DOI:
10.1039/C8RA07657J
(Paper)
RSC Adv., 2018,
8, 38935-38940
Efficient visible-light full-color tuning of self-organized helical superstructures enabled by fluorinated chiral switches†
Received
14th September 2018
, Accepted 13th November 2018
First published on 19th November 2018
Abstract
Light-driven chiral switches have the ability to tune and control the self-organized helical superstructures of cholesteric liquid crystals (CLCs), resulting in the photo-induced reflection wavelength shift of the CLCs. A new type of axially chiral switch functionalized with fluorine atoms ortho to the azobenzene moiety is found to exhibit reversible visible-light-driven photoisomerization due to a separation of the n–π* absorption bands of the trans and cis isomers. These chiral switches all have high HTP values and the doped CLCs with 15.8 wt% concentration demonstrates reversible dynamic tuning of the reflection color within the entire visible spectrum driven by 530 nm and 445 nm visible light. It is also noteworthy that the thermal stability is improved thanks to the cis form of the fluorinated azobenzenes possessing a remarkably long half-life. The newly designed visible-light-driven chiral switches may broaden the application of CLCs, especially in the fields where high energy UV light is unfavorable.
Introduction
Cholesteric liquid crystals (CLCs) possessing self-organized helical superstructures1 are a class of materials capable of selectively reflecting light according to Bragg's law. Dynamic tuning of reflection wavelength of the CLCs by external stimuli, including heat,2,3 electric field,4,5 chemical reaction6,7 and light8–16 is of great significance in many applications, such as color filters,17 reflectors,18,19 displays,20,21 lasers,22,23 and sensors.24,25 Among all the stimuli, light wields advantages of remote, spatial and temporary controllability, therefore light-driven CLCs are widely studied.26,27
The commonly used method to obtain light-driven CLCs is to dope a small amount of photoresponsive chiral switches into nematic liquid crystals (LCs).28,29 The ability of a chiral switch to twist the nematic LC into helical superstructures is represented by the helical twisting power (HTP, β) in the equation: β = 1/(pc), where c is the concentration of the chiral switch and p is the pitch length of the helical superstructures. The central wavelength of the reflection λ is related to the pitch, i.e. defined as λ = np, where n is the average refraction index of the LC host. The configurational change of the chiral switches upon light irradiation enables the HTP variation, resulting in the photocontrol of the helices as well as the reflection wavelength.
Azobenzene-based chiral switches draw tremendous attention because of the dramatic difference in molecular geometry between the rod-like trans form and bent cis form, leading to large variation in HTP upon photoisomerization.26 However, the necessity of high energy UV light to induce the trans–cis isomerization of normal azobenzene30,31 impedes their practical applications, since UV light irradiation might bring about material decomposition and damage to biosamples. In this regard, developing photoresponsive CLCs that primarily respond to visible light and near-infrared (NIR) is highly desired for the wide utilizations.12,13
Li et al. developed NIR-light-tunable CLCs loaded with azobenzene-based chiral switches and upconversion nanoparticles (UCNPs). The UCNPs successfully converted NIR light of different power density to UV light or visible light, which triggered the trans–cis and cis–trans isomerization of the chiral switches respectively and realized the reversible tuning of the reflection wavelength in visible spectrum.13 However, energy transfer from the UCNPs to the chiral switches resulted in low photoisomerization efficiency and slow response in this system. It is more straightforward to design novel chiral switches, whose photoisomerization in two directions can be induced by visible light. Recently, Li et al. designed and synthesized a special kind of azobenzene-based chiral switches possessing long conjugated structure. The reversible tuning of reflection colors from the doped CLCs was achieved by visible-light-driven photoisomerization.12
It has been proved that azobenzenes with ortho-fluorine atoms have lower energy of the n-orbital of the cis isomer, giving rise to a separation of n–π* absorption bands of the trans and cis isomers.32,33 Green and blue light can therefore be used to induce the trans–cis and cis–trans isomerization, respectively. This inspired us to design chiral switches based on fluoroazobenzene to realize visible-light tuning of helical superstructures of the CLC. One should emphasize that Katsonis et al. recently have synthesized fluorinated chiral switches, but they have not reported the visible-light-driven photoisomerization and still used UV light to trigger the trans–cis process.34 Herein, we reported the synthesis of axially chiral binaphthyl azobenzenes containing fluorine atoms ortho to azo moieties, which were proved to exhibit reversible visible-light-driven photoisomerization both in organic solvent and LC media (Fig. 1a). Furthermore, the chiral switches all exhibited high HTP values and only a small amount of the chiral switches successfully induced the formation of the helical superstructures. The CLCs formed by doping the chiral switches into nematic LC E7 generated the full-color dynamical change of the reflection driven by visible light with different wavelengths.
