DOI:
10.1039/C8RA07255H
(Paper)
RSC Adv., 2018,
8, 40219-40231
The potential use of thermosensitive chitosan/silk sericin hydrogels loaded with longan seed extract for bone tissue engineering†
Received
30th August 2018
, Accepted 9th November 2018
First published on 3rd December 2018
Abstract
In this study, hydrogels that were thermosensitive at body temperature were developed using chitosan (CS)/silk sericin (SS)/β-glycerophosphate (β-GP) loaded with longan seed extract (LE) for use in bone tissue engineering. These hydrogels were transformed into a gel at 37 °C within 10 min via interactions between CS and β-GP. The incorporation of SS resulted in a shorter gelation time of 5–7 min. The morphological structure of the thermosensitive CS/β-GP hydrogels exhibited an irregular pore structure, whereas the morphological structure of the thermosensitive CS/SS/β-GP hydrogels became more slender and porous. The incorporation of SS affected the network structure of the CS hydrogels, which degraded more rapidly. Moreover, the cumulative amounts of both gallic acid (GA) and ellagic acid (EA) released from the hydrogels loaded with LE increased with an increase in the SS content. Finally, these thermosensitive hydrogels were non-toxic to both a mouse fibroblast cell line (NCTC clone 929) and a mouse osteoblast cell line (MC3T3-E1) and promoted the attachment of MC3T3-E1 cells to the surface of the hydrogels. Therefore, these thermosensitive hydrogels might be a promising candidate for bone tissue engineering.
1 Introduction
Thermosensitive hydrogels are materials that are in the liquid form for a desired period of time below or at room temperature and turn into a gel at physiological or body temperature after a few minutes.1 Thermosensitive hydrogels have aroused a great deal of interest in various applications (e.g., drug delivery,2 tissue engineering,3 cell encapsulation,4 etc.). Such thermosensitive hydrogel systems may provide several advantages over conventional hydrogels for drug delivery systems, including protection of the drug from the environment and prolongation of drug release. Besides, their initial liquid formulations can flow to the target site without a surgical procedure for placing and filling a defect by virtue of their fluidity to exactly fit the interior space.5 Hence, the operational performance requirements for implantation are not necessary.
Chitosan (CS)-based materials have been used in the medical and pharmaceutical fields as biomaterials in the development of targeted and controlled drug delivery because of their biodegradability, biocompatibility, non-toxicity, low immunogenicity, antibacterial nature, and good cell adhesion.6,7 β-Glycerophosphate (β-GP) has been used as a catalyst for achieving a sol-to-gel transition in CS solutions at a physiological temperature or 37 °C.3 In addition, thermosensitive chitosan/β-glycerophosphate (CS/β-GP) hydrogels have been utilized as an intelligent drug delivery system to improve patient compliance by maintaining the range of doses of therapeutic drugs for a long time while avoiding the requirement for frequent injections of therapeutic drugs.8
Currently, silk sericin (SS) has received increasing attention as an alternative option in bone tissue engineering owing to its environmentally friendly, renewable and non-toxic properties. It has several biological advantages, such as low immunogenicity, biodegradability, and excellent biocompatibility.9 Moreover, it has antioxidant activities,10 antibacterial activities,11 and anticoagulation activities12 and can promote cell growth and differentiation.13 Hence, it has become an attractive option as a biomaterial in the field of regenerative medicine. However, it was blended with other polymers to improve their properties for use in biomedical applications.14 Therefore, SS could be used as an additional component to enhance the thermogelling property and biocompatibility of thermosensitive hydrogels.
The longan, or Dimocarpus longan Lour. (syn. Euphoria longana Lam.), is a semitropical fruit that is widely cultivated in Northern Thailand and is called “Lamyai” in Thailand.15 Longan seeds contain large amounts of polyphenolic compounds such as corilagin, gallic acid (GA), and ellagic acid (EA),16 but the pulp and peel contain small amounts of polyphenolic compounds. GA and EA have been used in several studies to promote their health benefits owing to their antimicrobial, antioxidant and anti-inflammatory activities.17 Besides, it has been reported that EA can be used to prevent alveolar bone loss and accelerate the formation of new bone after teeth are extracted.18
In this study, novel thermosensitive hydrogels based on chitosan (CS) and silk sericin (SS) and loaded with longan seed extract (LE) as a model drug were developed to achieve a balance between the thermogelling property and biocompatibility for use in bone tissue engineering. It was hypothesized that a system based on a combination of CS and SS could form a sol at room temperature and be transformed rapidly into a gel state at physiological temperature. The properties of these thermosensitive hydrogels, including molecular structures, rheological behavior, and morphological characteristics, were characterized using Fourier transform infrared (FTIR) spectroscopy, rheometry, and scanning electron microscopy (SEM), respectively. Furthermore, the degradability, profiles of the in vitro release of both GA and EA from the hydrogels, cytotoxicity, and cell morphology were studied to investigate the potential use of these materials in bone tissue engineering.
2 Experimental
2.1 Materials
CS derived from snow crab shells/Alaska crab shells (viscosity = 600–900 cps, degree of deacetylation ≥ 95%) was purchased from Bio 21 Co., Ltd (Chonburi, Thailand). Raw silk cocoons from the mulberry silkworm Bombyx mori were obtained from the Queen Sirikit Sericulture Center (Chiang Mai, Thailand). β-Glycerophosphate disodium salt hydrate, GA, EA, glycine, and 2,2-diphenyl-1-picrylhydrazyl (DPPH) were purchased from Sigma-Aldrich (USA). L-Ascorbic acid was purchased from Chem-Supply Pty Ltd (Australia). Anhydrous sodium carbonate (Na2CO3), sodium chloride (NaCl), anhydrous disodium hydrogen orthophosphate (Na2HPO4), potassium chloride (KCl), and monopotassium phosphate (KH2PO4) were purchased from Ajax Chemicals (Australia). Alpha-amylase was purchased from HiMedia Laboratories (India). Ethanol, methanol, and dimethyl sulfoxide (DMSO) were purchased from RCI LabScan Limited (Bangkok, Thailand). Glacial acetic acid was purchased from Merck KGaA (Germany). All chemicals were of analytical reagent grade and were used without further purification.
2.2 Extraction of longan seed
The longan cultivar Edor (Dimocarpus longan Lour.) was used in this study. Edor is a commercial cultivar available in Northern Thailand. Fresh longan fruit were obtained from a longan farm in Lamphun province, Thailand. Longan seed was used for extraction because it contains large amounts of GA and EA. Longan seed extract (LE) was extracted using an organic solvent. Firstly, 200 mg of dried ground longan seed was accurately weighed and extracted three times with 50 mL of mixtures with different fractions of water and ethanol (100
:
0, 70
:
30, 50
:
50, 30
:
70, and 0
:
100) at room temperature for 3 days. The extracts from each extraction process were then combined and filtered through filter paper. After that, ethanol was evaporated from each extract, which was dried using a freeze-drying method. Finally, the percentage yield of each fraction of LE was calculated.
