DOI:
10.1039/C7SC05242A
(Edge Article)
Chem. Sci., 2018,
9, 2222-2229
Enhanced circular dichroism at elevated temperatures through complexation-induced transformation of a three-layer cyclophane with dualistic dynamic helicity†
Received
11th December 2017
, Accepted 23rd January 2018
First published on 23rd January 2018
Abstract
When two planes stacked one above the other are twisted, they provide a dynamic pair of helical conformations with (M)- or (P)-helicity. We designed a three-layer cyclophane that consists of two such dynamic pairs: the top and middle planes, and the middle and bottom planes. Hence, several global conformations could be created for the overall molecule, e.g., double-helical forms with a pair with the same helicity [(M,M) or (P,P)], and a meso-like form with a pair with a different helicity (M,P). These conformations dynamically interconvert to each other in solution. Chiroptical properties were given by the helical-sense preference of the double-helical forms, which was brought about through complexation with a chiral hydrogen-bonding guest. In terms of the conformational energy in a complexed state, when a desirable relationship between double-helical and meso-like forms was attained, complexation-induced circular dichroism was enhanced at elevated temperatures and decreased at lowered temperatures.
Introduction
The ability to control molecular states in solution is crucial to affect the molecular properties in solution. Considerable effort has been devoted to the design of smart molecules that can be shaped, aggregated or disaggregated in response to a change in the environment.1 Temperature is one of the most sensible stimuli to use to induce changes in solution properties, as represented by LCST (lower critical solution temperature) and UCST (upper critical solution temperature), and plays a role in the phase-transition process induced by a change in affinity of the solute for the solvent.2 Alternatively, an equilibrium between the conformations of a molecule is also desirable for changes in its properties in solution. Especially in dynamic chiral chemistry, the ability to control the molecular conformation in an equilibrium between two enantiomeric or diastereomeric forms with opposite senses of chirality is, in fact, the ability to control and modulate chiroptical properties.3 When an equilibrium is established between two conformations A and B that interconvert to each other, the ratio of populations is defined as the equilibrium constant K = [B]/[A], which changes with temperature. We can consider various cases for such a combination of A and B (Scheme 1). For example, we can assume a long-chained molecule with two conformations, a random form (A) and a helical form (B) (Scheme 1a). If the helical form is energetically favored, the population of the helical form will be preferred over the random form and the value of K will be greater than 1 at lower temperatures. A pair of helices with M-helicity (A) or P-helicity (B) can also be considered (Scheme 1b), though in this case the optical activities of the helices do not differ in intensity but rather have opposite signs. If some chiral source is present in this system, e.g., a chiral auxiliary group (*) is associated with the helix, a particular diastereomeric form can be preferred through the intramolecular transmission of chirality. In these cases, the value of K will increase at lower temperatures and decrease at elevated temperatures when ΔH is negative. Consequently, the chiroptical properties due to a diastereomeric excess between A and B are enhanced at lower temperatures and attenuated at elevated temperatures. This is the typical case for most conventional systems.4–6 Even with a driving force to bias the equilibrium by the formation of a supramolecular complex with a chiral additive through noncovalent interactions such as hydrogen bonding (supramolecular transmission of chirality), similar results are observed as long as the optically active form is more energetically-favored (Scheme 1c and d). In this regard, however, the increase in temperature is limited while the supramolecular complex remains formed. Since complexation is also defined with an equilibrium constant, a complexed form is favored at lower temperatures and disfavored at elevated temperatures in the case of ΔHcomplexation < 0. This leads to the same result that chiroptical properties are enhanced at lower temperatures.4b,d,5c,d,6b
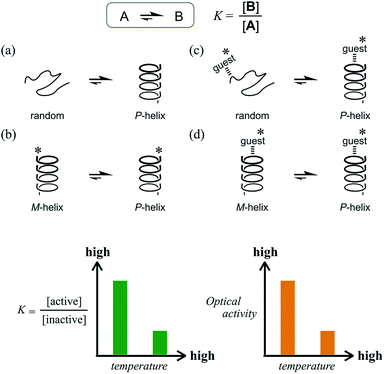 |
| Scheme 1 Dynamic conformational equilibrium between two forms, A (optically less active or inactive) and B (optically active). The equilibrium constant K decreases with temperature when ΔH is negative, and is accompanied by a decrease in optical activity with temperature. In this scheme, we assume a conformational preference for form B. (a) Random and helical forms; (b) a pair of diastereomeric forms with opposite senses; (c) and (d) in a complexed state. | |
What type of system is required to show an increase in chiroptical properties at elevated temperatures based on such a conformational equilibrium? Only a few successful examples have been reported, but they were based on a solvation/desolvation process.7 Instead, we envisioned a system where the optically inactive form is energetically favored compared to the optically active form (Scheme 2).
