. Sci. Technol., 2018, 52(2), 644–653 CrossRef CAS PubMed.
- D. Cai, X. Yang, S. Wang, Y. Chao, J. L. Morel and R. Qiu, J. Hazard. Mater., 2017, 324, 516–525 CrossRef CAS PubMed.
- X. H. Xia, A. H. Rabearisoa, X. M. Jiang and Z. N. Dai, Environ. Sci. Technol., 2013, 47(19), 10955–10963 CrossRef CAS PubMed.
- Y. L. Zhang, S. S. Lin, C. M. Dai, L. Shi and X. F. Zhou, Environ. Sci. Pollut. Res., 2014, 21, 5827–5835 CrossRef CAS PubMed.
- M. Xi, Y. Y. Zi, Q. G. Wang, S. Wang, G. L. Cui and F. L. Kong, Phys. Chem. Earth, 2018, 103, 35–44 CrossRef.
- H. L. Chen, J. M. Zhou and B. H. Xiao, J. Soils Sediments, 2010, 10(5), 915–922 CrossRef CAS.
- H. C. Fu, W. Guo, R. Q. Wang, X. Q. Zhang, M. M. Zhang, W. Ma and J. L. Dai, Soil Sediment Contam., 2015, 24, 624–638 CrossRef CAS.
- K. Kalbitz, S. Geyer and W. Geyer, Chemosphere, 2000, 40, 1305–1312 CrossRef CAS PubMed.
- Y. N. Hu, X. P. Liu, J. M. Bai, K. M. Shih, E. Y. Zeng and H. F. Cheng, Environ. Sci. Pollut. Res., 2013, 20(9), 6150–6159 CrossRef CAS PubMed.
- L. L. Niu, F. X. Yang, C. Xu, H. Y. Yang and W. P. Liu, Environ. Pollut., 2013, 176, 55–62 CrossRef CAS PubMed.
- L. Jiang, J. Huang, L. Liang, P. Y. Zheng and H. Yang, J. Agric. Food Chem., 2008, 56(24), 11933–11940 CrossRef CAS PubMed.
- K. Xiao, J. Y. Sun, Y. X. Shen, S. Liang, P. Liang, X. M. Wang and X. Huang, RSC Adv., 2016, 6, 24050–24059 RSC.
- A. C. Bejarano, A. Widenfalk, A. W. Decho and G. T. Chandler, Environ. Toxicol. Chem., 2003, 22, 2100–2105 CrossRef CAS PubMed.
- N. H. Song, X. L. Yin, G. F. Chen and H. Yang, Chemosphere, 2007, 68, 1779–1787 CrossRef CAS PubMed.
- N. H. Song, S. Zhang, M. Hong and H. Yang, Environ. Pollut., 2010, 158, 906–912 CrossRef CAS PubMed.
- J. Lee, J. H. Park, Y. S. Shin, B. C. Lee, N. I. Chang, J. Cho and S. D. Kim, Ecotoxicol. Environ. Saf., 2009, 72, 335–343 CrossRef CAS PubMed.
- M. Chen and J. Hur, Water Res., 2015, 79, 10–25 CrossRef CAS PubMed.
- C. E. W. Steinberg, Ecology of Humic Substances in Freshwaters, Springer, 2003 Search PubMed.
- J. Hur, M. H. Lee, H. Song and M. A. Schlatman, Environ. Sci. Pollut. Res., 2013, 20, 4176–4187 CrossRef CAS PubMed.
- Y. F. Wang, X. Y. Zhang, X. Zhang, Q. J. Meng, F. J. Gao and Y. Zhang, Chemosphere, 2017, 180, 531–539 CrossRef CAS PubMed.
- S. Chen, Y. K. Yang, C. Q. Liu, F. Q. Dong and B. J. Liu, Chemosphere, 2015, 141, 162–168 CrossRef CAS PubMed.
- Q. Wang, Z. C. Yang, B. Chai, S. Cheng, X. H. Lu and X. F. Bai, RSC Adv., 2016, 6, 14730–14740 RSC.
- M. Kočárek, R. Kodešová, L. Vondráčková, O. Golovko, M. Fér, A. Klement, A. Nikodem, O. Jakšík and R. Grabic, Environ. Pollut., 2016, 218, 563–573 CrossRef PubMed.