 |
| Fig. 1 (a) Chemical structure of the chiral switches studied in the experiment. (b) Changes of UV-vis absorption spectra of S-1-5 in chloroform (2 × 10−5 M) upon irradiation of 530 nm light (27 mW cm−2) from the initial state to the PSS530. (c) Partial UV-vis absorption spectra of S-1-5 between 420-490 nm showing the separation of n–π* bands of trans and cis isomers. (d) Changes of UV-vis absorption spectra of S-1-5 in chloroform (2 × 10−5 M) upon irradiation of 445 nm light (0.4 mW cm−2) from the PSS530 to the PSS445. | |
Results and discussion
Synthesis and characterization
The visible-light-driven chiral switches were prepared in a facile synthesis route (Fig. 1a and Experimental section Fig. 6), in which the 1,1′-binaphthyl group provides axial chirality and fluoroazobenzene moiety offers the distinct optical properties. Besides, the introduction of two mesogenic rod-like biphenyl enhances the solubility in commercially available nematic LC hosts including E7 and 5CB. The chemical structures of chiral switches were identified by 1H, 19F and 13C NMR spectroscopy.
Visible-light-driven photoisomerization
UV-vis spectra were recorded to make clear the changes of the absorption of the chiral switches before and after sequential visible light irradiation at 530 nm and 445 nm. A solution of S-1-5 in chloroform was placed in dark to maximize the absorption at 342 nm which corresponds to the π–π* absorption band of trans o-fluorinated azobenzene. As shown in Fig. 1b, 530 nm light irradiation triggered trans–cis isomerization evidenced by the intensity decrease of π–π* absorption band. In the visible part of the spectra (420–490 nm), two isobestic points at 428 nm and 464 nm were found due to the separation of n–π* absorption bands of the two isomers (Fig. 1c). The n–π* absorption band of cis isomer (450 nm) appeared with a slight blue-shift compared to that of trans isomer (468 nm), which is different from the parent azobenzenes without the substitution of electron-withdrawing fluorine atoms. The solution of S-1-5 in chloroform took about 140 s to reach photostationary state (PSS) upon 530 nm light irradiation, whereas its reverse process took about 190 s to reach PSS445 by 445 nm light irradiation (Fig. 1d). Similar phenomena were also found in the other two chiral switches (Fig. S1 and S2, ESI†). These results indicate that both the trans–cis and cis–trans isomerization of the newly designed chiral switches can be fully triggered by visible light in solvent.
Measurement of HTP
Encouraged by the reversible visible-light-driven photoisomerization of the chiral switches in organic solvent, we doped the chiral switches into the commercially available nematic LC host to evaluate their HTP variation upon visible light irradiation. As shown in Fig. 2a and S3, ESI†, a small amount of the chiral switches (0.4 wt% in E7) successfully induced the formation of CLC phase, which was testified by the characteristic fingerprint textures observed by polarized optical microscope (POM), indicating that the chiral switches all have high HTP. The screw senses of the CLCs doped with the chiral switches were all determined to be left-handed according to the miscibility tests (Fig. S4, ESI†). The HTP values of the chiral switches were measured according to Grandjean–Cano method35 (Fig. S5, ESI†). Taking the mixture of 1.0 wt% S-1-5 in E7 as an example (Fig. 2c), the distance between disclination lines gradually increased from 85 to 304 μm and finally became stable at PSS530 thanks to the trans–cis isomerization of S-1-5. The resultant percent change in β was calculated as 72.4%. Reverse process occurred when irradiated with 445 nm light. The excellent fatigue resistance was confirmed by repeated irradiation of 530 nm and 445 nm light after many cycles and no obvious degradation was observed (Fig. 2b and S8, ESI†). Therefore, the reversible tuning of S-1-5 was achieved only by visible light irradiation in LC media, which successfully induced the change of the helical superstructures. S-3-5 and S-1-5 also showed similar properties in CLC manipulation (Fig. S6–8, ESI†).
