DOI:
10.1039/C7SC05109C
(Edge Article)
Chem. Sci., 2018,
9, 3227-3232
Reversible stapling of unprotected peptides via chemoselective methionine bis-alkylation/dealkylation†
Received
30th November 2017
, Accepted 20th February 2018
First published on 26th February 2018
Abstract
We have developed a general peptide macrocyclization strategy that involves a facile and chemoselective methionine bis-alkylation/dealkylation process. This method provides a straightforward and easy approach to generate cyclic peptides with tolerances of all amino acids (including Cys), variable loop sizes, and different linkers. The Met bis-alkylation we apply in this strategy yields two additional on-tether positive charges that could assist in the cellular uptake of the peptides. Notably, the bis-alkylated peptide could be reduced to release the original peptide both in vitro and within cellular environments. This strategy provides an intriguing and facile traceless post-peptide-synthesis modification with enhanced cellular uptakes. Peptides constructed with this method could be utilized to zero in on various protein targets or to achieve other goals, such as drug delivery.
Introduction
Because of their pivotal role in modulating life processes, protein–protein interactions (PPI) provide a rich space for drug development.1 Although peptides have been, and continue to be, broadly utilized as efficient PPI modulators, their intrinsic instability in vivo coupled with poor cellular uptake largely reduce their therapeutic potential.2 Constrained peptides, which are chemically “locked” into bioactive conformations and have improved proteolytic stability and membrane permeability, represent promising candidates for the next-generation of peptide therapeutics.3 Therefore, the endeavor to chemically stabilize peptides in order to attain desirable biophysical properties is an effort that has piqued the interests of both industry and academia.4 These efforts, in general, have involved a precisely designed tether that is chemically added to the peptides at different positions, either as a side cross tether,5 an N-capped nucleated moiety, or a hydrogen bond surrogate.6 Stabilized peptides have proven useful in targeting various intracellular PPIs, including p53/Mdm2, estrogen receptor α (ER-α), BCL-2 family proteins, hypoxia-inducible factor (HIF), Notch complexes, and so on.7 For the most part, the additional chemical modifications were intentionally designed at the solvent exposed face in order to avoid unwanted interactions with target proteins. However, as both proteins and peptides are flexible molecules, structural evidence indicates that the tether can contribute to the protein-peptide interactions.8 Contrastingly, in many cases peptides stabilized with additional tethers were found to have detrimental effects on binding with their targets, as reported by Baumach et al. on somatostatin receptor subtype 2 (SST2)9 and Mizejewski et al. on human alpha fetoprotein (HAFP).10 This effect is understandable but still problematic, and highlights the need for newer peptides that maintain the advantages of conventional constrained peptides, but avoid unwanted tether/protein interactions.
Thus, we set out to develop a post-peptide-synthesis modification, which would do just that. The enhanced serum stability and cellular uptake hallmarks of conventional peptides were preserved, while the original peptide was released upon intracellular stimulus, deflecting the potential for any unwanted interactions. The intracellular reductive cleavage of disulfide bonds was well utilized for various applications.11 However, disulfide bonds typically do not show a significant enhancement in uptake. Very recently, Grison et al. introduced a GSH reducible dibromomaleimide linker into two model peptides (BID and RNase S) that led to enhancements of helicity and proteolytic resistance.12 Notably, the linker could be further modified with biotin, fluorescein, and PEG azides. Deming et al. systematically studied the reversible alkylation of Met in linear peptides,13 and we reported a reversible alkylation of thioether-tethered cyclic peptides with preferable biophysical properties.14 Herein, we report a straightforward and traceless modification of peptides by bis-alkylation/dealkylation of Met residues that resulted in satisfying yields and excellent functional group tolerance.15 Peptides modified with this method showed enhanced cellular uptake, negligible cytotoxicity, and were reducible upon GSH treatment, as summarized in Fig. 1.
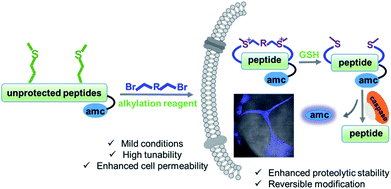 |
| Fig. 1 Schematic presentation of the Met bis-alkylation/dealkylation strategy for peptide macrocyclization. GSH = glutathione. | |
This convenient and triggered release strategy significantly broadens the chemical space of stabilized peptides and could have many important uses among various research areas, including therapeutic development, probing peptides, biomaterial, and drug delivery.