LE from each fraction was analyzed to determine the contents of polyphenolic compounds (i.e., GA and EA) using a Waters 600E high-performance liquid chromatography (HPLC) system. Separation was performed with an Alltech Prevail C18 column (250 mm × 4.6 mm, 5 μm), and the temperature of the column was set at 25 °C. The mobile phase consisted of 0.1% (v/v) formic acid in water (solvent A) and methanol (solvent B) for gradient elution (0 min, 96
:
4; 27 min, 20
:
80; 28 min, 96
:
4 and 33 min, 96
:
4) with a total run time of 33 min at a flow rate of 1.0 mL min−1. The sample injection volume was 10 μL. The detection of polyphenolic compounds was achieved using a UV-vis spectrophotometer with a diode array detector at 270 nm. Standard solutions with seven concentrations (25, 50, 100, 150, 200, 250, and 300 ppm) of GA and EA were injected, and then a calibration curve was obtained by plotting the peak area against the concentration of each sample. Moreover, the antioxidant activity and cytotoxicity of LE were determined using a 2,2-diphenyl-1-picrylhydrazyl (DPPH) radical scavenging assay and a resazurin microplate assay (REMA), respectively, as shown in the ESI.†
2.3 Preparation of thermosensitive chitosan/β-glycerophosphate (CS/β-GP) hydrogels
Firstly, the optimal conditions for preparing the thermosensitive CS/β-GP hydrogels were identified. CS solutions with various concentrations (3.0, 3.5, and 4.0%, w/v) were prepared by dissolving CS powder in an aqueous acetic acid solution (1%, v/v). The CS solutions were stirred well to give a homogeneous solution at room temperature. In addition, a 50% (w/v) solution of β-GP was prepared by dissolving β-GP powder in deionized water. The CS and β-GP solutions were cooled to 4 °C using an ice bath for 15 min. Then, the 50% (w/v) solution of β-GP was added dropwise to the CS solution under stirring for 20 min with various volume ratios of CS to β-GP (0.7
:
0.3, 0.5
:
0.5, and 0.3
:
0.7 mL).
The pH of the solution prepared in each set of conditions was measured to ensure a physiologically acceptable neutral solution at room temperature using a Eutech Cyberscan pH 510 meter (Thermo Scientific, Germany) before the determination of the gelation time. The freshly prepared thermosensitive CS/β-GP hydrogels were examined by a simple test tube inversion method. A 1 mL sample of the solution was added to a vial in a temperature-controlled bath at 37 °C. In order to observe whether the solution had undergone gelation, the vial was inverted horizontally, and the gelation time was defined as the time at which the solution no longer flowed.
2.4 Preparation of silk sericin and thermosensitive chitosan/silk sericin/β-glycerophosphate (CS/SS/β-GP) hydrogels
Raw Thai silk cocoons from B. mori silkworms were cut into small pieces and degummed with 0.5% (w/v) sodium carbonate (Na2CO3) at 85 °C for 45 min. The aqueous solution obtained from boiling silk cocoons was collected and was denoted as silk sericin (SS). This aqueous solution was then filtered to remove insoluble materials (or degummed silk fibers). The SS solution was dialyzed in deionized water for 3 days using a cellulose membrane (Cellu-Sep® T4, nominal MWCO: 12
000–14
000), and the deionized water was replaced every 12 h to remove salts. Lipids and impurities were removed from the SS solution by centrifugation at 3500 rpm at 25 °C for 20 min. Finally, the SS solution was lyophilized using a freeze-drying method at −40 °C for 19 h.
Thermosensitive CS/SS/β-GP hydrogels were prepared with a fixed concentration of CS (3.5%, w/v) and SS solutions with various concentrations (1.5, 2.5 and 3.5%, w/v). The incorporation of SS into the CS solution was chosen to examine its effect on the gelation time of the modified hydrogels. Firstly, a 3.5% (w/v) solution of CS was prepared by dissolving CS powder in an aqueous acetic acid solution (1%, v/v). Then, 1.5, 2.5, and 3.5% (w/v) solutions of SS were prepared by dissolving SS powder in deionized water. The mixture of CS and SS solutions prepared in each set of conditions was well stirred to give a homogeneous solution at room temperature. A 50% (w/v) solution of β-GP was prepared by dissolving β-GP powder in deionized water. After that, all the solutions were cooled to 4 °C using an ice bath for 15 min. Then, the 50% (w/v) solution of β-GP was added dropwise to the mixture of CS and SS solutions under stirring for 20 min with a volume ratio of the CS/SS mixture to the β-GP solution that was equal to 0.5
:
0.5 mL.
The pH of the solution prepared in each set of conditions was measured to ensure a physiologically acceptable neutral solution at room temperature using a pH meter before the determination of the gelation time. Then, the freshly prepared thermoresponsive hydrogels were examined by a simple test tube inversion method according to the previous test procedure.
2.5 Rheological and viscosity measurements
Rheological measurements of the thermosensitive CS/β-GP and CS/SS/β-GP hydrogels were carried out using a Bohlin Gemini 200HR nano-rotational rheometer (Malvern, UK) equipped with a parallel geometry with a diameter of 40 mm, and the gap was set to 1 mm. A 1 mL sample of the solutions was placed on top of the rheometer and equilibrated at 20 °C for 4 min. During the gelation process, the rheological properties of samples were measured within the temperature range of 20 °C to 50 °C at a heating rate of 2 °C min−1 and a frequency of 3 Hz. Changes in the elastic (storage) modulus (G′) and the viscous (loss) modulus (G′′) were recorded as a function of temperature.
The sol–gel transition behavior of the thermosensitive CS/β-GP and CS/SS/β-GP hydrogels was investigated in terms of the viscosity of the hydrogels using a Bohlin Gemini 200HR nano-rotational rheometer (Malvern, UK) within the temperature range of 20–50 °C.
2.6 Chemical structure and morphological study
The thermosensitive CS/β-GP and CS/SS/β-GP hydrogels were incubated in a water bath at 37 °C prior to being observed. When the hydrogels were transformed into gels, the gels were frozen at −40 °C for 24 h and then lyophilized by a freeze-drying method for 19 h. A sample and potassium bromide were ground into a homogeneous fine powder and then pressed into a disc. Measurements were carried out in the range of 4000–700 cm−1 with a resolution of 4 cm−1 using a Spectrum GX Fourier transform infrared spectrometer (PerkinElmer, USA).
The surface of the freeze-dried hydrogels was then coated with a thin layer of gold under vacuum, and the morphology was observed using a LEO 1450 VP scanning electron microscope (Carl Zeiss, Germany) with an accelerating voltage of 10 kV.
2.7 Weight loss
The weight loss behavior of the thermosensitive CS/β-GP and CS/SS/β-GP hydrogels was examined by an immersion method under simulated physiological conditions. A 1 mL sample of each thermosensitive hydrogel was formed under incubation at 37 °C for 3 h. Each hydrogel was cut into small pieces of about 5 mm × 5 mm and then weighed (Wi) and immersed in 2 mL of phosphate-buffered saline (PBS) (pH 7.4). After that, each sample was incubated at 37 °C under continuous shaking. At predetermined time intervals (1, 3, 5, 7 and 10 days), the sample was carefully removed from the medium and weighed (Wt) after being dried. The weight loss percentage (W%) of the hydrogels was calculated according to the following equation:
2.8 In vitro degradation
The in vitro degradation of the thermosensitive CS/β-GP and CS/SS/β-GP hydrogels was examined by an immersion method under simulated physiological conditions. A 1 mL sample of each thermosensitive hydrogel was formed under incubation at 37 °C for 3 h and then immersed in 6 mL of either PBS (pH 7.4) or PBS (pH 6.8) containing α-amylase. After that, each sample was incubated at 37 °C under continuous shaking. At predetermined time intervals (1, 3, 5, 7, and 10 days), 1 mL of the medium after the sample was immersed was carefully withdrawn and the same amount of fresh medium was added. A ninhydrin assay was used to determine the number of free amino groups in the released medium. Glycine was used as a standard amino acid. After incubation with ninhydrin reagent at 95 °C for 10 min, the solution was left to cool and an ethanol solution was then added as a stabilizing solvent. Finally, the absorbance of the solution at 570 nm was measured using a Lambda 35 UV-vis spectrophotometer (PerkinElmer, USA).
2.9 In vitro release of longan seed extract
LE, as a model compound, was dissolved in deionized water. Firstly, 5 mL of each CS/β-GP or CS/SS/β-GP solution was mixed with an LE solution (0.5 mg mL−1) to form a homogeneous solution. Then, 1 mL of each hydrogel loaded with LE was placed in a tube and incubated at 37 °C to form a gel. After that, 6 mL of PBS (pH 7.4) was added to a tube. All samples were incubated at 37 °C under continuous shaking. At predetermined time intervals, 1 mL of the released medium was removed and stored at 4 °C until analyzed and was immediately replaced with fresh medium to maintain a constant volume. Finally, the cumulative released amounts of both GA and EA as a function of time were determined using a UV-vis spectrophotometer and calculated by constructing a calibration curve of the absorbance versus the concentration of the compounds from 0 to 100 mg L−1.