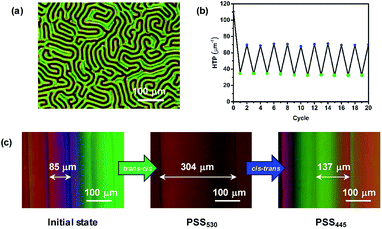 |
| Fig. 2 (a) Fingerprint textures of 0.4 wt% S-1-5 in E7 observed through POM. Scale bar, 100 μm. (b) Cycles of the changes of HTP of 1.0 wt% S-1-5 in E7 upon alternate irradiation with 530 nm and 445 nm light. (c) POM images showing the distance changes of disclination lines of 1.0 wt% S-1-5 in achiral LC E7 in the wedge cell before (left) and after sequential visible light irradiation at 530 nm (middle) and 445 nm (right). | |
The HTP values of three chiral switches are summarized in Table 1. Compared to the previously reported visible-light-driven chiral switches,12 these chiral switches all have higher HTP value at the initial state and more significant change of HTP upon light irradiation. The HTPs of S-3-5 and S-1-5 in 5CB are higher than that in E7, while S-1-3 shows higher HTP value in E7 than in 5CB. The higher HTP of S-3-5 and S-1-5 in 5CB may arise from the higher degree of similarity between the end groups of chiral switches and 5CB host. Different LC hosts result in different intermolecular association between the chiral switches and the host. Therefore, we could conclude that subtle dependence of HTP exists on the molecular structures of both the dopant and the nematic LC host, which is consistent with previously reported results.29 Moreover, HTP values of these chiral switches exhibit remarkable differences among various PSSs induced by visible light, which indicates reversible visible-light-tunable property of the chiral switches.
Table 1 Helical twisting power β (μm−1, mol%) of the chiral switches in different nematic LC hosts at different states
Sample |
Host |
β (μm−1, mol%) |
Δβa |
Δβ/βinib (%) |
Initial |
PSS530 |
PSS445 |
Decrease. Percent change in β from the initial state to the PSS530. |
S-3-5 |
E7 |
100 |
28 |
59 |
72 |
72.4 |
5CB |
133 |
34 |
80 |
99 |
74.5 |
S-1-3 |
E7 |
152 |
39 |
78 |
113 |
74.5 |
5CB |
143 |
33 |
67 |
110 |
76.9 |
S-1-5 |
E7 |
110 |
32 |
69 |
78 |
70.6 |
5CB |
146 |
29 |
68 |
117 |
80.3 |
Phototunable reflection
The high HTP value and the considerable difference among various states of the chiral switches allowed us to tune the reflection color of the CLC reversibly by visible light. Although S-1-3 has higher HTP, the poor solubility in LC host (less than 10 wt%) indicates that it is not an ideal candidate for CLC manipulation. The HTP variation of S-1-5 between PSS530 and PSS445 is larger than that of S-3-5. Therefore, S-1-5 was chosen to demonstrate efficient tuning of the reflection color in CLC upon visible light irradiation. A mixture of 15.8 wt% S-1-5 in achiral nematic LC E7 was filled into a 5 μm thick planar aligned cell via capillary force. Successfully, the CLC mixture exhibited reversible tuning of reflection color across the entire visible spectrum. At the initial state, the reflection band was located in the UV region (<400 nm). When irradiated with 530 nm light for 7 seconds, the cell appeared to be blue and the central reflection wavelength was shifted to around 440 nm. As shown in Fig. 3a and c, the reflection band red-shifted over the entire visible light region and the reflection color dynamically changed from blue via green to dark red upon continual 530 nm light irradiation within 27 s. The reverse process happened upon 445 nm light irradiation (Fig. 3b and d). Fig. 4 shows the real cell images of the CLC mixture in primary blue, green, red (RGB) reflection colors tuned by 530 nm and 445 nm light. Interestingly, a green and red “CLC” pattern in blue background was created by photomask technology. Therefore, reversible tuning of the reflection color and erasable patterning process of the CLC mixture were achieved in the visible spectrum, which is of significance in the practical display applications. These rewritable materials are expected to replace paper and lay the foundation for the realization of inkless printing technology. Besides, the reflection wavelength of the cell can be extended to NIR region (1175 nm) under irradiation of 530 nm light as expected. In the previously reported visible-light-driven CLC, high doping concentration of 22.7 wt% was needed to induce helical superstructures exhibiting dynamic reflection colors, which may narrow the temperature range of LC phase and increase viscosity of the CLC.36 Here, the new types of visible-light-driven chiral switches possess high HTP value, which can efficiently induce and tune helical superstructures with low doping concentration.