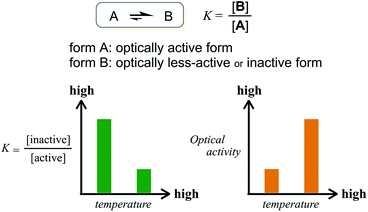 |
| Scheme 2 Design concept for the heat-induced increase in optical activity based on dynamic conformational equilibrium between two forms, A (optically active) and B (optically less-active or inactive). The equilibrium constant K decreases with temperature when ΔH is negative. In this scheme, we assume a conformational preference for form B. | |
Such a molecule should be allowed to adopt different conformations that can interconvert to each other. At least one of them can be chiral, and the other is relatively optically less-active or inactive.8 In this regard, we envisioned two-fold dynamic helicity arranged in a multi-layered structure.9–12 Different desired conformations would be generated through the motion of the two dynamic helical forms in a three-layer molecule. Each pair of planes arranged one above the other, i.e., the top and middle, and the middle and bottom, provides dynamic helicity with (M)- or (P)-helicity in terms of the direction of twisting.12,13a,b Movement of the top and bottom planes with respect to the middle plane would create double-helical forms [(M,M) or (P,P)] as well as a meso-like form (M,P).
The former are chiral and the latter is not chiral due to cancellation.14,15 If the latter form could be energetically favored over the former, this molecule could be a candidate. However, this is not a sufficient condition (as described later in detail, the equilibrium must involve different conformations with a desired relationship in terms of the conformational energy in a complexed state). The helical forms with (M)- or (P)-helicity are energetically equivalent, and optical activity can not emerge unless one form is favored. To break this balance, a transmission of chirality would be useful.3,13 When some chiral element is supramolecularly or intramolecularly attached to the molecule, a couple of double-helical forms are considered to be a diastereomeric pair with different conformational energies, and this would not be considered a meso-like form. The most essential point is the energetical relationship between the optically active and inactive forms under conditions where some chirality is transmitted to the molecule. If such a desired relationship could be attained (A: optically active and B: optically less-active or inactive), the energetically unfavorable form A would no longer be dominant in the equilibrium. However, its chiroptical properties could be significant and increase with an increase in the relative population with a rise in temperature (Scheme 2). The dominant form B should have only weak or silent chiroptical properties even though it constitutes the major population.
Thus, we designed a three-layered molecule 1 with a plane of hexakis(phenylethynyl)benzene (HPEB) stacked between two planes of 1,3,5-tris(phenylethynyl)benzene (TPEB). Each plane is correlated through a three-fold bridge (Fig. 1). Of the three planes in this molecule, two planes stacked one above the other can twist in one of two ways, either clockwise or counterclockwise, and can form a dynamic pair of enantiomeric conformations with (M)- or (P)-helicity. The three-layer 1 contains two such pairs: the top and middle planes, and the middle and bottom planes. Thus, the molecule could adopt either of two different conformations: a double-helical form with (M,M)- or (P,P)-helicity and a meso-like form with (M,P)-helicity. The former is chiral and can be more optically active than the latter if we can achieve a cancellation of chirality. We used a terephthalamide unit as a bridge.13 Two carbonyl groups of the terephthalamide can twist with respect to the central benzene core. Conrotatory twisting of the two amide groups is allowed in two directions, and can offer a dynamic pair of local enantiomeric conformations in the bridge. The direction of the local twisting could be controlled through a supramolecular transmission of point chirality when a chiral guest molecule is bound at the two carbonyl groups.13,16 A two-layer 2 was also designed and used as a model molecule. We used a chiral diammonium salt (S,S)-3 or (R,R)-3 as a guest molecule,13 which carried the helical-sense preference of the local dynamic helicity induced in the bridge through the formation of hydrogen bonds.