Results and discussion
First, we utilized a model peptide with an RGD moiety, shown in Fig. 2A, to screen for suitable Met bisalkylation conditions (Table S1†). RGD was intentionally used as model due to its broad use as a cancer cell-targeting peptide as well as its bulky Arg/Asp residues, which could be used to examine the reaction's chemoselectivity and functional group tolerance.16 Based on our trials, this reaction could not be applied in solid phase synthesis. This may be due to the low nucleophilicity of the residue and its pseudo-dilution effect in solid phase, or the degradation of cyclized peptides in the resin cleavage cocktail (TFA/TIS/EDT/H2O).17 The modification was smoothly fulfilled in the solution phase with high conversion. Using these optimized conditions, we tested different di-alkylating linkers (Fig. 2A). The simple alkyl linkers, f and g, with significant lower electrophilicity did not react under the standard conditions. Based on HPLC-MS analysis, the other six dibromide linkers reacted smoothly with peptide 1 to generate corresponding bis-alkylation products with satisfying conversions. Linker e yielded a small amount of mono alkylated product (Fig. S3†). The product 1d was confirmed by ESI-MS with the addition of 54 Da and 1H-NMR analysis showing a clear shift of Met methyl and olefinic protons (Fig. 2B and S1†). Peptides with different residues and loop sizes were tested for the reaction scope. Linkers a and c, which are commonly used in the peptide stapling,5 were used with two Met positioned at i, i+3, i+4, and i+7 respectively, and reacted smoothly with satisfying conversions, as shown in Fig. 2C. The different cyclization efficiency of peptide 5, 6, and 7 showed that both spacer and sequences influenced the cyclizing efficiency (Fig. 2C and S3†). Notably, peptide 5 reacted smoothly, providing peptide 5a/5c with high conversion and an intact Cys after the reaction. The free thiol group on peptide 5a was confirmed by the 5,5′-dithiobis-(2-nitrobenzoic acid) (DTNB) assay18 and confirmed by HPLC-MS as shown in Fig. S2.† Furthermore, this method could be easily extended to construct a bicyclic peptide. Peptide 10, with three Mets (Fig. 2D), reacted smoothly with 1,3,5-tris(bromomethyl) benzene to generate bicyclic peptide 10j. Based on our experimental observation, this method could provide an efficient and general approach towards generating cyclic peptides with different linkers and different loop sizes.
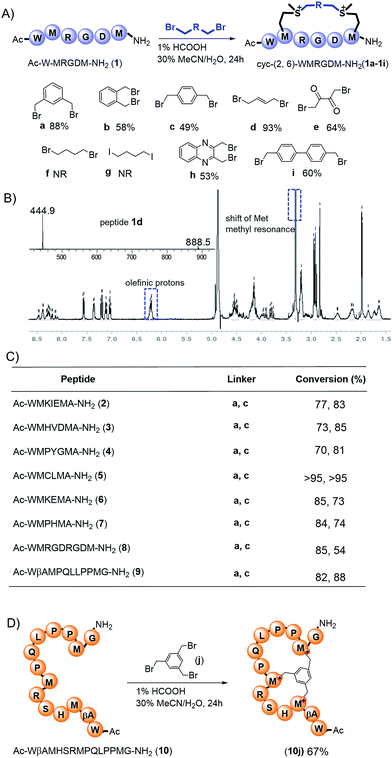 |
| Fig. 2 Preparation of the cyclic peptides via methionine alkylation. (A) Met bis-alkylation of peptide 1 with different linkers. Peptides were dissolved in a solution of 1% HCOOH MeCN/H2O (30 : 70, v/v) and stirred for 24 h at room temperature. The optimization process was summarized in Table S1.† NR: no reaction. (B) ESI-MS and 1H-NMR spectra of peptide 1d in CD3OD. (C) Different peptide sequences and loop sizes cyclized by Met bis-alkylation. (D) Peptide 10 with three Mets reacted with 1,3,5-tris(bromomethyl) benzene to generate bicyclic peptide 10j. Conversions: [desired product/(desired product + starting material)] were determined by integration of reverse-phase HPLC. | |
Unlike most of the present cyclic peptide construction strategies, our method does not require preparation of precious unnatural amino acids and laborious experimental operation. In general, peptides could be purchased from commercial sources and directly used. Additionally, peptides cleaved from the resin could be used directly for cyclization without the need for time-consuming HPLC purification.