 |
| Fig. 3 Reflection color images of the CLC mixture in a 5 μm planar cell with 15.8 wt% S-1-5 in E7 (a) upon consecutive visible light irradiation at 530 nm (15 mW cm−2) and (b) 445 nm (0.4 mW cm−2). Reflective spectra of the CLC mixture doped with 15.8 wt% S-1-5 in E7 upon consecutive visible light irradiation at (c) 530 nm (15 mW cm−2) and (d) 445 nm (0.4 mW cm−2). | |
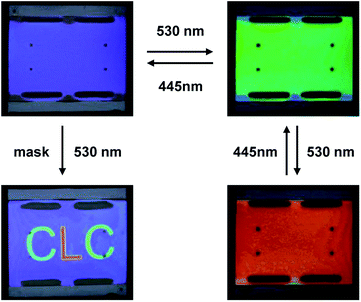 |
| Fig. 4 Real cell images of CLC mixture in a 5 μm planar cell (2.0 cm × 2.5 cm) with 15.8 wt% S-1-5 in E7 upon irradiation of visible light showing primary RGB colors and “CLC” pattern. | |
Thermal relaxation
As is well-known, the application of light-directed RGB reflection colors change in CLCs doped with azo-based chiral switches was limited because of the competing thermal back relaxation (cis–trans isomerization in darkness), which results in the lack of stability.11 It's worth noting here that the thermal relaxation process of the reflection wavelength took about 50 hours through the entire visible region (Fig. 5), illustrating that our CLCs exhibit excellent thermal stability compared to that with common azo-based chiral switches without fluorine atoms, which is 10 hours.37 The substitution of electron-withdrawing fluorine atoms may stabilize the cis isomers of the chiral switches and therefore lead to the excellent thermal stability of the CLCs.29,34 Better thermal stability of our newly designed chiral switches results in color stabilities of the reflecting displays, which is of great importance in practical applications.
 |
| Fig. 5 Thermal back process of central reflection wavelength of 15.8 wt% S-1-5 in E7 from the PSS530 at room temperature (25 °C). | |
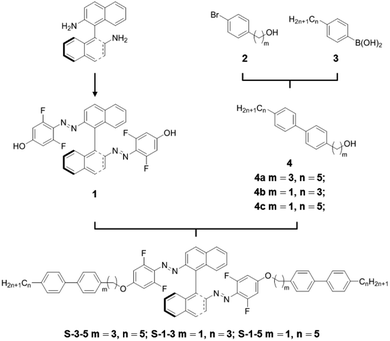 |
| Fig. 6 Synthesis of visible-light-driven chiral switches. | |
Conclusions
In conclusion, we have designed a series of visible-light-driven chiral switches based on o-fluorinated azobenzene. The substitution of four fluorine atoms endows chiral switches with visible-light-driven two-way photoisomerization, and makes cis isomers more thermally stable at the same time. A small amount of S-1-5 is able to efficiently induce self-organized helical superstructures in the CLC, whose reflection color is capable of being reversibly tuned in full-range visible spectrum. The investigation of these intriguing materials would assist full exploration of different potential applications of the CLCs, in which high energy UV light is unfavourable.