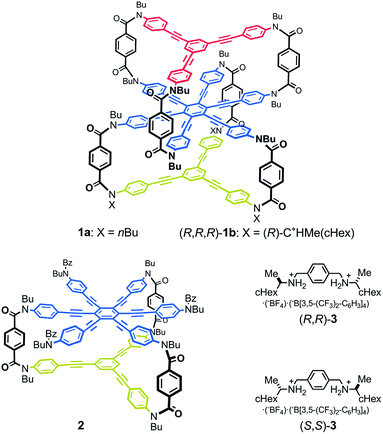 |
| Fig. 1 Chemical structures of 1a, (R,R,R)-1b, 2, and chiral ditopic guests (R,R)-3/(S,S)-3. | |
Results and discussion
Synthesis and dynamic structures of three-layer 1
We synthesized three-layer cyclophanes 1a and (R,R,R)-1b by a step-wise stacking strategy (Scheme 3), based on a three-fold condensation reaction between tricarboxylic acid chloride and trianiline. Two intermediate planes of TPEB with acid chlorides or anilines were derived from 4a/b or 5.13a Both carboxylic acid ester and BOC-protected aniline were attached to the periphery of HPEB 6, and respectively deprotected at the right time. Acid chlorides were derived from the corresponding carboxylic acid esters, followed by hydrolysis under basic conditions. Anilines were prepared by deprotection under acid conditions. We started with the first stacking of 4 and 6. The resulting two-layer 7a/b was ultimately stacked onto 5 to give three-layer 1. Two-layer model 2 was similarly obtained by stacking of 4 onto a benzoylated analog, instead of a BOC group of 6. The details of the new compound synthesis are summarized in Schemes S1 and S2.†
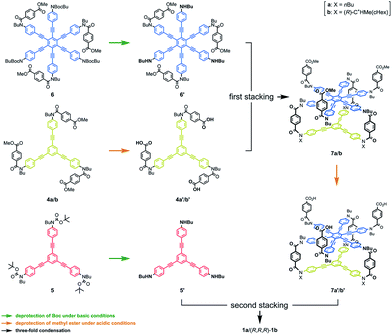 |
| Scheme 3 Chemical structures of synthetic intermediates 4–7, and the stepwise-stacking strategy for the synthesis of three-layer 1. | |
A conformational search for a three-layer model 1′ [NMe] revealed many conformers with a local minimum. All of the pairs of planes stacked one above the other in each conformer adopted a twisted form with (M)- or (P)-helicity, and every conformer could be classified as either a double-helical form (A) or meso-like form (B) in terms of global helicity. No conformer with D3d symmetry, as depicted in Fig. 1, was found within an energy-window of 100 kJ mol−1. Among the several conformers found in each group, the most energy-minimized structure is shown in Fig. 2. The conformational energy estimated for a meso-like form (B) was lower than that for a double-helical form (A). The details are summarized in Fig. S1.† Regarding the local conformation of the middle plane in group A, we found a chiral propeller with all six blades conrotatorily twisted in the same direction, and considered that conformers with double-helical forms are chiral. Alternatively, for conformers in group B, two blades located at para-position with respect to each other were twisted in a disrotatory direction. As a result, a centrosymmetric center was generated to give a nonpropeller form in the middle plane, and the conformers in group B are not globally chiral. Similar results were observed with a two-layer model 2′ (Fig. S2†).