Deming et al., along with our previous research, demonstrated that sulfonium is reducible to regenerate the original peptides under reductive conditions.13,14 We tested cyclized peptides 1c, 1d, and 1i under reductive conditions (Fig. 3A) and found that upon treatment with 2-mercaptopyridine (10 equiv., 10 mM) in PBS buffer (pH = 7.4), all peptides showed time-dependent regeneration of the parental peptide 1 (Fig. 3A and B). Peptide 1i showed the quickest dealkylation, in opposition to alkylation kinetics. As shown in Fig. 3C, the bis-alkylation cyclization significantly enhanced the trypsin stability of peptides 1c and 1i, compared to their parental linear peptide 1.
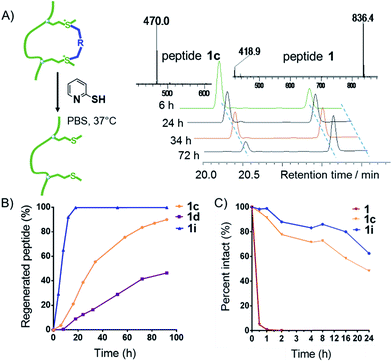 |
| Fig. 3 Bis-alkylated peptides are reducible and more resistant to proteolysis. (A) Dealkylation of model peptides 1c, 1d, and 1i (1 mmol) in the presence of PyS (10 mmol) in PBS (pH = 7.4) at 37 °C. HPLC traces of dealkylation process of peptide 1c at different time intervals were presented. (B) Plot of regenerated peptides versus time. (C) In vitro trypsin (1%) digestion assay. Peptide 1, 1c, and 1i (1 mmol) incubated at 37 °C for different time intervals. The amounts of the peptides were examined by integration of reverse-phase HPLC. | |
A major limitation of peptides' therapeutic application is poor cellular uptake, and peptide stabilization could provide an intriguing solution.1,19 With two additional on-tether positive charges from the sulfonium, we envisioned this modification strategy might lead to a facile and reversible approach for the generation of cell permeable peptides. In order to examine the cyclic peptide's permeability, peptide 11 FITC-βAMRRRM-NH2 was cyclized using linker a–e, h, and i, and tested in HeLa and U2OS cells using a FACS assay (Fig. 4A). As controls, we prepared peptides 12 (FITC-βA-CRRRC-NH2) and 13 (FITC-βA-hCRRRhC-NH2, hC = homo-Cys) that were cyclized using linker c and the cell penetrating peptide TAT (FITC-βA-RKKRRQRRR). According to the results of confocal microscopy imaging, the sulfonium cyclic peptides displayed a diffuse cellular distribution in HeLa cells, and the majority of the peptides were distributed in the cytoplasm and a small fraction was also detected in the nucleus (Fig. 4B). As shown in Fig. 4C and S4,† all cyclized peptides exhibited better cellular uptakes than peptide 11, with peptide 11c showing the best uptake. In the presence of 10% fetal bovine serum, the cellular uptake of peptide 11a, 11c and 11i in HeLa cells show the same tendency as in the absence of serum at different concentrations (Fig. S5†). MTT assay show that there are no obvious cytotoxicity of these cationic peptides at 60 μM in HeLa cells even incubated for 12 h (Fig. S6†). To explore the on-tether positive charge effects, peptide 12c and 13c were prepared,3 and we observed that peptide 11c showed a better cellular uptake than the thioether cyclic peptide. The superior cellular uptakes of peptide 11c may be attributed to the on-tether positive charges. As peptide macrocyclization is not the ubiquitous effect for enabling intracellular access,20 the cellular uptakes of peptide FAM-βA-RMILMRLLQ-CONH2 (14) and FAM-βA-MCNVVPLY(po3)DLLLEM-CONH2 (15) containing different sequences cyclized by linker c were tested in T47D cells (Fig. 3D). The results showed that peptide 14c showed very limited improvement of cellular uptake than its linear precursor, while peptide 15c with a head-to-tail cyclization manner showed significantly improved cellular uptake than its linear precursor.