2.10 In vitro cytotoxicity and cell morphology
An indirect evaluation of the cytotoxicity of the thermosensitive CS/β-GP and CS/SS/β-GP hydrogels loaded with LE was performed using NCTC clone 929 (22nd passage) or MC3T3-E1 (4th passage) cells. The NCTC clone 929 cells were obtained from mouse areolar fibroblasts (ATCC® CCL-1™), whereas the preosteoblasts (MC3T3-E1 cells) were obtained from normal mouse subclone 4 cells (ATCC® CRL-2593™). The hydrogels were first incubated at 37 °C for 3 h and then immersed in serum-free medium (SFM) containing Dulbecco's modified Eagle medium (DMEM; Gibco, USA) or minimum essential medium (MEM; Gibco, USA) and a 1% antibiotic-antimycotic formulation (Gibco, USA) for an incubation period of 24 h to produce extraction media with various concentrations (5, 10, and 50 mg mL−1). Before testing, the extraction media were sterilized using 0.22 μm Minisart syringe filters (Sartorius, Germany). NCTC clone 929 and MC3T3-E1 cells were separately cultured in wells of a 96-well TCPS plate (SPL Life Science, Korea) at 8000 cells per well in DMEM or MEM for 24 h to enable cell attachment. The cells were then starved with SFM for 12 h. After that, the medium was replaced with the extraction medium and the cells were re-incubated for 24 h. The viability of the cells cultured with each extraction medium was finally determined by a 3-(4,5-dimethylthiazol-2-yl)-2,5-diphenyltetrazolium bromide (MTT) assay (see ESI†). The viability of cells cultured with fresh SFM was used as a control.
The cell morphology on the hydrogels was observed with a LEO 1450 VP scanning electron microscope (Carl Zeiss, Germany). Firstly, the thermosensitive CS/β-GP and CS/SS/β-GP hydrogels loaded with LE and without LE were incubated at 37 °C for 3 h and then sterilized under UV radiation for 1 h. MC3T3-E1 (4th passage) cells were seeded onto the hydrogels at a density of 3 × 104 cells per well and then cultured in a 24-well TCPS plate (SPL Life Science, Korea) for 24 h to enable cell attachment. After 24 h, the culture medium in each well plate was removed and each sample was washed with PBS twice. The cells cultured on the samples were then fixed with 3% (v/v) glutaraldehyde (Sigma-Aldrich, USA) for 30 min before dehydration. Dehydration of the samples was performed by a graded series of ethanol concentrations (30, 50, 70, 90, and 100%, v/v) for ∼2 min each. The samples were immersed in 100% hexamethyldisilazane (HMDS; Sigma-Aldrich, USA) for ∼5 min and then dried in air after the removal of HMDS at room temperature for 24 h. Finally, the samples were mounted on SEM stubs and coated with gold before SEM observations were performed.
2.11 Statistical analysis
The results were presented as the mean ± standard error of mean. Statistical analysis was carried out by one-way analysis of variance (one-way ANOVA) and Tukey's test in SPSS (IBM SPSS, USA). Statistical significance was accepted at a value of p < 0.05.
3 Results and discussion
3.1 Longan seed extraction
Rangkadilok et al.15 and Soong et al.16 reported that higher contents of polyphenolic compounds (such as corilagin, GA, and EA) were found in the seeds than in the fruit or pulp of longan. Hence, in this study longan seeds were used to extract polyphenolic compounds. Different fractions of water and ethanol were used to extract polyphenolic compounds in seeds of the longan cultivar Edor. Table 1 shows the percentage yields of LE and the contents of GA and EA in LE produced using different fractions of water and ethanol. The results show that the percentage yields of LE produced using different fractions of water and ethanol were ∼26–56%. The amounts of GA in LE from different fractions were ∼4–13 mg g−1, whereas the amounts of EA were ∼15–26 mg g−1. However, the amounts of GA (∼13 mg g−1) and EA (∼26 mg g−1) produced by solvent extraction using a water to ethanol ratio of 100
:
0 were the highest in comparison with the other fractions. Hence, LE produced by solvent extraction using a water to ethanol ratio of 100
:
0 was selected for further study and used for loading into the thermosensitive hydrogels as a model drug.
Table 1 Percentage yields of LE and contents of GA and EA in LE produced using mixtures with different fractions of water and ethanol
Extraction solvent |
Weight of extract (g) |
% yield |
GA (mg g−1) |
EA (mg g−1) |
Water : ethanol (100 : 0) |
0.0519 |
25.95 |
13.19 |
26.13 |
Water : ethanol (70 : 30) |
0.1120 |
56.00 |
9.47 |
25.94 |
Water : ethanol (50 : 50) |
0.0968 |
48.40 |
7.05 |
15.27 |
Water : ethanol (30 : 70) |
0.1047 |
52.35 |
6.16 |
21.68 |
Water : ethanol (0 : 100) |
0.0928 |
46.40 |
3.62 |
18.52 |
3.2 Determination of antioxidant activity and cytotoxicity of longan seed extract
The polyphenolic compounds (e.g., GA and EA) in LE are known to have the most powerful antioxidant properties because of their free radical scavenging activity and their specific chemical structure.19 In this study, a DPPH˙ radical scavenging assay was used to measure the antioxidant activity of LE. DPPH˙ radicals were converted into DPPH–H (diphenylpicrylhydrazine) molecules in the presence of the antioxidant compounds that were found in LE by the donation of an electron or a hydrogen atom. When the antioxidant compounds were combined with a DPPH solution, the color was changed from the purple color of DPPH˙ radicals to pale yellow owing to the disappearance of DPPH˙ radicals. The absorption maximum of DPPH˙ radicals in methanol was measured with a spectrophotometer at 517 nm. It has been reported that a decrease in the absorbance of DPPH˙ radicals is caused by antioxidant compounds.20 The antioxidant activities of LE in various concentrations (5–2500 μg mL−1) and ascorbic acid are shown in ESI Fig. S1.† The results show that the percentage antioxidant activity of LE increased from approximately 26% to 86% with an increase in the concentration of LE in the range of 5–2500 μg mL−1, whereas ascorbic acid, as a standard antioxidant compound, displayed an increase in antioxidant activity from approximately 33% to 95%. The DPPH˙ radical scavenging effect increased with an increase in the concentration of the active compounds. The antioxidant activities of both LE and ascorbic acid were high in the concentration range between 20 and 2500 μg mL−1. Moreover, the half-maximum inhibitory concentration (IC50) of LE was 8.90 μg mL−1, which was close to that of the standard compound ascorbic acid (IC50 = 6.76 μg mL−1). It has been reported that the polyphenolic compounds in LE have strong antioxidant activity, although this is slightly weaker than that of ascorbic acid. According to these results, LE might accelerate the healing of fractured bones by suppressing the inflammatory and destructive effects of free radicals on whole-body systems.21
The viability of NCTC clone 929 cells was determined using different concentrations of LE, and cell activity was measured using an REMA,22 as shown in ESI Fig. S2.† The results showed that the LE concentrations (3.13–100 μg mL−1) used in this assay did not exhibit any cytotoxic effect on NCTC clone 929 cells. These results suggested that LE had no toxic effects on cell survival and growth.