- R. Bi, Q. Lu, T. Yuan, S. G. Zhou, Y. Yuan and Y. F. Cai, J. Environ. Sci., 2013, 25(10), 2093–2101 CrossRef CAS.
- A. O. Badejo, J. Gal, S. M. Hyun, H. I. Yi and K. H. Shin, Quatern. Int., 2014, 344(4), 211–223 CrossRef.
- Y. Zhuang, R. Y. Li, H. Yang, D. L. Chen, Z. Y. Chen, B. B. Gao and B. He, Remote Sens., 2018, 10, 390 CrossRef.
- J. K. Gao, J. L. Lv, H. M. Wu, Y. C. Dai and M. Nasir, Chemosphere, 2018, 193, 1027–1035 CrossRef CAS PubMed.
- D. Bannister, M. Herzog, H. F. Graf, J. S. Hosking and C. A. Short, J. Clim., 2017, 30(17), 6701–6722 CrossRef.
- T. Inamura, Y. Mukai, A. Maruyama, S. Ikenaga, G. Li, X. M. Bu, Y. H. Xiang, D. Qin and T. Amano, Plant Soil, 2009, 315, 195–209 CrossRef CAS.
- X. L. Xu, L. Wang, H. Y. Cai, L. Y. Wang, L. Liu and H. Z. Wang, Food Secur., 2017, 9, 485–495 CrossRef.
- H. Ma, J. Zhang, L. Y. Tong and J. X. Yang, Environ. Sci.: Processes Impacts, 2015, 17(8), 1455–1461 RSC.
- L. A. Iturri, R. Funk, M. Leue, M. Sommer and D. E. Buschiazzo, Aeolian Res., 2017, 28, 39–49 CrossRef.
- A. O. Barakat, A. Mostafa, T. L. Wade, S. T. Sweet and N. B. El Sayed, Chemosphere, 2013, 93(3), 545–554 CrossRef CAS PubMed.
- H. A. N. Abdulla, E. C. Minor, R. F. Dias and P. G. Hatcher, Geochim. Cosmochim. Acta, 2013, 118(10), 231–246 CrossRef CAS.
- F. C. Wu, R. D. Evans and P. J. Dillon, Anal. Chim. Acta, 2002, 464, 47–55 CrossRef CAS.
- Y. Yamashita and E. Tanoue, Mar. Chem., 2003, 82, 255–271 CrossRef CAS.
- J. Lehmann and M. Kleber, Nature, 2015, 528(7580), 60–68 CrossRef CAS PubMed.
- R. Kalathoor, J. Botterweck, A. Schäffer, B. Schmidt and J. Schwarzbauer, J. Soils Sediments, 2015, 15, 659–670 CrossRef CAS.
- M. Li, Y. G. Chen, Y. L. Su, R. Wan and X. Zheng, RSC Adv., 2016, 6(18), 14993–15001 RSC.
- T. Ohno and C. S. Cronan, Int. J. Environ. Anal. Chem., 1997, 66, 119–136 CrossRef CAS.
- J. Labanowski and G. Feuillade, Water Res., 2011, 45, 315–327 CrossRef CAS PubMed.
- E. I. Karavanova, Eurasian Soil Sci., 2013, 46, 833–844 CrossRef CAS.
- M. Kitis, T. Karanfil, A. Wigton and J. E. Kilduff, Water Res., 2002, 36, 3834–3848 CrossRef CAS PubMed.
- L. F. Han, K. Sun, J. Jin, X. Wei, X. H. Xia, F. C. Wu, B. Gao and B. S. Xing, Environ. Sci. Technol., 2014, 48(19), 11227–11234 CrossRef CAS PubMed.
- D. Zhang, B. Pan, L. C. Robert and B. S. Xing, Environ. Pollut., 2015, 196, 292–299 CrossRef CAS PubMed.
- C. Panagiotopoulos, D. J. Repeta, L. Mathieu, J. F. Rontani and R. Sempéré, Mar. Chem., 2013, 154(5), 34–45 CrossRef CAS.
- L. Hahn, M. M. Lubtow, T. Lorson, F. Schmitt, A. Appelt-Menzel, R. Schobert and R. Luxenhofer, Biomacromolecules, 2018, 19(7), 3119–3128 CrossRef CAS PubMed.