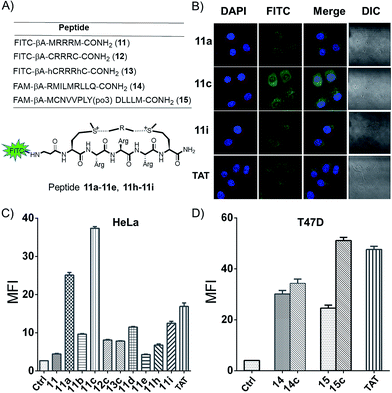 |
| Fig. 4 Met bis-alkylation increases the peptides' cellular uptakes. (A) A schematic presentation of peptides. (B) Confocal microscopy images of HeLa cells after treatment for 4 h with 10 μM peptides 11a, 11c, 11i, and TAT. (C) Flow cytometry comparison of the cellular uptake efficiency of the FITC labelled peptides 11a–e, 11h, and 11i, thioether cyclic peptides 12c and 13c, and the cell penetrating peptide TAT (10 μM, 4 h) in Hela cells. (D) Flow cytometry comparison of the cellular uptake efficiency of the FAM labelled peptides 14, 14c, and 15, 15c (10 μM, 4 h) in T47D cells in the medium containing 10% fetal bovine serum. | |
We prepared peptides (16–19) for the AMC release assay to test whether or not the tether is reducible under cellular circumstances (Fig. 5A and B). Peptide 16 was a reported substrate of caspase-3, while peptide 17 and 18 were linear control peptides based on mutations of peptide 16. Peptide 17i was a Met bis-alkylated peptide 17 with linker i, while Peptide 18i was a Cys bis-alkylated peptide 18 with linker i as a negative control. Peptide 19 was peptide 16 fused with an N-terminus R9 for better cellular uptake (Fig. 5B). First, the peptides were allowed to react with caspase-3, and then monitored by an enVision multilable plate reader (Ex = 340 nm, Em = 450 nm) based on AMC cleavage.21 Results from the in vitro caspase-3 assays clearly showed that the constrained peptide 17i was not a suitable substrate for caspase-3 and that peptide 17's quick degradation suggested the addition and mutation of Met would not interfere with the enzyme's activity. Peptide 18 and 18i showed no caspase-3 activity in vitro. Peptide 19 showed decreased susceptibility to caspase-3 compared with peptide 16 (Fig. 5C). Subsequently, U2OS cells were treated by the apoptosis inducer ABT-737 (10 μM) for 1 hour to induce caspase activity, and then were lysed and collected and allowed to react with different peptides (Fig. 5D). Compared with the in vitro kinetic test, the AMC release efficiency of peptide 17i increased gradually during 24 h, indicating that peptide 17i was defalcated to the linear peptide and recognized by caspses-3. As expected, when using the U2OS cell lysate pretreated with the pan caspase inhibitor Z-VAD–(OMe)–CH2F (FMK), peptide 17i's caspase-3 activity was reduced significantly (Fig. 5D). However, peptide 18i, which was cyclized by an irreversible method, showed no susceptibility to caspase-3. The reducible properties of peptide 17i was also observed in cell by the AMC release assay (Fig. S9†). After incubation for 12 hours, 17i show higher caspase-3 activity than the linear peptide 16, while lower than 19, suggesting that the reduction of peptide 17i is a slow process in cell. The confocal microscopy assays were carried out to further confirm the reversible nature of the bis-alkylation of Met. U2OS cells were treated with peptides for 4 hours to allow adequate cellular uptake, then washed to remove any of the peptides in solution, and finally incubated in fresh medium with additional 10 mM GSH to accelerate the reduction procedure. At different time intervals, the cells were treated with ABT-737 (10 μM, 1 h) to induce caspase activity, and then fixed (Fig. 5A). As shown in Fig. 5E, under the 405 nm excitation, peptide 17i was lighted gradually. In the presence of 10% fetal bovine serum, peptide 17i could also be lighted at 50 μM while with a weaker fluorescence intensity which likely due to the slow reduction process in the cellular environment at a relative lower concentration (Fig. S7A†). Peptide 19 was lighted at an earlier time and also exhibited a membrane lysis effect (Fig. S7B and S8†). Lactate dehydrogenase (LDH) release assay of the sulfonium peptide 17i and 18i showed negligible membrane toxicity at 160 μM (Fig. S8†).