3.3 Gelation time of thermosensitive CS/β-GP hydrogels
In the CS/β-GP system, the addition of β-GP, as a weak base, to an acidic CS solution neutralizes the system from a pH of 4.0 to a pH of 7.0, which is physiologically acceptable. Chenite et al.7 and Cho et al.23 found that the mechanisms of gelation in CS/β-GP systems might be explained by the following factors: hydrogen bonding between CS chains, electrostatic interactions, and hydrophobic interactions involving the main molecular forces in gel formation. The several effective interactions responsible for the sol-to-gel transition can be briefly described as follows: (1) the addition of β-GP to a CS solution increases the ionic strength, which leads to a reduction in electrostatic repulsion between CS chains, and this can induce an increase in inter-chain hydrogen bonding in CS; (2) an electrostatic attraction occurs between the oppositely charged ammonium groups in CS and phosphate groups in β-GP; and (3) the hydrophobic or water-structuring character of the glycerol moiety in β-GP can enhance the hydrophobic interactions between CS molecules. In this study, the influences of the CS concentration and the volume ratio of CS to β-GP on the gelation time were studied. The gelation time of the thermosensitive CS/β-GP hydrogels at 37 °C was determined by a simple test tube inversion method. The hydrogel was a flowable viscous solution in the range of 4–25 °C. After incubation at 37 °C for a period, a sol–gel transition was observed, and the hydrogel was transformed into a non-flowing gel. The gelation times of thermosensitive CS/β-GP hydrogels prepared using different parameters are shown in Table 2. The concentration of CS in the range from 3.0% to 4.0% (w/v) and the volume ratio of CS to β-GP (i.e., 0.7
:
0.3, 0.5
:
0.5 and 0.3
:
0.7 mL) had a strong effect on the gelation time. A volume ratio of 0.7
:
0.3 mL of CS to β-GP in each set of conditions resulted in a prolonged gelation time; in particular, at a concentration of 3.0% (w/v) CS a period of approximately 20 h was required to form a gel. In contrast, the addition of a large amount of β-GP caused the gelation time to decrease. A gel was formed within 10 min under the following conditions: a 3.0% (w/v) CS solution at a CS: β-GP volume ratio of 0.3
:
0.7 mL, a 3.5% (w/v) CS solution at a CS: β-GP volume ratio of 0.3
:
0.7 or 0.5
:
0.5 mL, and a 4.0% (w/v) CS solution at a CS: β-GP volume ratio of 0.3
:
0.7 or 0.5
:
0.5 mL. Although a CS: β-GP volume ratio of 0.3
:
0.7 mL at all CS concentrations resulted in a short gelation time, the hydrogels were deformed after gelation because of their high contents of β-GP. In the case of a 4.0% (w/v) CS solution at a CS: β-GP volume ratio of 0.5
:
0.5 mL, the hydrogel did not exhibit high fluidity because the solution had high viscosity, which resulted in difficulties with injection. Therefore, the optimal formulation comprised a 3.5% (w/v) CS solution and a CS: β-GP volume ratio of 0.5
:
0.5 mL.
Table 2 Preparation parameters and gelation times of the thermosensitive CS/β-GP hydrogels
Concentration of CS (%, w/v) |
CS : β-GP (mL) |
pH |
Gelation time at 37 °C (min) |
p < 0.05 in comparison with the thermosensitive CS/β-GP hydrogels prepared from a 3.0% (w/v) solution of CS. |
3.0 |
0.7 : 0.3 |
7.04 ± 0.06 |
1207.00 ± 30.05 |
0.5 : 0.5 |
7.57 ± 0.00 |
52.83 ± 1.89 |
0.3 : 0.7 |
7.86 ± 0.02 |
7.82 ± 0.76 |
3.5 |
0.7 : 0.3 |
7.24 ± 0.01 |
388.22 ± 29.50a |
0.5 : 0.5 |
7.64 ± 0.01 |
9.97 ± 0.09a |
0.3 : 0.7 |
7.88 ± 0.05 |
6.69 ± 0.44 |
4.0 |
0.7 : 0.3 |
7.35 ± 0.01 |
30.29 ± 0.62a |
0.5 : 0.5 |
7.79 ± 0.00 |
4.01 ± 0.15a |
0.3 : 0.7 |
8.14 ± 0.01 |
2.58 ± 0.08a |
The stability of the thermosensitive hydrogels at different pH values (4, 7, and 10) of the medium at 37 °C is shown in Table S1.† The concentration of CS was fixed at 3.5% (w/v), and the volume ratio of CS to β-GP was fixed at 0.5
:
0.5 mL. The results demonstrated that the thermosensitive CS/β-GP hydrogels formed under all conditions were in a liquid form at room temperature and a gel form at 37 °C. The thermosensitive CS/β-GP hydrogels at a pH of 4 exhibited a longer gelation time (∼32 min). However, an increase in the pH of the medium caused the gelation time to decrease. The gelation times of the thermosensitive CS/β-GP hydrogels at pH value of 7 and 10 were ∼10 min and ∼5 min, respectively. As the pH of the medium increased, the pH of the CS solution also increased. Thus, an increase in the pH of the hydrogel system caused the electrostatic interactions and hydrogen bonding between polymer chains to increase, which resulted in a decrease in the gelation time.
3.4 Gelation time of thermosensitive CS/SS/β-GP hydrogels
The gelation times of the thermosensitive CS/SS/β-GP hydrogels prepared using different SS concentrations are shown in Table 3. The gelation times of the thermosensitive CS/SS/β-GP hydrogels were shorter than those of the CS/β-GP hydrogels. The gelation time of the hydrogels displayed a decreasing trend with an increase in the SS concentration from 1.5% to 3.5% (w/v). The reason might be that the SS molecules incorporated into the CS/β-GP solution increased the attractive forces between neighboring macromolecular chains, which thereby reduced the gelation time. At a low temperature, hydrogen bonds were formed in the hydrogels between the amino groups of CS and SS, and SS also formed hydrogen bonds with water molecules. When the temperature was increased, the intermolecular hydrogen bonding interactions decreased. Therefore, the energized water molecules that surrounded the molecular chains were removed and the hydrophobic molecules in the CS and SS chains were highly entangled with each other. Thus, it was possible that the addition of SS to the CS/β-GP hydrogel enhanced the hydrophobic interactions, which were the main driving force for the formation of a gel and the reduction in the time required for gel formation at a high temperature. Fig. 1 also confirms that the thermosensitive CS/SS3/β-GP hydrogels had thermosensitive properties at 37 °C and did not revert to their original state at 25 °C.
Table 3 Preparation parameters and gelation times of the thermosensitive CS/SS/β-GP hydrogels
Sample code |
CS (%, w/v) |
SS (%, w/v) |
β-GP (%, w/v) |
CS : β-GP (mL) |
Gelation time at 37 °C (min) |
p < 0.05 in comparison with the thermosensitive CS/β-GP hydrogels. |
CS/β-GP |
3.5 |
— |
50 |
0.5 : 0.5 |
9.97 ± 0.09 |
CS/SS1/β-GP |
3.5 |
1.5 |
50 |
0.5 : 0.5 |
6.91 ± 0.08a |
CS/SS2/β-GP |
3.5 |
2.5 |
50 |
0.5 : 0.5 |
6.69 ± 0.15a |
CS/SS3/β-GP |
3.5 |
3.5 |
50 |
0.5 : 0.5 |
5.59 ± 0.24a |
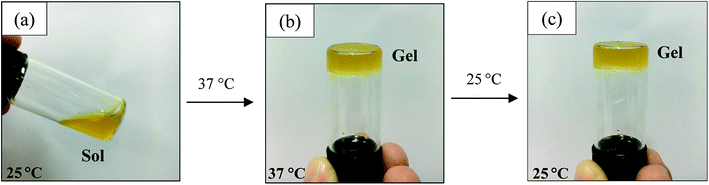 |
| Fig. 1 Photographs of the sol–gel transition of a CS/SS3/β-GP solution from a sol state at 25 °C (a) to a gel state at 37 °C (b) and the gel state at 25 °C after the sol–gel transition (c). | |
3.5 Rheological properties
The effects of the gelation temperature of the thermosensitive CS/β-GP and CS/SS/β-GP hydrogels on the elastic (storage) modulus (G′) and viscous (loss) modulus (G′′) were determined using a rotational rheometer. When the temperature was increased from 20 °C to 50 °C, the hydrogels prepared under all conditions exhibited a rapid increase in the storage modulus (G′), which was explained by the phase transition from a sol to a gel around 35–39 °C (Fig. 2). The growth rate of the elastic modulus (G′) was higher than that of the viscous modulus (G′′) (G′ > G′′) beyond the gelling point, which indicates that the development of a gel structure contributed to an increase in the elasticity or gel strength of the hydrogels.24 The elastic modulus of the thermosensitive CS/β-GP hydrogel exceeded 30 Pa at 45 °C beyond the gelling point, but the elastic modulus of the thermosensitive CS/SS/β-GP hydrogels prepared under all conditions remained below 30 Pa at 45 °C, whereas the thermosensitive CS/SS/β-GP hydrogels had lower strength, which might have been due to the weaker hydrophobic interactions between CS chains. Moreover, the crossover of the elastic modulus (G′) and the loss modulus (G′′) moved to a lower temperature, which indicates that the gelation temperature was reduced by the incorporation of SS. The crossover point (where G′ and G′′ are equal) of the thermosensitive CS/β-GP hydrogel occurred at a higher temperature (39 °C) than that of the thermosensitive CS/SS/β-GP hydrogels (∼35–38 °C). Because the SS molecules incorporated into the CS/β-GP solution caused the molecular interactions to increase, the gelation temperature was reduced. Hence, from these results it might be concluded that the addition of SS to the hydrogels had an effect on the gelation temperature.