- S. Xue, Y. Wen, X. J. Hui, L. N. Zhang, Z. H. Zhang, J. Wang and Y. Zhang, J. Environ. Sci., 2015, 27, 168–178 CrossRef PubMed.
- D. N. Chang, W. D. Cao, J. S. Bai, S. J. Gao, X. C. Wang, N. H. Zeng and S. Katsuyoshi, Spectrosc. Spectral Anal., 2017, 37(1), 221–226 CAS.
- N. H. Song, L. Chen and H. Yang, Geoderma, 2008, 146, 344–352 CrossRef CAS.
- R. Rasool, S. S. Kukal and G. S. Hira, Soil Tillage Res., 2008, 101, 31–36 CrossRef.
- H. L. Chen, J. M. Zhou and B. H. Xiao, J. Soils Sediments, 2010, 10, 915–922 CrossRef CAS.
- S. H. Wu, H. J. He, X. Inthapanya, C. P. Yang, L. Lu, G. M. Zeng and Z. F. Han, Environ. Sci. Pollut. Res., 2017, 24(20), 16560–16577 CrossRef CAS PubMed.
- M. Xi, Y. Y. Zi, Q. G. Wang, S. Wang, G. L. Cui and F. L. Kong, Phys. Chem. Earth, 2018, 103, 35–44 CrossRef.
- E. A. Kim, H. V. M. Nguyen, H. S. Oh, J. Hur and J. H. Choi, Environ. Sci. Pollut. Res., 2016, 23, 5203–5213 CrossRef CAS PubMed.
- J. B. Fellman, E. Hood and R. G. M. Spencer, Limnol. Oceanogr., 2010, 55, 2452–2462 CrossRef CAS.
- M. Heinz, D. Graeber, D. Zak, E. Zwirnmann, J. Gelbrecht and M. T. Pusch, Environ. Sci. Technol., 2015, 49, 2081–2090 CrossRef CAS PubMed.
- J. A. Leenheer and J. P. Croué, Environ. Sci. Technol., 2003, 37, 18A–26A CrossRef CAS PubMed.
- E. C. Minor, M. M. Swenson, B. M. Mattson and A. R. Oyler, Environ. Sci.: Processes Impacts, 2014, 16, 2064–2079 RSC.
- E. M. Perdue and J. F. Koprivnjak, Estuarine, Coastal Shelf Sci., 2007, 73, 65–72 CrossRef.
- A. Huguet, L. Vacher, S. Relexans, S. Saubusse, J. M. Froidefond and E. Parlanti, Org. Geochem., 2009, 40, 706–719 CrossRef CAS.
- D. N. Kothawala, C. A. Stedmon, R. A. Müller, G. A. Weyhenmeyer, S. J. Köhler and L. J. Tranvik, Glob. Change Biol., 2014, 20, 1101–1114 CrossRef PubMed.
- N. M. Mazrui, S. Jonsson, S. Thota, J. Zhao and R. P. Mason, Geochim. Cosmochim. Acta, 2016, 194, 153–162 CrossRef CAS PubMed.
- N. Mladenov, Y. Zheng, B. Simone, T. M. Bilinski, D. M. McKnight, D. Nemergut, K. A. Radloff, M. M. Rahamn and K. M. Ahmed, Environ. Sci. Technol., 2015, 49, 10815–10824 CrossRef CAS PubMed.
- T. Jiang, J. Kaal, J. Liang, Y. L. Zhang, S. Q. Wei, D. Y. Wang and N. W. Green, Sci. Total Environ., 2017, 603, 461–471 CrossRef PubMed.
- C. Chen, J. J. Dynes, J. Wang and D. L. Sparks, Environ. Sci. Technol., 2014, 48(23), 13751–13759 CrossRef CAS PubMed.
- B. Pan, S. Ghosh and B. S. Xing, Environ. Sci. Technol., 2007, 41, 6472–6478 CrossRef CAS PubMed.
- Y. Y. Li, C. M. Xu, K. H. Chung and Q. Shi, Energy Fuels, 2014, 29, 2923–2930 CrossRef.
- D. F. Ma, B. Peng, Y. H. Zhang, B. Y. Gao, Y. Wang, Q. Y. Yue and Q. Li, Bioresour. Technol., 2014, 165, 81–87 CrossRef CAS PubMed.