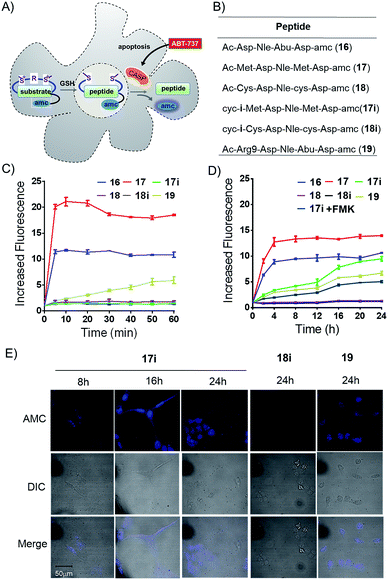 |
| Fig. 5 (A) Schematic illustration of the AMC release assay. (B) Peptides used for the AMC release assay. (C) AMC release efficiency of peptides 16–19 (5 μm) treated with caspase-3 protein for 1 h and monitored at 5 minute intervals. (D) Time-dependent release of AMC of peptides 16–19 (5 μm) treated with U2OS cell lysates in the absence and presence of caspase inhibitor FMK (100 μm) for 24 h. (E) Confocal microscopy images of U2OS cells after treatment with peptides 17i, 18i and 19 at 100 μM for 4 h, followed by incubation with GSH at 10 mM for 8, 16, or 24 h and then treatment with the apoptotic inducer ABT-737 (10 μM) for 1 h. | |
No fluorescent signals were observed in cells treated by peptide 18i following 24 hours. Taken together, we concluded that, under the adopted conditions, the sulfonium bond cyclized peptide 17i was dealkylated and recognized by the caspase.
Conclusions
In conclusion, we have herein reported a chemoselective bis-alkylation/dealkylation of Met that provides a facile and reversible peptide stapling strategy. This method yields satisfying functional group tolerance and conversion yet only requires simple operation on commercially available peptides. Because two additional positive charges are added onto the tether via sulfonium bond formation, the resulting cyclic peptides show better cellular uptakes and enzymatic stability than their linear parental peptides. This process is straightforward, mild, functional-group-tolerant, and high-yielding, and one could easily design suitable modifications on the tether or on the peptides.22 Numerous potential applications could be achieved based on this traceless modification strategy,23 and investigations for utilizing this strategy to construct PPI ligands or develop drug delivery vectors are currently underway in our laboratory and will be reported in due course. As a proof of concept study, this method provides an intriguing alternative to those that are presently utilized for the stabilization of peptides. We are at this time investigating new modifications of both Met and linkers in our laboratory. We believe further development of this strategy could lead to standardization of a facile peptide modification protocol that could be easily utilized by scientists in both the biological or material research fields.
Conflicts of interest
There are no conflicts of interest to declare.
Acknowledgements
We acknowledge financial support from the Natural Science Foundation of China grants 21778009 and 81701818; MOST 2015DFA31590; the Shenzhen Science and Technology Innovation Committee, JCYJ20170412150719814 and GJHS20170310093122365. Human cervical cancer cell line, HeLa (CCL-2, American Type Culture Collection), Human breast cancer cell line, T47D (HTB-113, American Type Culture Collection) and Human osteosarcoma cancer cell line, U2OS (HTB-96, American Type Culture Collection) were obtained from American Type Culture Collection (ATCC).
Notes and references
- L. G. Milroy, T. N. Grossmann, S. Hennig, L. Brunsveld and C. Ottmann, Chem. Rev., 2014, 114, 4695–4748 CrossRef CAS PubMed.
- K. Fosgerau and T. Hoffmann, Drug Discovery Today, 2015, 20, 122–128 CrossRef CAS PubMed; D. J. Craik, D. P. Fairlie, S. Liras and D. Price, Chem. Biol. Drug Des., 2013, 55, 136–147 Search PubMed; A. Roxin and G. Zheng, Future Med. Chem., 2012, 4, 1601–1618 CrossRef PubMed.
- A. Zorzi, K. Deyle and C. Heinis, Curr. Opin. Chem. Biol., 2017, 38, 24–29 CrossRef CAS PubMed; A. F. C. Teresa and C. Alessio, ChemMedChem, 2016, 11, 787–794 CrossRef PubMed.
- A. A. Ivanov, F. R. Khuri and H. Fu, Trends Pharmacol. Sci., 2013, 34, 393–400 CrossRef CAS PubMed; A. A. Kaspar and J. M. Reichert, Drug Discovery Today, 2013, 18, 807–817 CrossRef PubMed.