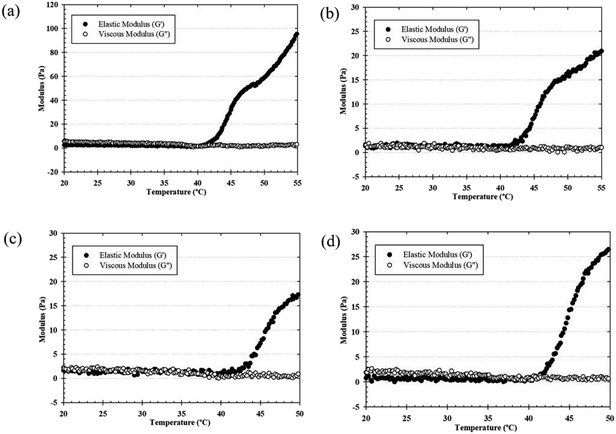 |
| Fig. 2 Typical temperature dependence of G′ and G′′ values of the thermosensitive CS/β-GP (a), CS/SS1/β-GP (b), CS/SS2/β-GP (c) and CS/SS3/β-GP (d) hydrogels. | |
3.6 Viscosity measurements
Injectable hydrogels based on thermosensitive polymers have interesting properties for tissue engineering. However, thermosensitive hydrogels should be strong enough to produce gels with sufficient stability for the proposed use. To prove the enhancement in the sol–gel transition behavior of the thermosensitive hydrogels, the values of the viscous modulus (Pa s) of the thermosensitive CS/β-GP and CS/SS/β-GP hydrogels were measured as a function of temperature. As shown in Fig. 3, the maximum value of the viscous modulus of the thermosensitive CS/β-GP hydrogel was higher than those of the thermosensitive CS/SS/β-GP hydrogels prepared under all conditions. However, the phase transition temperature (37 °C) of the thermosensitive CS/SS/β-GP hydrogels prepared under all conditions was slightly lower than that of the thermosensitive CS/β-GP hydrogel. These results indicated that the addition of SS to the hydrogels did not enhance their viscoelastic properties. This might be attributed to the expected electrostatic interaction between SS and CS chains. The electrostatic interactions of the amine (–NH2) and hydroxyl (–OH) groups in SS and the electron-rich oxygen atoms in the –NH2/–OH groups in CS caused a reduction in charge density on the CS chains. Because the number of charged ammonium groups on the CS chains was an important parameter that controlled the gelation of the system, the addition of SS to the hydrogels caused a decrease in their viscoelastic properties, which was due to a decline in the interaction between the ammonium groups in CS and the phosphate groups in β-GP.
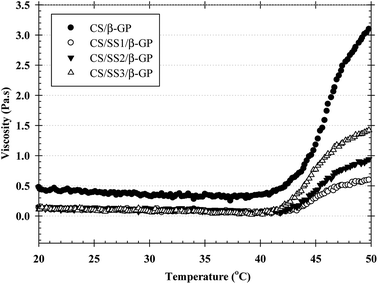 |
| Fig. 3 Solution viscosity of the CS/β-GP and CS/SS/β-GP hydrogels as a function of temperature. | |
3.7 FTIR analysis
FTIR spectroscopy was used to identify the interactions between the components of CS, SS, and β-GP. The FTIR spectra of the samples are shown in Fig. 4. All the samples exhibited broad absorption bands around 3600 and 3000 cm−1. These bands indicated the occurrence of O–H stretching and N–H stretching in the structures. The peaks in the range of 1685–1657 cm−1 were attributed to C
O stretching in amide I, and those at 1597–1459 cm−1 were assigned to N–H bending in amide II in CS and SS. Moreover, the spectra of CS and SS also displayed peaks at 1400–1200 cm−1, which were assigned to an in-phase combination of N–H in-plane bending and C–N stretching vibrations in amide III.25 In the case of the thermosensitive CS/β-GP and CS/SS3/β-GP hydrogels, the FTIR spectra displayed peaks at 3600–3000 cm−1, which indicated O–H stretching. Peaks due to the inorganic phase of the PO43− groups were observed at 970 and 750 cm−1. The peaks due to N–H and O–H stretching in CS shifted after the formation of the gel. This might indicate bonding between N–H groups in CS and O–H groups in β-GP or hydrogen bonding between C
O groups in CS and O–H groups in β-GP. In addition, the peaks due to amides I, II and III in CS and SS decreased because hydrogen bonds and a chemical complex were formed between amino groups in CS or SS and OH and PO43− groups in β-GP. In addition, the peak due to C–CH3 symmetric deformation decreased in the spectra of the hydrogels, which indicated that H bonds might have been formed. Obviously, hydrogen bonding and electrostatic interactions existed between CS and β-GP.26
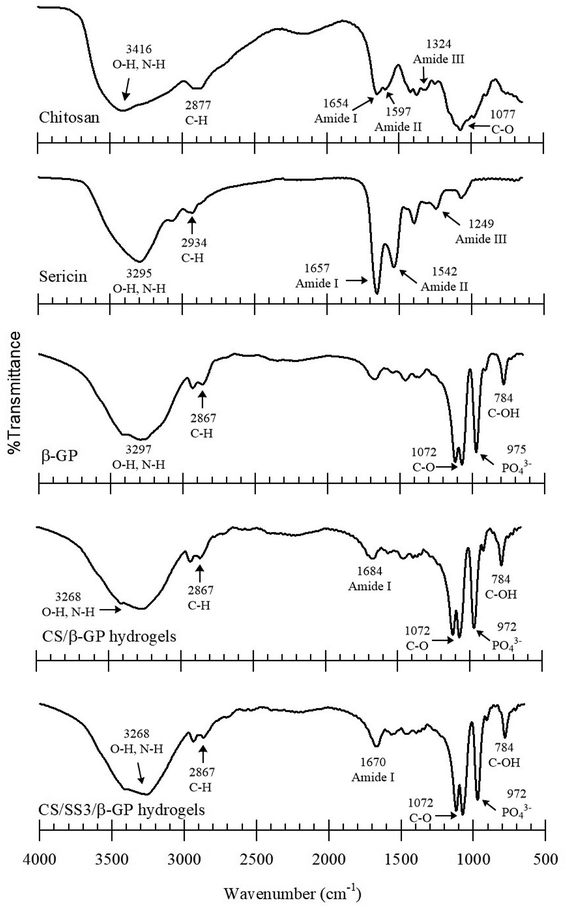 |
| Fig. 4 FTIR spectra of CS, SS, β-GP, CS/β-GP hydrogel, and CS/SS3/β-GP hydrogel. | |
3.8 Scanning electron microscopy
SEM images of thermosensitive CS/β-GP and CS/SS3/β-GP hydrogels that were lyophilized at least 24 h before the observations are shown in Fig. 5. The morphological appearance of the thermosensitive CS/β-GP hydrogel exhibited an irregular pore structure, whereas the morphological appearance of the CS/SS/β-GP hydrogel became more slender and porous. It has been reported that three-dimensional hydrogels with irregular porous structures can encourage the entrance of water or biological fluids into the hydrogel structures and support the diffusion of drugs out of the hydrogels.27
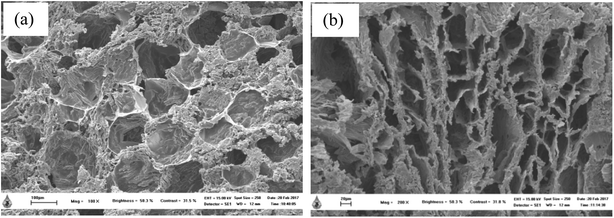 |
| Fig. 5 SEM images of thermosensitive (a) CS/β-GP (scale bar = 100 μm) and (b) CS/SS3/β-GP (scale bar = 20 μm) hydrogels. | |
3.9 Weight loss behavior of hydrogels
The weight loss behavior of the thermosensitive CS/β-GP and CS/SS/β-GP hydrogels was examined under simulated physiological conditions at 37 °C. The weight loss of the thermosensitive hydrogels in PBS (pH 7.4) after 1, 3, 5, 7, and 10 days is shown in Fig. 6. After 1 day, the weight loss of the thermosensitive CS/β-GP, CS/SS1/β-GP, CS/SS2/β-GP, and CS/SS3/β-GP hydrogels was approximately 39%, 31%, 38%, and 36%, respectively, whereas after 3 days this value significantly increased to approximately 42%, 53%, 54%, and 55%, respectively. After 5 days, the value was approximately 56%, 58%, 60%, and 58%, respectively. After 7 days, the value was approximately 57%, 56%, 58%, and 61%, respectively. After 10 days, the value was approximately 63%, 58%, 64%, and 66%, respectively. According to these results, the weight loss of hydrogels prepared under all conditions was fairly high in the medium after an immersion time of 3 days and rather stable after an immersion time of 5–7 days. This indicated that water molecules diffused into the hydrogel rapidly during the initial 3 days and the maximum water absorption reached equilibrium after an immersion time of 3 days. In addition, the hydrogels containing SS exhibited a significantly higher weight loss after 3 days owing to the high water solubility of SS, which led to the breakup of polymer linkages and an increase in the degradation of the hydrogels. Therefore, the incorporation of SS affected the weight loss of these thermosensitive hydrogels and had the potential to enhance the availability of drugs at the desired site.