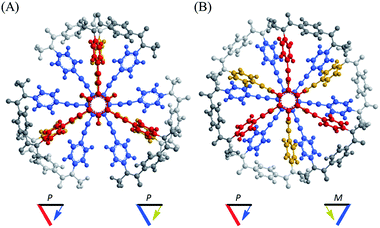 |
| Fig. 2 The most energy-minimized structures for a three-layer model 1′ [NMe] with (A) double-helical form (+28.2 kJ mol−1) and (B) meso-like form (rel. 0 kJ mol−1), obtained by a conformational search using MacroModel software (v9.9 OPLS_2005, Monte Carlo Multiple Minimum method, non-solvated, 50 000 steps). Views from the top (red), middle (blue) and bottom (yellow). Only one enantiomeric form with (P,P)-helicity is depicted in (A). | |
We investigated the dynamic structure of 1a in solution by VT measurements in 1H NMR spectroscopy (Fig. 3). At any temperature (223–323 K), only a single set of averaged resonances was recorded. Notably, the chemical shifts of these averaged resonances changed with temperature. We considered that this result, along with the results of the above conformational search, meant that different structures with different conformational energies were present in solution and they were dynamically interconvertible in equilibrium with changes in the population ratio. We reached a similar conclusion for two-layer model 2 (Fig. S3†). The changes in the chemical shift for 1a seemed more significant than those for 2 with only a single pair of global helical forms with (M)- or (P)-helicity.
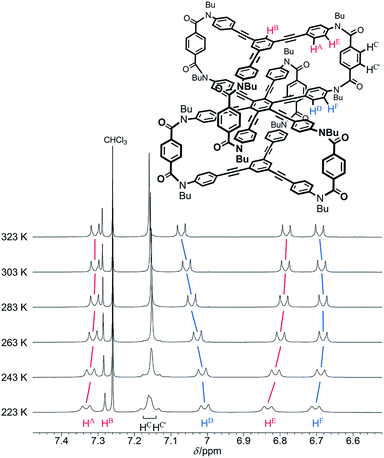 |
| Fig. 3 Partial VT 1H NMR spectra (400 MHz) of 1a, measured in chloroform-d at 223–323 K. Two protons HC and HC′ are assigned to peaks with a larger chemical shift and another, respectively. | |
Complexation of three-layer host 1a with a chiral guest
First, we describe the results with a simple model 2 (Fig. S4†). As a ditopic hydrogen-bonding guest, (R,R)-3 was added to a solution of 2, and the phenylene protons of both 2 and 3 (HD of 2 and Ha of 3) moved upfield (Fig. S4A†). This result showed that the ditopic guest was captured at some bridge through the formation of hydrogen bonds at two amide carbonyls in the bridge. Based on changes in the chemical shift for several protons of 2 and 3, Job plots were created to show that the stoichiometry was 1
:
2 for a complex of 2·3. Titration experiments, performed at various temperatures, showed that a sigmoidal change was induced upon 1
:
2 complexation in a positive allosteric manner (Fig. S4B†). The positive allosterism can be explained by assuming that a particular direction of twisting was induced for every three-fold bridge when the two planes were twisted. Binding constants K1:2/M−2 were estimated by a curve-fitting method17 to be 4.2 × 105 (273 K), 2.5 × 105 (293 K) and 2.1 × 105 (313 K).13a,b,18 Through a plot of the binding constants at different temperatures against 1/T, we confirmed that the change in enthalpy upon complexation was negative.
Next, we demonstrate the complexation of three-layer 1a with 3 (Fig. 4). We confirmed that the ditopic guest was bound at some bridge by the observation of upfield shifts, similar to those for 2 and 3, for the corresponding phenylene protons (HC and HC′ of 1a and Ha of 3) (Fig. 4a and b). Also, we found significant changes in the chemical shift for other protons far from the binding sites of 1a. These complexation-induced changes are believed to be indicative of a change in the environment due to the proximity of a guest and/or to changes in the populations of the contributors in equilibrium. In a series of titration curves, measured at 293 K (Fig. 4a), we found a turning point for some titration curves with the addition of around three or four equivalents of 3 (e.g., an upfield shift was initially induced, and later in titration, it turned in the opposite direction). Such points were also found at higher temperatures (303 K and 313 K, Fig. S5A†). While the chemical shifts changed little with temperature before the appearance of these points, greater changes with temperature were observed after their appearance. This observation was especially significant for phenylene protons (HA, HD, HE and HF). These results indicated that some change was induced in the relative direction of twisting of the top and bottom planes with respect to the middle plane, i.e., with a change in the populations of double-helical and meso-like forms, with the addition of more guest. For protons (HC and HC′) in the binding site of 1a, they were significantly differentiated before and after the points appeared. These results were considered to be characteristic of three-layer 1a, since there was no such point found in titration curves from two-layer 2. We considered that the appearance of these points was due to the possibility that energetically-minor conformations could emerge and contribute to the equilibrium in the presence of a sufficient amount of added guest.