- D. Y. Jackson, D. S. King, J. Chmielewski, S. Singh and P. G. Schultz, J. Am. Chem. Soc., 1991, 113, 9391–9392 CrossRef CAS; C. E. Schafmeister, J. Po and G. L. Verdine, J. Am. Chem. Soc., 2000, 122, 5891–5892 CrossRef; S. Bellotto, S. Chen, I. R. Rebollo, H. A. Wegner and C. Heinis, J. Am. Chem. Soc., 2014, 136, 5880–5883 CrossRef PubMed; F. Zhang, O. Sadovski, S. J. Xin and G. A. Woolley, J. Am. Chem. Soc., 2007, 129, 14154–14155 CrossRef PubMed; N. Assem, D. J. Ferreira, D. W. Wolan and P. E. Dawson, Angew. Chem., Int. Ed., 2015, 54, 8665–8668 CrossRef PubMed; A. M. Spokoyny, Y. Zou, J. J. Ling, H. Yu, Y. S. Lin and B. L. Pentelute, J. Am. Chem. Soc., 2013, 135, 5946–5949 CrossRef PubMed; H. Jo, N. Meinhardt, Y. Wu, S. Kulkarni, X. Hu, K. E. Low, P. L. Davies, W. F. DeGrado and D. C. Greenbaum, J. Am. Chem. Soc., 2012, 134, 17704–17713 CrossRef PubMed; Y. H. Lau, P. de Andrade, S.-T. Quah, M. Rossmann, L. Laraia, N. Sköld, T. J. Sum, P. J. E. Rowling, T. L. Joseph, C. Verma, M. Hyvönen, L. S. Itzhaki, A. R. Venkitaraman, C. J. Brown, D. P. Lane and D. R. Spring, Chem. Sci., 2014, 5, 1804–1809 RSC; Y. Zou, A. M. Spokoyny, C. Zhang, M. D. Simon, H. Yu, Y. S. Lin and B. L. Pentelute, Org. Biomol. Chem., 2014, 12, 566–573 Search PubMed.
- H. Zhao, Q.-S. Liu, H. Geng, Y. Tian, M. Cheng, Y.-H. Jiang, M.-S. Xie, X.-G. Niu, F. Jiang, Y.-O. Zhang, Y.-Z. Lao, Y.-D. Wu, N.-H. Xu and Z.-G. Li, Angew. Chem., Int. Ed., 2016, 55, 12088–12093 CrossRef CAS PubMed; Y. Tian, D. Wang, J. Li, C. Shi, H. Zhao, X. Niu and Z. Li, Chem. Commun., 2016, 52, 9275–9278 RSC; A. Patgiri, M. Z. Menzenski, A. B. Mahon and P. S. Arora, Nat. Protoc., 2010, 5, 1857–1865 CrossRef PubMed.
- P. M. Cromm, J. Spiegel and T. N. Grossmann, ACS Chem. Biol., 2015, 10, 1362–1375 CrossRef CAS PubMed; C. Phillips, L. R. Roberts, M. Schade, R. Bazin, A. Bent, N. L. Davies, R. Moore, A. D. Pannifer, A. R. Pickford, S. H. Prior, C. M. Read, A. Scott, D. G. Brown, B. Xu and S. L. Irving, J. Am. Chem. Soc., 2011, 133, 9696–9699 CrossRef PubMed; D. Wang, W. Liao and P. S. Arora, Angew. Chem., Int. Ed., 2005, 44, 6525–6529 CrossRef PubMed; L. K. Henchey, S. Kushal, R. Dubey, R. N. Chapman, B. Z. Olenyuk and P. S. Arora, J. Am. Chem. Soc., 2010, 132, 941–943 CrossRef PubMed.
- M. M. Wiedmann, Y. S. Tan, Y. Wu, S. Aibara, W. Xu, H. F. Sore, C. S. Verma, L. Itzhaki, M. Stewart, J. D. Brenton and D. R. Spring, Angew. Chem., Int. Ed., 2017, 56, 524–529 CrossRef CAS PubMed; A. Glas, D. Bier, G. Hahne, C. Rademacher, C. Ottmann and T. N. Grossmann, Angew. Chem., Int. Ed., 2014, 53, 2489–2493 CrossRef PubMed; M. L. Stewart, E. Fire, A. E. Keating and L. D. Walensky, Nat. Chem. Biol., 2010, 6, 595–601 CrossRef PubMed; T. E. Speltz, S. W. Fanning, C. G. Mayne, G. L. Greene and T. W. Moore, Angew. Chem., Int. Ed., 2016, 55, 4252–4255 CrossRef PubMed; M. Xie, H. Zhao, Q. Liu, Y. Zhu, F. Yin, Y. Liang, Y. Jiang, D. Wang, K. Hu, X. Qin, Z. Wang, Y. Wu, N. Xu, X. Ye, T. Wang and Z. Li, J. Med. Chem., 2017, 60, 8731–8740 CrossRef PubMed.