- A. Nuzzo, A. Sanchez, B. Fontaine and A. Piccolo, J. Geochem. Explor., 2013, 129, 1–5 CrossRef CAS.
- A. D. Neilen, D. W. Hawker, K. R. O'Brien and M. A. Burford, Chemosphere, 2017, 184, 969–980 CrossRef CAS PubMed.
- S. D. Li, M. L. Zhang, H. Yang, D. Q. Liu, L. Y. Yu, T. Huang and C. C. Huang, Spectrosc. Spectral Anal., 2017, 37, 1183–1188 CAS.
- P. Sharma, Y. Laor, M. Raviv, S. Medina, I. Saadi, A. Krasnovsky, M. Vager, G. J. Levy, A. Bar-Tal and M. Borisover, Geoderma, 2017, 305, 197–207 CrossRef CAS.
- W. Chen, P. Westerhoff, J. A. Leenheer and K. Booksh, Environ. Sci. Technol., 2003, 37(24), 5701–5710 CrossRef CAS PubMed.
- J. H. Choi, Y. G. Kim, Y. K. Lee, S. P. Pack, J. Y. Jung and K. S. Jang, Biotechnol. Bioprocess Eng., 2017, 22(5), 637–646 CrossRef CAS.
- I. V. Kemkin and R. A. Kemkina, Dokl. Earth Sci., 2014, 455, 475–480 CrossRef CAS.
- N. A. Mikhailova, V. S. Arzhanova and S. A. Apollonova, Eurasian Soil Sci., 2004, 37, 500–505 Search PubMed.
- T. N. Lutsenko, V. S. Arzhanova and S. Y. Bratskaya, Eurasian Soil Sci., 2014, 47, 581–590 CrossRef CAS.
- Z. R. Tang, C. H. Huang, W. B. Tan, X. S. He, H. Zhang, D. Li and B. D. Xi, Chin. J. Anal. Chem., 2018, 46, 422–430 CAS.
- P. Boguta, P. M. Pieczywek and Z. Sokołowska, Sensors, 2016, 16, 1760 CrossRef PubMed.
- W. He and J. Hur, Water Res., 2015, 83, 217–226 CrossRef CAS PubMed.
- K. M. G. Mostofa, F. C. Wu, C. Q. Liu, W. L. Fang, J. Yuan, W. L. Ying, L. Wen and M. Yi, Limnology, 2010, 11, 217–231 CrossRef CAS.
- H. J. Lei, Y. P. Han and X. Liu, Spectrosc. Spectral Anal., 2015, 35, 1926–1932 CAS.
- Y. L. Zhang, S. H. Yao, J. D. Mao, D. C. Olk, X. Y. Cao and B. Zhang, Soil Biol. Biochem., 2015, 85, 137–144 CrossRef CAS.
- H. W. Pan, H. B. Yu, Y. H. Song, L. Zhu, R. X. Liu and E. D. Du, Environ. Sci. Pollut. Res., 2017, 24, 6563–6571 CrossRef CAS PubMed.
- W. Wu, H. J. Sheng, C. G. Gu, Y. Song, S. Willbold, Y. Qiao, G. X. Liu, W. Zhao, Y. Wang, X. Jiang and F. Wang, Sci. Total Environ., 2018, 631, 1495–1503 CrossRef PubMed.
- S. E. M. Dvorski, M. Gonsior, N. Hertkorn, J. Uhl, H. Muller, C. Griebler and P. Schmitt-Kopplin, Environ. Sci. Technol., 2016, 50, 5536–5546 CrossRef CAS PubMed.
- H. B. Peng, B. Pan, M. Wu, R. Liu, D. Zhang, D. Wu and B. S. Xing, J. Hazard. Mater., 2012, 211–212, 342–348 CrossRef CAS PubMed.
- B. Z. Zhao, J. B. Zhang, Y. Y. Yu, D. L. Karlen and X. Y. Hao, Environ. Sci. Pollut. Res., 2016, 23(17), 17581–17591 CrossRef CAS PubMed.
- M. Yamamoto, A. Nishida, K. Otsuka, T. Komai and M. Fukushima, Bioresour. Technol., 2010, 101, 4456–4460 CrossRef CAS PubMed.
- B. Wang, X. Shen, S. Chen, Y. C. Bai, G. Yang, J. P. Zhu, J. C. Shu and Z. Y. Xue, Ecol. Indic., 2018, 93, 998–1004 CrossRef.