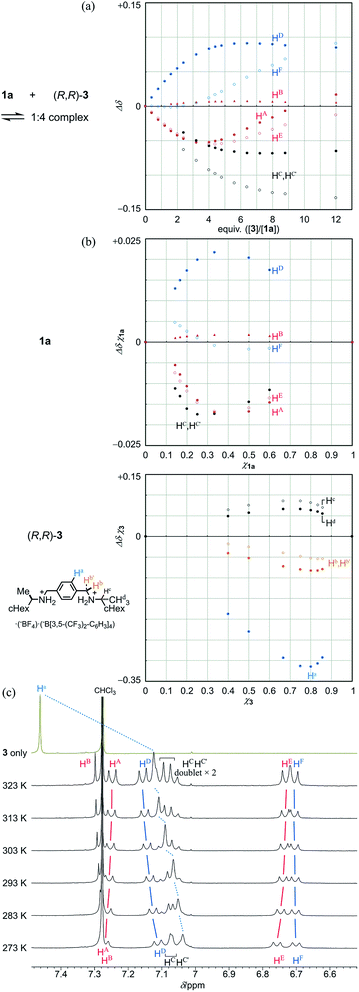 |
| Fig. 4 (a) Titration curves and (b) Job plots for the complexation of 1a with (R,R)-3 based on changes in the chemical shift (Δδ); (c) partial VT 1H NMR spectra (400 MHz) of 1a in the presence of (R,R)-3 ([3]/[1a] = 4), measured at 273–323 K. The 1H NMR spectrum of 3, measured at 293 K. All spectra were measured in chloroform-d containing 3 vol% acetonitrile-d3. Conditions for titration: [1a] = 3.3 × 10−4 M and [3] = 4.0 × 10−3 M, measured at 293 K. Conditions for Job plot: [1a] + [3] = 2 mM, measured at 293 K. | |
Due to these turning points, it did not seem to be appropriate to estimate the stoichiometry with these protons in the Job plots. Instead, we used only protons at the binding site, which were directly affected by the proximity of the guest, and assumed that the stoichiometry was 1
:
3 or 1
:
4 for a complex of 1a with 3 (Fig. 4b).19 The most important result was that the stoichiometry remained unchanged at 273 K or 313 K (Fig. S5B†). Although it is considered that a hydrogen-bonded complex is often unfavorable at elevated temperatures, the current complex remained intact even when the temperature was raised to 313 K. This was supported by VT measurements in the presence of 3 in a ratio of 1
:
4 (Fig. 4c). As the temperature was increased, a slight change toward downfield was induced for the central phenylene protons Ha in 3. However, we concluded that the guest remained captured in a complex at elevated temperatures, based on a comparison to the original chemical shift of 3 itself, as well as a similar comparison regarding the phenylene protons HC and HC′ in the bridge of 1a (Fig. 3 and 4c). For other protons far from the binding site, we observed changes in the chemical shift induced for a set of averaged resonances with temperature. This result showed that different conformers could be present in the equilibrium even in a complexed state. The spectra measured at lower temperatures seemed similar to those of 1a itself (Fig. 3), which indicated that the major contributor with a more energetically-favored form remained unchanged between before and after complexation. On the other hand, the appearance of the spectrum changed at elevated temperatures. This change was considered to reflect the possibility that conformers with both globally twisted and locally twisted forms (cf. Fig. S1†), which could not appear in the absence of a guest, were newly involved in the equilibrium in a complexed state.