- W. R. Baumach, T. A. Carrick, M. H. Pausch, B. Bingham, D. Carmingnac, I. C. A. F. Robinson, R. Houghten, C. M. Eppler, L. A. Price and J. R. Zysk, Mol. Pharmacol., 1998, 54, 864–873 CrossRef.
- G. J. Mizejewski, M. Muehlemann and M. Dauphinee, Chemotherapy, 2006, 52, 83–90 CrossRef CAS PubMed.
- Z. Qian, X. Xu, J. F. Amacher, D. R. Madden, E. C. Boyaka and D. Pei, Angew. Chem., Int. Ed., 2015, 54, 5874–5878 CrossRef CAS PubMed; Z. Qian, C. A. Rhodes, L. C. McCroskey, J. Wen, G. A. Kubi, D. J. Wang, D. C. Guttridge and D. Pei, Angew. Chem., Int. Ed., 2017, 56, 1525–1529 CrossRef PubMed.
- A. M. Grison, G. M. Burslem, J. A. Miles, L. K. A. Pilsl, D. J. Yeo, Z. Imani, S. L. Warriner, M. E. Webb and A. J. Wilson, Chem. Sci., 2017, 8, 5166–5171 RSC.
- J. R. Kramer and T. J. Deming, Chem. Commun., 2013, 49, 5144–5146 RSC; J. R. Kramer and T. J. Deming, Biomacromolecules, 2012, 13, 1719–17123 CrossRef CAS PubMed.
- K. Hu, C. Sun and Z. Li, Bioconjugate Chem., 2017, 28, 2001–2007 CrossRef CAS PubMed; X. Shi, Y. Jiang, D. Yang, H. Zhao, Y. Tian and Z. Li, Chin. Chem. Lett., 2017 DOI:10.1016/j.cclet.2017.07.003; H. Lin, Y. Jiang, Q. Zhang, K. Hu and Z. Li, Chem. Commun., 2016, 52, 10389–10391 RSC; H. Lin, Y. Jiang, K. Hu, Q. Zhang, C. He, T. Wang and Z. Li, Org. Biomol. Chem., 2016, 14, 9993–9999 Search PubMed; Q. Zhang, F. Jiang, B. Zhao, H. Lin, Y. Tian, M. Xie, G. Bai, A. M. Gilbert, G. H. Goetz, S. Liras, A. A. Mathiowetz, D. A. Price, K. Song, M. Tu, Y. Wu, T. Wang, M. E. Flanagan, Y. D. Wu and Z. Li, Sci. Rep., 2016, 6, 38573 CrossRef PubMed; K. Hu, H. Geng, Q. Zhang, Q. Liu, M. Xie, C. Sun, W. Li, H. Lin, F. Jiang, T. Wang, Y. D. Wu and Z. Li, Angew. Chem., Int. Ed., 2016, 55, 8013–8017 CrossRef PubMed; Y. Tian, J. Li, H. Zhao, X. Zeng, D. Wang, Q. Liu, X. Niu, X. Huang, N. Xu and Z. Li, Chem. Sci., 2016, 7, 3325–3330 RSC; B. Zhao, Q. Zhang and Z. Li, J. Pept. Sci., 2016, 22, 540–544 CrossRef PubMed.
- T. J. Deming, Chem. Rev., 2016, 116, 786–808 CrossRef CAS PubMed; T. J. Deming, Bioconjugate Chem., 2017, 28, 691–700 CrossRef PubMed.
- Y. Wang and D. H.-C. Chou, Angew. Chem., Int. Ed., 2015, 54, 1–5 CrossRef CAS; Y. Wang, B. J. Bruno, S. Cornillie, J. M. Nogieira, D. Chen, T. E. Cheatham, C. S. Lim and D. H. Chou, Chemistry, 2017, 23, 7087–7092 CrossRef PubMed.
- M. Taichi, T. Kimura and Y. Nishiuchi, Int. J. Pept. Res. Ther., 2009, 15, 247–253 CrossRef CAS.
- M. Ozyurek, S. Baki, N. Gungor, S. E. Celik, K. Guclu and R. Apak, Anal. Chim. Acta, 2012, 750, 173–181 CrossRef CAS PubMed.