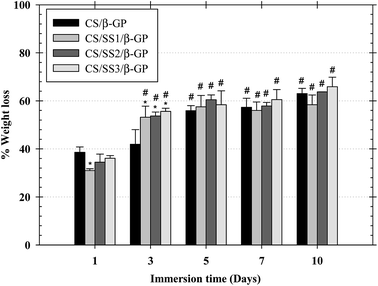 |
| Fig. 6 Weight loss behavior of the thermosensitive CS/β-GP and CS/SS/β-GP hydrogels in a PBS medium (n = 3). *p < 0.05 in comparison with the thermosensitive CS/β-GP hydrogel at any given time point, and #p < 0.05 in comparison with an immersion time of 1 day for any given type of hydrogel. | |
3.10 In vitro degradation of hydrogels
The in vitro degradation of the thermosensitive CS/β-GP and CS/SS/β-GP hydrogels was investigated by measuring the loss of free amino groups in PBS (pH 7.4) and PBS containing α-amylase (pH 6.8) at 37 °C. The amounts of free amino acid groups were measured by a ninhydrin assay. The cumulative amounts of free amino acids released from the hydrogels over a period of up to 2 weeks are presented in Fig. 7. The results showed that the thermosensitive CS/β-GP hydrogel exhibited a lower degradation rate than those prepared under other conditions in both PBS and PBS containing α-amylase. The incorporation of SS affected the network structure of the CS hydrogel, which caused it to degrade faster. These results suggested that SS might interfere with the polymeric network of CS and reduce the intermolecular interactions between CS and β-GP. Thus, water molecules might easily diffuse into the hydrogel matrix, which would lead to the loss of amino groups from the polymer chain. In addition, the morphological appearances of hydrogels with and without SS exhibited different pore structures. The difference in pore structure was due to the incorporation of SS into the thermosensitive CS/β-GP hydrogels, as shown in Fig. 5. According to the results, the morphology of the thermosensitive CS/β-GP hydrogels exhibited a less porous structure, whereas the thermosensitive CS/SS/β-GP hydrogels exhibited more porous structures at a high SS concentration. Because the morphology of the materials plays a significant role in the degradation of hydrogels, the thermosensitive CS/SS/β-GP hydrogels with high porosity gave rise to a higher diffusion rate of water than those with low porosity. Thus, it can be concluded that the loss of free amino acid groups from the thermosensitive hydrogels was influenced by a combination of diffusion through pores and degradation of the polymer matrix.
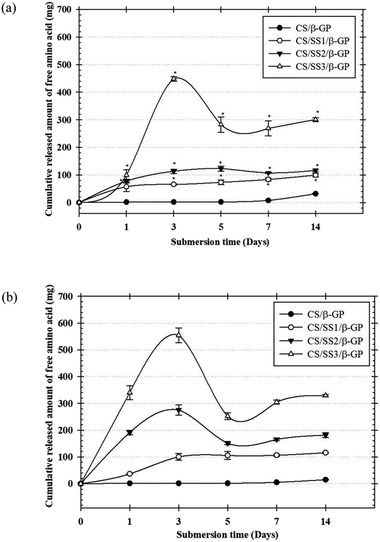 |
| Fig. 7 In vitro degradation of the thermosensitive CS/β-GP and CS/SS/β-GP hydrogels in PBS (a) and PBS containing α-amylase (b). The amounts of free amino groups were determined as a function of the degradation time by a ninhydrin assay at 570 nm (n = 3). *p < 0.05 in comparison with the thermosensitive CS/β-GP hydrogels at a given time point. | |
3.11 In vitro release of longan seed extract from hydrogels
The profiles of the in vitro release of both GA and EA from the thermosensitive CS/β-GP and CS/SS/β-GP hydrogels loaded with LE were investigated as a function of time. Fig. 8 shows the profiles of the cumulative release of both GA and EA from the hydrogels over a period of 7 days. The profiles of the cumulative release of both drugs from all the hydrogels are presented in terms of the weight of the drug released (in mg) divided by the actual weight of LE (in mg). The results showed that the cumulative amounts of both drugs released from the thermosensitive CS/β-GP hydrogels loaded with LE gradually increased during the first immersion period, increased more gradually afterward, and then reached a plateau during the longest immersion period. On the other hand, the cumulative amounts of both drugs released from the thermosensitive CS/SS/β-GP hydrogels greatly increased during the first immersion time, increased substantially afterward, and then reached a plateau at the end of the observation period. Specifically, the maximum cumulative amounts of both GA and EA released from the thermosensitive CS/SS3/β-GP hydrogel were significantly higher than those released from the other hydrogels. Hence, the release of large amounts of phenolic compounds from the thermosensitive CS/SS3/β-GP hydrogel loaded with LE could promote the bone healing process.18,21 In addition, higher cumulative amounts of GA were released from these hydrogels after immersion in the medium in comparison with the released amounts of EA. This could be explained by the fact that GA has higher solubility in water than EA because EA contains four phenolic groups and two lactone groups, which result in poor water solubility.28 According to the results, the cumulative amounts of both drugs released from the thermosensitive CS/β-GP hydrogel loaded with LE were lower than those released from the thermosensitive CS/SS/β-GP hydrogels loaded with LE. These results suggested that SS might interfere with the polymeric network of CS and reduce the intermolecular interactions between CS and β-GP. Therefore, water molecules might easily diffuse into the hydrogel matrix, which would lead to the degradation of the hydrogel and an increase in the released amounts of drugs. Hence, the behavior of the drugs in terms of release from the hydrogels might be due to the degradation of the hydrogels, according to the results of in vitro degradation.