- M. J. M. Rusan and H. I. Malkawi, Desalin. Water Treat., 2016, 57(57), 27945–27953 CAS.
- T. Ahmed, K. Ohta, O. Nagafuchi and M. Maruo, Environ. Toxicol. Chem., 2012, 31, 2201–2209 CrossRef CAS PubMed.
- S. Frankki, Y. Persson, M. Tysklind and U. Skyllberg, Environ. Sci. Technol., 2006, 40, 6668–6673 CrossRef CAS PubMed.
- J. H. Writer, J. N. Ryan and L. B. Barber, Environ. Sci. Technol., 2011, 45, 7275–7283 CrossRef CAS PubMed.
- S. Xue, Q. L. Zhao, L. L. Wei, Y. T. Song and M. Tie, Environ. Monit. Assess., 2013, 185, 4591–4603 CrossRef CAS PubMed.
- H. Y. Sun, X. Shi, J. D. Mao and D. Q. Zhu, Environ. Toxicol. Chem., 2010, 29, 1934–1942 CAS.
- A. Muscolo, M. Sidari and S. Nardi, J. Geochem. Explor., 2013, 129, 57–63 CrossRef CAS.
- O. E. Trubetskaya, O. A. Trubetskoj, G. Voyard and C. Richard, J. Geochem. Explor., 2013, 132, 84–89 CrossRef CAS.
- M. Q. Yan, D. Dryer, G. V. Korshin and M. F. Benedetti, Water Res., 2013, 47, 588–596 CrossRef CAS PubMed.
- C. H. Fan, Y. C. Zhang and J. H. Wang, Spectrosc. Spectral Anal., 2015, 35, 3146–3150 CAS.
- J. H. Writer, J. N. Ryan and L. B. Barber, Environ. Sci. Technol., 2011, 45, 7275–7283 CrossRef CAS PubMed.
- M. X. Wei, B. Wang, S. Chen, Y. C. Bai, F. Q. Dong, J. P. Zhu, M. Li and J. Wei, Spectrosc. Spectral Anal., 2017, 37(9), 2861–2868 CAS.
- J. K. Gao, J. L. Lv, H. M. Wu, Y. C. Dai and M. Nasir, Chemosphere, 2018, 193, 1027–1035 CrossRef CAS PubMed.
- E. E. Lavonen, D. N. Kothawala, L. J. Tranvik, M. Gonsior, P. Schmitt-Kopplin and S. J. Kohler, Water Res., 2015, 85, 286–294 CrossRef CAS PubMed.
- B. N. Bhadra and S. H. Jhung, J. Hazard. Mater., 2017, 340, 179–188 CrossRef CAS PubMed.
- Q. Q. Wu, Q. Yang, W. J. Zhou and L. Z. Zhu, J. Soils Sediments, 2015, 15, 2210–2219 CrossRef CAS.
- N. Liang, D. Zhang, C. X. Wei, H. Li, S. Ghosh, Y. B. Cao and B. L. Guo, Environ. Eng. Sci., 2015, 32, 703–712 CrossRef CAS.
- D. M. Wu, Y. H. Yun, L. Jiang and C. Y. Wu, Sci. Total Environ., 2018, 616, 1449–1456 CrossRef PubMed.
- S. Xue, Q. L. Zhao, L. L. Wei, Y. T. Song and M. Tie, Environ. Monit. Assess., 2013, 185, 4591–4603 CrossRef CAS PubMed.
- T. Polubesova and B. Chefetz, Crit. Rev. Environ. Sci. Technol., 2014, 44, 223–254 CrossRef CAS.
- K. L. Yang, Y. L. Zhang, Y. P. Dong, Z. Nie and W. Li, Environ. Eng. Sci., 2017, 34, 675–686 CrossRef CAS.
- A. Y. Zherebker, I. V. Perminova, A. I. Konstantinov, A. B. Volikov, Y. I. Kostyukevich, A. S. Kononikhin and E. N. Nikolaev, J. Anal. Chem., 2016, 71, 372–378 CrossRef CAS.
- M. Markiewicz, C. Jungnickel and H. P. H. Arp, Environ. Sci. Technol., 2013, 47, 6951–6958 CrossRef CAS PubMed.