Experimental
Materials and methods
All chemicals and solvents were purchased from commercial suppliers and used without further purification. 1H NMR, 13C NMR and 19F NMR spectra were recorded in CDCl3. Chemical shifts are in δ units (ppm) with the residual solvent peak as the internal standard. The coupling constant (J) is reported in hertz (Hz). NMR splitting patterns are designed as follows: s, singlet; d, doublet; t, triplet; and m, multiplet. UV-vis spectrum was taken by a Perkin Elmer Lambda 650 Spectrometer. Textures, contact areas, reflection colors and distance changes of disclination lines were observed by a Leika DM2500p polarizing optical microscopy (POM). 530 nm light irradiation was carried out by a CCS HLV-24GR-3W LED light source. 445 nm light irradiation was carried out by a JD BL-445-300 laser light source. Reflection spectra of CLCs were examined with an Ideaoptica spectrometer.
Synthesis of chiral switches
Synthesis of intermediate 1. (S)-(−)-1,1′-Binaphthyl-2,2′-diamine (0.48 g, 1.7 mmol) was dissolved in a solution of H2O (9 mL) and concentrated HCl (1.3 mL). The solution was cooled to 0 °C with ice water bath. A solution of sodium nitrite (0.29 g, 4.2 mmol) in H2O (10 mL) was added dropwise with stirring. The solution of 3,5-difluorophenol (0.50 g, 3.87 mmol) and NaOH (0.45 g, 11.3 mmol) in H2O (15 mL) was dropped into the resulting brown yellow suspension. The suspension was acidified with aqueous HCl and filtered. The precipitate was washed with H2O and dried to get the crude product, which was purified by chromatography on silica gel with dichloromethane/ethyl acetate (19
:
1) to give a red brown solid (0.25 g, 26%). 1H NMR (500 MHz, CDCl3) δ = 8.19–8.05 (m, 4H), 7.98 (d, J = 8.2 Hz, 2H), 7.51 (t, J = 7.2 Hz, 2H), 7.34–7.21 (m, 4H), 6.17 (d, J = 12.8 Hz, 4H).
General procedure for the synthesis of intermediate 4. To a magnetically stirred mixture of 2 (1.0 mmol) and 3 (1.4 mmol) in toluene (20 mL) at ambient temperature under Ar atmosphere was added a solution of potassium carbonate (4.0 mmol) in H2O (10 mL). A small amount of methyltrioctylammonium chloride and Pd(PPh3)4 were added to the solution. The reaction mixture was then slowly heated to reflux for 12 h and cooled to ambient temperature. The solvents were removed under reduced pressure. The crude product was purified by chromatography on silica gel with dichloromethane to give the white pure product.
4a. White solid, 89% yield. 1H NMR (400 MHz, CDCl3) δ = 7.50 (dd, J = 8.2, 6.6 Hz, 4H), 7.25 (t, J = 8.2 Hz, 4H), 3.71 (t, J = 6.4 Hz, 2H), 2.75 (t, J = 7.8 Hz, 2H), 2.63 (t, J = 7.8 Hz, 2H), 1.98–1.89 (m, 2H), 1.70–1.60 (m, 2H), 1.42–1.31 (m, 2H), 0.90 (t, J = 7.0 Hz, 3H). 13C NMR (126 MHz, CDCl3) δ = 141.89, 140.57, 138.84, 138.35, 128.80, 126.99, 126.82, 62.34, 35.59, 34.23, 31.72, 31.59, 31.21, 22.59, 14.07.