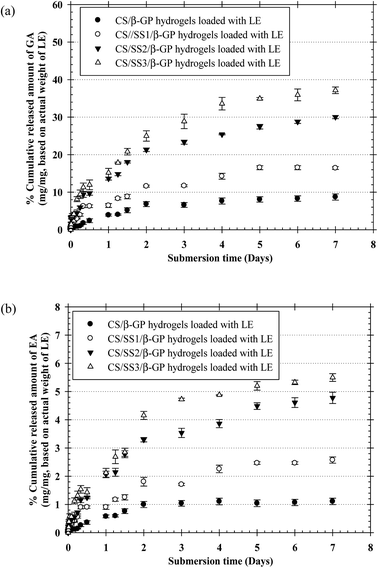 |
| Fig. 8 Profiles of the cumulative release of GA (a) and EA (b) from the thermosensitive CS/β-GP and CS/SS/β-GP hydrogels loaded with LE reported as the percentage of the weight of GA or EA released divided by the actual weight of LE in the hydrogels and determined by total immersion in a PBS medium at 37 °C (n = 3). | |
3.12 Investigation of indirect cytotoxicity and cell attachment of hydrogels
The biocompatibility of injectable polymeric biomaterials is an important consideration for bone tissue engineering, because the viability of cells should be maintained during gel formation.29 CS is derived from a naturally occurring polymer and is known to be a biomaterial because of its ability to stimulate cell growth and interactions between cells and the extracellular matrix (ECM).30 Therefore, naturally derived biomaterials exhibit excellent biocompatibility, promote cell attachment and can support cell growth with high levels of viability and proliferation of cells.31 To evaluate the potential of the thermosensitive hydrogels loaded with LE for use in bone tissue engineering applications, an investigation of the indirect cytotoxicity of these hydrogels was carried out. The viability of NCTC clone 929 and MC3T3-E1 cells cultured with the extraction media from the hydrogels after incubation for 24 h was determined by an MTT assay, and the results are shown in Fig. 9. Clearly, the viability of NCTC clone 929 cells cultured with all the extraction media from all the thermosensitive hydrogels ranged between ∼86% and ∼118%, whereas the viability of MC3T3-E1 cells ranged between ∼78% and ∼97%. The ISO 10993–5 standard for in vitro cytotoxicity states that “reduction of cell viability by more than 30% is considered a cytotoxic effect”. The viability of cells cultured with the extraction media from all the hydrogels was greater than 70% after treatment for 24 h. These results indicated that these hydrogels were non-toxic to both NCTC clone 929 and MC3T3-E1 cells.
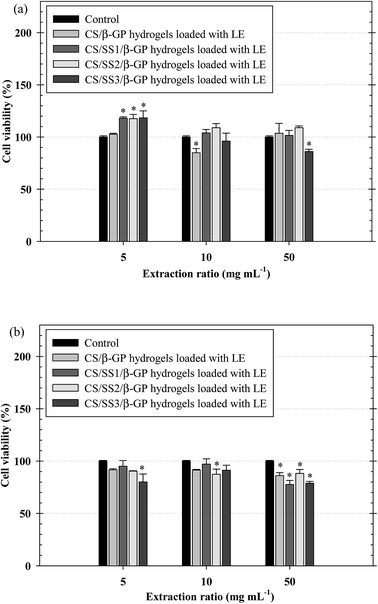 |
| Fig. 9 Investigation of the indirect cytotoxicity of thermosensitive CS/β-GP and CS/SS/β-GP hydrogels loaded with LE (n = 3) cultured with NCTC clone 929 (a) and MC3T3-E1 (b) cells. The viability of cells cultured with fresh SFM was used as a control. *p < 0.05 in comparison with the control. | |
The morphology of MC3T3-E1 cells cultured on the thermosensitive hydrogels was observed by SEM. SEM images of attached MC3T3-E1 cells on the thermosensitive CS/β-GP and CS/SS/β-GP hydrogels loaded with LE and without LE in comparison with a cover glass used as a control after cell culture for 24 h are presented in Fig. 10. The results show that MC3T3-E1 cells were attached to the cover glass and exhibited a round shape. However, the morphology of the attached MC3T3-E1 cells exhibited better adhesion and a flatter shape on the surfaces of all the thermosensitive hydrogels in comparison with the cover glass. Moreover, the attachment behavior of MC3T3-E1 cells exhibited a typical elongated and spindle-like morphology on the thermosensitive hydrogels loaded with LE. Therefore, the thermosensitive hydrogels loaded with LE have the potential for use in bone tissue engineering applications.
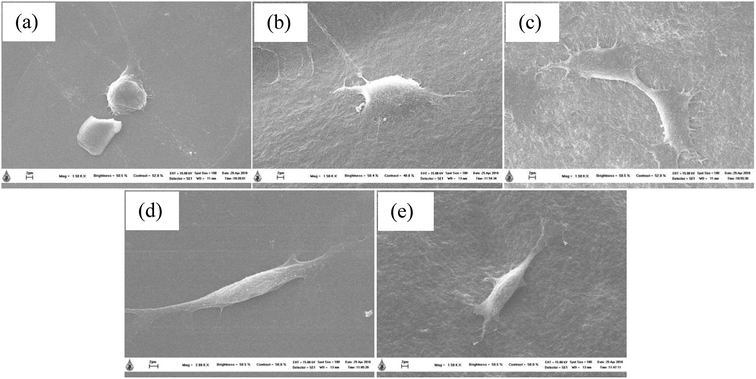 |
| Fig. 10 Morphology and adhesion behavior of MC3T3-E1 cells on a cover glass (a), thermosensitive CS/β-GP hydrogel without LE (b), CS/SS1/β-GP hydrogel without LE (c), CS/β-GP hydrogel loaded with LE (d), and CS/SS1/β-GP hydrogel loaded with LE (e) after culture for 24 h. | |
4 Conclusions
Injectable thermosensitive CS/β-GP and CS/SS/β-GP hydrogels loaded with LE were successfully fabricated for use in bone tissue engineering applications. The thermosensitive CS/β-GP hydrogels were transformed from a sol into a gel at 37 °C. Different ratios of CS to β-GP were employed to determine the optimal conditions for the preparation of the hydrogels. The optimal conditions comprised a 3.5% (w/v) CS solution and a volume ratio of CS to β-GP of 0.5
:
0.5 mL. The incorporation of SS into the CS/β-GP solution resulted in a shorter gelation time of 5–7 min. The morphological structure of the thermosensitive CS/β-GP hydrogel exhibited an irregular pore structure, whereas the thermosensitive CS/SS/β-GP hydrogels became more slender and porous. The weight loss of the thermosensitive CS/β-GP and CS/SS/β-GP hydrogels was greater than 50% after 3 days. The incorporation of SS affected the network structure of the CS hydrogels, which caused them to degrade more rapidly in both PBS and PBS containing α-amylase. Moreover, the cumulative amounts of both GA and EA released from the thermosensitive hydrogels loaded with LE increased with an increase in the SS content. The fact that greater cumulative amounts of phenolic compounds were released from the thermosensitive CS/SS3/β-GP hydrogel loaded with LE implies that this hydrogel has potential for the restoration of functional bone tissue. Finally, the thermosensitive hydrogels loaded with LE were non-toxic to both NCTC clone 929 and MC3T3-E1 cells and also promoted the attachment of MC3T3-E1 cells to the surface of materials. Hence, the thermosensitive hydrogels loaded with LE might be promising materials for use in bone tissue engineering applications.
Conflicts of interest
There are no conflicts to declare.
Acknowledgements
The authors would like to thank the Scientific and Technological Instruments Center (STIC) and Mae Fah Luang University. Porntipa Pankongadisak gratefully acknowledges the Royal Golden Jubilee PhD scholarship (PHD/0094/2558), Thailand Research Fund (TRF).
References
- B. Jeong, S. W. Kim and Y. H. Bae, Thermosensitive sol-gel reversible hydrogels, Adv. Drug Delivery Rev., 2012, 64, 154 CrossRef.