- Q. Chu, R. E. Moellering, G. J. Hilinski, Y.-W. Kim, T. N. Grossmann, J. T. H. Yeh and G. L. Verdine, MedChemComm, 2015, 6, 111–119 RSC; Y. Tian, X. Zeng, J. Li, Y. Jiang, H. Zhao, D. Wang, X. Huang and Z. Li, Chem. Sci., 2017, 8, 7576–7581 RSC; R. Wallbrecher, L. Depre, W. P. R. Verdurmen, P. H. B. Geurts, R. H. van Duinkerken, M. J. Zekveld, P. Timmerman and R. Brock, Bioconjugate Chem., 2014, 25, 955–964 CrossRef CAS PubMed; Y. A. Nagel, P. S. Raschle and H. Wennemers, Angew. Chem., Int. Ed., 2017, 56, 122–126 CrossRef PubMed.
- F. Bernal, A. F. Tyler, S. J. Korsmeyer, L. D. Walensky and G. L. Verdine, J. Am. Chem. Soc., 2007, 129, 2456–2457 CrossRef CAS PubMed; R. E. Moellering, M. Cornejo, T. N. Davis, C. D. Bianco, J. C. Aster, S. C. Blacklow, A. L. Kung, D. G. Gilliland, G. L. Verdine and J. E. Bradner, Nature, 2009, 462, 182–188 CrossRef PubMed; T. N. Grossmanna, J. T.-H. Yeha, B. R. Bowman, Q. Chu, R. E. Moellering and G. L. Verdine, Proc. Natl. Acad. Sci. U. S. A., 2012, 109, 17942–17947 CrossRef PubMed; J. S. Appelbaum, J. R. LaRochelle, B. A. Smith, D. M. Balkin, J. M. Holub and A. Schepartz, Chem. Biol., 2012, 19, 819–830 CrossRef PubMed; J. K. L. Sinclair and A. Schepartz, Org. Lett., 2014, 16, 4916–4919 CrossRef PubMed; J. R. LaRochelle, G. B. Cobb, A. Steinauer, E. Rhoades and A. Schepartz, J. Am. Chem. Soc., 2015, 137, 2536–2541 CrossRef PubMed; M. Li, Y. Tao, Y. Shu, J. R. LaRochelle, A. Steinauer, D. Thompson, A. Schepartz, Z. Y. Chen and D. R. Liu, J. Am. Chem. Soc., 2015, 137, 14084–14093 CrossRef PubMed; K. Quach, J. LaRochelle, X. H. Li, E. Rhoades and A. Schepartz, Bioorg. Med. Chem., 2017 DOI:10.1016/j.bmc.2017.11.008; K. Hu, W. Li, M. Yu, C. Sun and Z. Li, Bioconjugate Chem., 2016, 27, 2824–2827 CrossRef PubMed; Y. Tian, Y. Jiang, J. Li, D. Wang, H. Zhao and Z. Li, ChemBioChem, 2017, 18, 2087–2093 CrossRef PubMed.
- S. Grootjans, B. Hassannia, I. Delrue, V. Goossens, B. Wiernicki, Y. Dondelinger, M. J. M. Bertrand, D. V. Krysko, M. Vuylsteke, P. Vandenabeele and T. V. Berghe, Nat. Protoc., 2016, 11, 1444–1454 CrossRef PubMed.
- S. P. Brown and A. B. Smith, J. Am. Chem. Soc., 2015, 137, 4034–4037 CrossRef CAS PubMed.
- M. E. B. Smith, F. F. Schumacher, C. P. Ryan, L. M. Tedaldi, D. Papaioannou, G. Waksman, S. Caddick and J. R. Baker, J. Am. Chem. Soc., 2010, 132, 1960–1965 CrossRef CAS PubMed; C. R. Braun, J. Mintseris, E. Gavathiotis, G. H. Bird, S. P. Gygi and L. D. Walensky, Chem. Biol., 2010, 17, 1325–1333 CrossRef PubMed; J. Collins, J. Tanaka, P. Wilson, K. Kempe, T. P. Davis, M. P. McIntosh, M. R. Whittaker and D. M. Haddleton, Bioconjugate Chem., 2015, 26, 633–638 CrossRef PubMed; W. H. Jeong, H. Lee, D. H. Song, J. H. Eom, S. C. Kim, H. S. Lee, H. Lee and J. O. Lee, Nat. Commun., 2016, 7, 11031 CrossRef PubMed.
Footnote |
† Electronic supplementary information (ESI) available. See DOI: 10.1039/c7sc05109c |
|
This journal is © The Royal Society of Chemistry 2018 |
Click here to see how this site uses Cookies. View our privacy policy here.