4b. White solid, 90% yield. 1H NMR (500 MHz, CDCl3) δ = 7.58 (d, J = 8.2 Hz, 2H), 7.51 (d, J = 8.2 Hz, 2H), 7.42 (d, J = 8.0 Hz, 2H), 7.25 (d, J = 8.1 Hz, 2H), 4.73 (s, 2H), 2.63 (t, J = 7.8 Hz, 2H), 1.72–1.66 (m, 2H), 0.98 (t, J = 7.3 Hz, 3H). 13C NMR (500 MHz, CDCl3) δ = 141.97, 140.65, 139.55, 138.16, 127.46, 127.17, 126.91, 65.19, 37.71, 24.58, 13.91.
4c. White solid, 96% yield. 1H NMR (500 MHz, CDCl3) δ = 7.59 (d, J = 8.2 Hz, 2H), 7.51 (d, J = 8.1 Hz, 2H), 7.43 (d, J = 8.4 Hz, 2H), 7.26 (d, J = 8.0 Hz, 2H), 4.74 (s, 2H), 2.64 (t, J = 7.8 Hz, 2H), 1.69–1.62 (m, 2H), 1.39–1.33 (m, 4H), 0.90 (t, J = 6.8 Hz, 3H). 13C NMR (500 MHz, CDCl3) δ = 142.20, 140.62, 139.57, 138.12, 128.84, 127.43, 127.13, 126.90, 65.14, 35.59, 31.57, 31.16, 22.56, 14.03.
General procedure for the synthesis of S-m-n. To a magnetically stirred solution 1 (1.0 mmol) and PPh3 (3.0 mmol) in anhydrous THF (20 mL) at ambient temperature under Ar atmosphere was added dropwise a mixture of 4 (2.5 mmol) and diethyl azodicarboxylate (3.0 mmol) in THF (10 mL). The reaction mixture was then slowly warmed to reflux for 12 h and cooled to ambient temperature. The solvents were removed under reduced pressure. The crude product was purified by chromatography on silica gel with dichloromethane/petroleum ether (2
:
3) to give the orange product.
S-3-5. Orange solid, 60% yield. 1H NMR (400 MHz, CDCl3) δ = 8.11 (d, J = 9.0 Hz, 2H), 8.04–7.91 (m, 4H), 7.56–7.40 (m, 12H), 7.31–7.17 (m, 10H), 6.31 (d, J = 10.6 Hz, 4H), 3.86 (t, J = 6.3 Hz, 2H), 2.76 (t, J = 7.5 Hz, 4H), 2.67–2.58 (m, 4H), 2.10–2.01 (m, 4H), 1.69–1.60 (m, 4H), 1.39–1.31 (m, 8H), 0.91 (t, J = 6.8 Hz, 6H). 13C NMR (126 MHz, CDCl3) δ = 160.38, 158.28, 158.22, 156.20, 156.15, 149.57, 141.93, 139.63, 139.06, 138.24, 137.89, 134.63, 134.20, 128.87, 128.78, 128.03, 127.97, 127.18, 127.04, 126.80, 126.50, 113.56, 99.05, 98.84, 67.65, 35.58, 31.57, 31.50, 31.16, 30.29, 22.56, 14.03. 19F NMR (400 MHz, CDCl3) δ = −118.05. Mp: 63 °C; MS (ESI): (M + H)+ 1095.51, found 1095.51.
S-1-3. Orange solid, 55% yield. 1H NMR (500 MHz, CDCl3) δ = 8.11 (d, J = 9.0 Hz, 2H), 7.98 (dd, J = 25.8, 8.5 Hz, 4H), 7.57 (d, J = 8.3 Hz, 4H), 7.53–7.35 (m, 12H), 7.31–7.23 (m, 6H), 6.41 (d, J = 10.4 Hz, 4H), 4.98 (s, 4H), 2.62 (t, J = 7.6 Hz, 4H), 1.73–1.62 (m, 4H), 0.97 (t, J = 7.3 Hz, 6H). 19F NMR (400 MHz, CDCl3) δ = −117.87. Mp: 85 °C; MS (ESI): (M + H)+ 983.39, found 983.39.