- H. B. Wang and Y. J. Zhang, J. Environ. Sci. Health, Part A: Toxic/Hazard. Subst. Environ. Eng., 2014, 49(1), 78–84 CrossRef CAS PubMed.
- H. Y. Fu, C. H. Wei, X. L. Qu, H. Li and D. Q. Zhu, Environ. Pollut., 2018, 232, 402–410 CrossRef CAS PubMed.
- J. Jin, K. Sun, Z. Y. Wang, Y. Yang, L. F. Han and B. S. Xing, Environ. Sci. Technol., 2017, 51(5), 2635–2642 CrossRef CAS PubMed.
- C. P. Yang, H. Qian, X. Li, Y. Cheng, H. J. He, G. M. Zeng and J. Y. Xi, Trends Biotechnol., 2018, 36(7), 673–685 CrossRef CAS PubMed.
- Y. Cheng, H. J. He, C. P. Yang, G. M. Zeng, X. Li, H. Chen and G. L. Yu, Biotechnol. Adv., 2016, 34(6), 1091–1102 CrossRef CAS PubMed.
- X. Y. Guo, X. L. Wang, X. Z. Zhou, X. Z. Kong, S. Tao and B. S. Xing, Environ. Sci. Technol., 2012, 46(13), 7252–7259 CrossRef CAS PubMed.
- Y. L. Li, W. He, W. X. Liu, X. Z. Kong, B. Yang, C. Yang and F. L. Xu, Environ. Pollut., 2015, 206, 461–468 CrossRef CAS PubMed.
- M. Uchimiya and D. I. Bannon, J. Agric. Food Chem., 2013, 61, 7679–7688 CrossRef CAS PubMed.
- K. L. Chen, L. C. Liu and W. R. Chen, Environ. Pollut., 2017, 231, 1163–1171 CrossRef CAS PubMed.
- L. Ming, L. H. Li, Y. X. Zhang, W. Y. Lin, G. Z. Wang, S. Zhang and P. Guo, Chem. Ecol., 2014, 30, 76–86 CrossRef CAS.
- C. Y. Yuan, Y. P. Chin and L. K. Weavers, Water Res., 2018, 132, 52–60 CrossRef CAS PubMed.
- M. L. Pace, I. Reche, J. J. Cole, A. Fernandez-Barbero, I. P. Mazuecos and Y. T. Prairie, Biogeochemistry, 2012, 108(1–3), 109–118 CrossRef CAS.
- D. Gondar, R. Lopez, J. Antelo, S. Fiol and F. Arce, J. Hazard. Mater., 2012, 235–236(2), 218–223 CrossRef CAS PubMed.
- B. Pan, M. Y. Qiu, M. Wu, D. Zhang, H. B. Peng, D. Wu and B. S. Xing, Environ. Pollut., 2012, 161, 76–82 CrossRef CAS PubMed.
- D. Durce, N. Maes, C. Bruggeman and L. Van Ravestyn, J. Contam. Hydrol., 2016, 185, 14–27 CrossRef PubMed.
- A. H. M. A. Sadmani, R. C. Andrews and D. M. Bagley, Chemosphere, 2014, 117, 170–177 CrossRef CAS PubMed.
- Y. L. Wang, C. M. Yang, L. M. Zou and H. Z. Cui, Appl. Spectrosc., 2015, 69, 623–634 CrossRef CAS PubMed.
- Y. Kim and D. Lee, J. Hazard. Mater., 2002, 91(1), 113–127 CrossRef CAS PubMed.
- W. Wu, H. J. Sheng, C. G. Gu, Y. Song, S. Willbold, Y. Qiao, G. X. Liu, W. Zhao, Y. Wang, X. Jiang and F. Wang, Sci. Total Environ., 2018, 631, 1495–1503 CrossRef PubMed.
- Y. H. Wu, Y. B. Si, D. M. Zhou and J. Gao, Chemosphere, 2015, 119, 690–696 CrossRef CAS PubMed.
- Y. F. Wang, X. Y. Zhang, X. Zhang, Q. J. Meng, F. J. Gao and Y. Zhang, Chemosphere, 2017, 180, 531–539 CrossRef CAS PubMed.
|
This journal is © The Royal Society of Chemistry 2018 |
Click here to see how this site uses Cookies. View our privacy policy here.