- C. Gong, T. Qi, X. Wei, Y. Qu, Q. Wu, F. Luo and Z. Qian, Thermosensitive polymeric hydrogels as drug delivery systems, Curr. Med. Chem., 2013, 20, 79 CrossRef CAS PubMed.
- L. Wang and J. P. Stegemann, Thermogelling chitosan and collagen composite hydrogels initiated with β-glycerophosphate for bone tissue engineering, Biomaterials, 2010, 31, 3976 CrossRef CAS PubMed.
- Z. Li, Z. Fan, Y. Xu, W. Lo, X. Wang, H. Niu, X. Li, X. Xie, M. Khan and J. Guan, pH-sensitive and thermosensitive hydrogels as stem-cell carriers for cardiac therapy, ACS Appl. Mater. Interfaces, 2016, 8, 10752 CrossRef CAS PubMed.
- E. Ruel-Gariépy and J.-C. Leroux, In situ forming hydrogels-review of temperature-sensitive systems, Eur. J. Pharm. Biopharm., 2004, 58, 409 CrossRef PubMed.
- L. Liu, Q. Gao, X. Lu and H. Zhou, In situ forming hydrogels based on chitosan for drug delivery and tissue regeneration, Asian J. Pharm. Sci., 2016, 11, 673 CrossRef.
- A. Chenite, C. Chaput, D. Wang, C. Combes, M. D. Buschmann, C. D. Hoemann, J. C. Leroux, B. L. Atkinson, F. Binette and A. Selmani, Novel injectable neutral solutions of chitosan form biodegradable gels in situ, Biomaterials, 2000, 21, 2155 CrossRef CAS PubMed.
- F. G. Tahrir, F. Ganji and T. M. Ahooyi, Injectable thermosensitive chitosan/glycerophosphate-based hydrogels for tissue engineering and drug delivery applications: a review, Recent Pat. Drug Delivery Formulation, 2015, 9, 107 CrossRef CAS.
- B. C. Dash, B. B. Mandal and S. C. Kundu, Silk gland sericin protein membranes: fabrication and characterization for potential biotechnological applications, J. Biotechnol., 2009, 144, 321 CrossRef CAS PubMed.
- N. Kato, S. Sato, A. Yamanaka, H. Yamada, N. Fuwa and M. Nomura, Silk protein, sericin, inhibits lipid peroxidation and tyrosinase activity, Biosci., Biotechnol., Biochem., 1998, 62, 145 CrossRef CAS PubMed.
- R. Rajendran, C. Balakumar, R. Sivakumar, T. Amruta and N. Devaki, Extraction and application of natural silk protein sericin from Bombyx mori as antimicrobial finish for cotton fabrics, J. Text. Inst., 2012, 103, 458 CrossRef CAS.
- Y. Tamada, M. Sano, K. Niwa, T. Imai and G. Yoshino, Sulfation of silk sericin and anticoagulant activity of sulfated sericin, J. Biomater. Sci., Polym. Ed., 2004, 15, 971 CrossRef CAS PubMed.
- Y. Q. Zhang, Applications of natural silk protein sericin in biomaterials, Biotechnol. Adv., 2002, 20, 91 CrossRef CAS PubMed.
- S. Kapoor and S. C. Kundu, Silk protein-based hydrogels: promising advanced materials for biomedical applications, Acta Biomater., 2016, 31, 17 CrossRef CAS PubMed.
- N. Rangkadilok, L. Worasuttayangkurn, R. N. Bennett and J. Satayavivad, Identification and quantification of polyphenolic compounds in Longan (Euphoria longana Lam.) fruit, J. Agric. Food Chem., 2005, 53, 1387 CrossRef CAS PubMed.
- Y. Y. Soong and P. J. Barlow, Isolation and structure elucidation of phenolic compounds from longan (Dimocarpus longan Lour.) seed by high-performance liquid chromatography-electrospray ionization mass spectrometry, J. Chromatogr. A, 2005, 1085, 270 CrossRef CAS PubMed.
- N. Rangkadilok, S. Sitthimonchai, L. Worasuttayangkurn, C. Mahidol, M. Ruchirawat and J. Satayavivad, Evaluation of free radical scavenging and antityrosinase activities of standardized longan fruit extract, Food Chem. Toxicol., 2007, 45, 328 CrossRef CAS PubMed.
- M. M. J. Al-Obaidi, F. H. Al-Bayaty, R. Al Batran, P. Hassandarvish and E. Rouhollahi, Protective effect of ellagic acid on healing alveolar bone after tooth extraction in rat-A histological and immunohistochemical study, Arch. Oral Biol., 2014, 59, 987 CrossRef CAS PubMed.
- G. Jiang, L. Wen, F. Chen, F. Wu, S. Lin, B. Yang and Y. Jiang, Structural characteristics and antioxidant activities of polysaccharides from longan seed, Carbohydr. Polym., 2013, 92, 758 CrossRef CAS PubMed.
- M. S. Blois, Antioxidant determinations by the use of a stable free radical, Nature, 1958, 181, 1199 CrossRef CAS.
- S. A. Sheweita and K. I. Khoshhal, Calcium metabolism and oxidative stress in bone fractures: role of antioxidants, Curr. Drug Metab., 2007, 8, 519 CrossRef CAS.
- J. O'Brien, I. Wilson, T. Orton and F. Pognan, Investigation of the alamar blue (resazurin) fluorescent dye for the assessment of mammalian cell cytotoxicity, Eur. J. Biochem., 2000, 267, 5421 CrossRef.
- J. Cho, M.-C. Heuzey, A. Bégin and P. J. Carreau, Physical gelation of chitosan in the presence of β-glycerophosphate: the effect of temperature, Biomacromolecules, 2005, 6, 3267 CrossRef CAS PubMed.
- A. Chenite, M. Buschmann, D. Wang, C. Chaput and N. Kandani, Rheological characterization of thermogelling chitosan/glycerol-phosphate solutions, Carbohydr. Polym., 2001, 46, 39 CrossRef CAS.
- F. Mirahmadi, M. Tafazzoli-Shadpour, M. A. Shokrgozar and S. Bonakdar, Enhanced mechanical properties of thermosensitive chitosan hydrogel by silk fibers for cartilage tissue engineering, Mater. Sci. Eng., C, 2013, 33, 4786 CrossRef CAS PubMed.
- Z. Modrzejewska, K. Nawrotek, W. Maniukiewicz and T. Douglas, Structural characteristics of thermosensitive chitosan glutamate hydrogels in variety of physiological environments, J. Mol. Struct., 2014, 1074, 629 CrossRef CAS.
- E. P. Azevedo and V. Kumar, Rheological, water uptake and controlled release properties of a novel self-gelling aldehyde functionalized chitosan, Carbohydr. Polym., 2012, 90, 894 CrossRef CAS PubMed.
- I. Bala, V. Bhardwaj, S. Hariharan and M. N. V. Ravi Kumar, Analytical methods for assay of ellagic acid and its solubility studies, J. Pharm. Biomed. Anal., 2006, 40, 206 CrossRef CAS PubMed.
- B. Chang, N. Ahuja, C. Ma and X. Liu, Injectable scaffolds: preparation and application in dental and craniofacial regeneration, Mater. Sci. Eng., R, 2017, 111, 1 CrossRef PubMed.
- I.-Y. Kim, S.-J. Seo, H.-S. Moon, M.-K. Yoo, I.-Y. Park, B.-C. Kim and C.-S. Cho, Chitosan and its derivatives for tissue engineering applications, Biotechnol. Adv., 2008, 26, 1 CrossRef CAS PubMed.
- F. J. O'Brien, Biomaterials & scaffolds for tissue engineering, Mater. Today, 2011, 14, 88 CrossRef.
Footnote |
† Electronic supplementary information (ESI) available. See DOI: 10.1039/c8ra07255h |
|
This journal is © The Royal Society of Chemistry 2018 |
Click here to see how this site uses Cookies. View our privacy policy here.