S-1-5. Orange soild, 60% yield. 1H NMR (400 MHz, CDCl3) δ = 8.11 (d, J = 9.0 Hz, 2H), 8.04–7.90 (m, 4H), 7.56 (d, J = 8.2 Hz, 4H), 7.53–7.41 (m, 8H), 7.37 (d, J = 8.3 Hz, 4H), 7.31–7.21 (m, 6H), 6.40 (d, J = 10.4 Hz, 4H), 4.98 (s, 4H), 2.63 (t, J = 15.6 Hz, 4H), 1.65 (m, 4H), 1.38–1.32 (m, 8H), 0.90 (t, J = 14.0 Hz, 6H). 13C NMR (126 MHz, CDCl3) δ = 160.01, 158.23, 158.17, 156.15, 156.09, 149.58, 142.40, 141.40, 137.95, 137.85, 134.66, 134.17, 134.03, 128.90, 128.86, 128.05, 127.97, 127.89, 127.28, 127.23, 126.94, 126.53, 113.55, 99.49, 99.28, 70.47, 35.58, 31.55, 31.14, 22.55, 14.02. 19F NMR (400 MHz, CDCl3) δ = −117.86. Mp: 72 °C; MS (ESI): (M + H)+ 1039.45, found 1039.45.
Conflicts of interest
There are no conflicts to declare.
Acknowledgements
This work is financially supported by the National Natural Science Foundation of China (51573029, 21734003), National Key R&D Program of China (2017YFA0701302), Natural Science Foundation of Shanghai (No.17ZR1440100) and Innovation Program of Shanghai Municipal Education Commission (Grant No. 2017-01-07-00-07-E00027).
Notes and references
- H. K. Bisoyi and Q. Li, Angew. Chem., Int. Ed., 2016, 55, 2994 CrossRef CAS PubMed.
- D. J. D. Davies, A. R. Vaccaro, S. M. Morris, N. Herzer, A. P. H. J. Schenning and C. W. M Bastiaansen, Adv. Funct. Mater., 2013, 23, 2723 CrossRef CAS.
- K. Masuki, A. Takuya, S. Natsuki and W. Tatsuo, Chem. Mater., 2009, 21, 564 CrossRef.
- J. Xiang, Y. N. Li, Q. Li, D. A. Paterson, J. M. D. Storey, C. T. Imrie and O. D. Lavrentovich, Adv. Mater., 2015, 27, 3014 CrossRef CAS PubMed.
- C. A. Bailey, V. P. Tondiglia, L. V. Natarajan, M. M. Duning, R. L. Bricker, R. L. Sutherland, T. J. White, M. F. Durstock and T. J. Bunning, J. Appl. Phys., 2010, 107, 013105 CrossRef.
- A. Saha, Y. Tanaka, Y. Han, C. M. W. Bastiaansen, D. J. Broer and R. P. Sijbesma, Chem. Commun., 2012, 48, 4579 RSC.
- Y. Han, K. Pacheco, C. W. M. Bastiaansen, D. J. Broer and R. P. Sijbesma, J. Am. Chem. Soc., 2010, 132, 2961 CrossRef CAS PubMed.
- T. J. White, M. E. McConney and T. J. Bunning, J. Mater. Chem., 2010, 20, 9832 RSC.
- Q. Li, Y. Li, J. Ma, D.-K. Yang, T. J. White and T. J. Bunning, Adv. Mater., 2011, 23, 5069 CrossRef CAS PubMed.
- M. Mathews and N. Tamaoki, J. Am. Chem. Soc., 2008, 130, 11409 CrossRef CAS PubMed.
- Y. Li, A. Urbas and Q. Li, J. Am. Chem. Soc., 2012, 134, 9573 CrossRef CAS PubMed.
- Y. Wang, A. Urbas and Q. Li, J. Am. Chem. Soc., 2012, 134, 3342 CrossRef CAS PubMed.
- L. Wang, H. Dong, Y. Li, C. Xue, L.-D. Sun, C.-H. Yan and Q. Li, J. Am. Chem. Soc., 2014, 136, 4480 CrossRef CAS PubMed.
- Y. Kim and N. Tamaoki, J. Mater. Chem. C, 2014, 2, 9258 RSC.
- S. Kurihara, S. Nomiyama and T. Nonaka, Chem. Mater., 2001, 13, 1992 CrossRef CAS.
- Q. Li, L. F. Li, J. Kim, H.-S. Park and J. Williams, Chem. Mater., 2005, 17, 6018 CrossRef CAS.
- J. Lub, P. van de Witte, C. Doornkamp, J. P. A. Vogels and R. T. Wegh, Adv. Mater., 2003, 15, 1420 CrossRef CAS.
- U. A. Hrozhyk, S. V. Serak, N. V. Tabiryan, T. J. White and T. J. Bunning, Opt. Express, 2010, 18, 9651 CrossRef CAS PubMed.
- L. De Sio, T. Placido, S. Serak, R. Comparelli, M. Tamborra, N. Tabiryan, M. L. Curri, R. Bartolino, C. Umeton and T. Bunning, Adv. Opt. Mater., 2013, 1, 899 CrossRef.
- T. Yoshioka, T. Ogata, T. Nonaka, M. Moritsugu, S.-N. Kim and S. Kurihara, Adv. Mater., 2005, 17, 1226 CrossRef CAS.
- E. Montbach, N. Venkataraman, A. Khan, I. Shiyanovskaya, T. Schneider, J. W. Doane, L. Green and Q. Li, SID Int. Symp. Dig. Tech.
Pap., 2008, 39, 919 CrossRef CAS.
- S. M. Morris, P. J. W. Hands, S. Findeisen-Tandel, R. H. Cole, T. D. Wilkinson and H. J. Coles, Opt. Express, 2008, 16, 18827 CrossRef CAS PubMed.
- S. Furumi and N. Tamaoki, Adv. Mater., 2010, 22, 886 CrossRef CAS PubMed.
- M. Moirangthem, R. Arts, M. Merkx and A. P. H. J. Schenning, Adv. Funct. Mater., 2016, 26, 1154 CrossRef CAS.
- D. J. Mulder, A. P. H. J. Schenning and C. W. M. Bastiaansen, J. Mater. Chem. C, 2014, 2, 6695 RSC.
- H. K. Bisoyi and Q. Li, Chem. Rev., 2016, 116, 15089 CrossRef CAS PubMed.
- J. Lu, W. Gu, J. Wei, W. Zhang, Z. Zhang, Y. Yu, N. Zhou and X. Zhu, J. Mater. Chem. C, 2016, 4, 9576 RSC.
- Y. Wang and Q. Li, Adv. Mater., 2012, 24, 1926 CrossRef CAS PubMed.
- H. K. Bisoyi and Q. Li, Acc. Chem. Res., 2014, 47, 3184 CrossRef CAS PubMed.
- T. J. White, R. L. Bricker, L. V. Natarajan, N. V. Tabiryan, L. Green, Q. Li and T. J. Bunning, Adv. Funct. Mater., 2009, 19, 3484 CrossRef CAS.
- Y. Xie, D. Fu, O. Jin, H. Zhang, J. Wei and J. Guo, J. Mater. Chem. C, 2013, 1, 7346 RSC.
- D. Bléger, J. Schwarz, A. M. Brouwer and S. Hecht, J. Am. Chem. Soc., 2012, 134, 20597 CrossRef PubMed.
- L. Qin, W. Gu, J. Wei and Y. Yu, Adv. Mater., 2018, 30, 1704941 CrossRef PubMed.
- H. Huang, T. Orlova, B. Matt and N. Katsonis, Macromol. Rapid Commun., 2017, 39, 1700387 CrossRef PubMed.
- G. Heppke and F. Oestreicher, Z. Naturforsch. A, 1977, 32, 899 Search PubMed.
- K. Akagi, Chem. Rev., 2009, 109, 5354 CrossRef CAS PubMed.
- J. Ma, Y. N. Li, T. White, A. Urbas and Q. Li, Chem. Commun., 2010, 46, 3463 RSC.
Footnote |
† Electronic supplementary information (ESI) available. See DOI: 10.1039/c8ra07657j |
|
This journal is © The Royal Society of Chemistry 2018 |
Click here to see how this site uses Cookies. View our privacy policy here.