Complexation-induced circular dichroism (CICD) of three-layer 1 and a temperature-dependent change in CICD
We start with a discussion of absorption prior to circular dichroism (CD). The UV spectra, measured in dichloromethane, of three-layer 1a, two-layer 2, and substructures 8a13a and 9a13c are shown in Fig. 5. The absorptions of 1a and 2 were similar in appearance and composed of two bands [λmax 301 nm (log
ε 5.35) and 373 nm (4.94) for 1a, 293 nm (5.14) and 369 nm (5.08) for 2]. These two bands were assigned to substructures TPEB 8a [313 nm (5.02)] and HPEB 9a [367 nm (5.21)], respectively. The latter absorption gradually decreased in intensity and shifted toward a longer wavelength region for 2 and 1a, in this order, compared to 9a. We considered that this result could be attributed to a change in the coplanarity of blades with respect to the central benzene ring in HPEB,13c,d,20 which would be slightly higher in 1a, where the corresponding middle plane is located between the planes of the top and bottom.
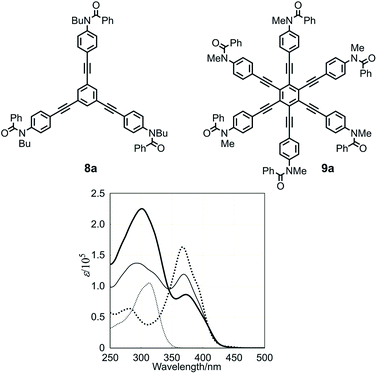 |
| Fig. 5 Chemical structures of 8a (thin dotted line) and 9a (bold dotted line), and UV-vis spectra of 1a (bold solid line), 2 (thin solid line), 8a (thin dashed line) and 9a (bold dashed line), measured in dichloromethane at 293 K. | |
Next, we demonstrate CICD of three-layer 1a, which has no chiral element other than two-fold dynamic helicity. When 1a was mixed with a chiral ditopic guest (S,S)-3 in dichloromethane, an absorption at 373 nm gradually reduced with the addition of up to three equivalents, and then new bands appeared at a longer wavelength region (Fig. 6a). In the CD spectrum, a couple of bisignated Cotton effects were induced throughout the entire region of absorption of 1a (Fig. 6b). Cotton effects induced in the longer wavelength region indicated some change in the middle plane, e.g., a propeller conformation emerged in the equilibrium in a complexed state, which led us to assume that double-helical forms could be involved in a complexed state. Mirror-images were given by the addition of antipodal guest (R,R)-3. Based on these results, we were certain that dynamic helical forms were present in solution, and a particular sense was preferred in response to chirality in the guest through the supramolecular transmission of chirality.
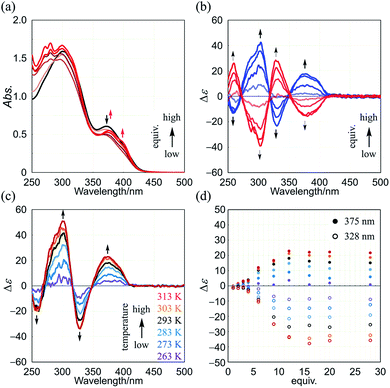 |
| Fig. 6 (a) UV-vis spectra of 1a (7.1 × 10−5 M) in the presence of (S,S)-3 [0 equiv. (1a only, black line), 1, 3, 6, 8 and 12 equiv. of (S,S)-3 (red lines)], measured at room temperature; (b) CD spectra of 1a (7.1 × 10−5 M) in the presence of a chiral guest [3, 6, 8 and 12 equiv. of (S,S)-3 (red lines) or (R,R)-3 (blue lines)], measured at 293 K; (c) VT CD spectra of 1a (7.4 × 10−5 M) in the presence of (R,R)-3 (12 equiv.), measured at 263–313 K; (d) titration curves for the complexation of 1a (7.4 × 10−5 M) with (R,R)-3 (1–28 equiv.), based on induced Cotton effects (Δε) at 375 (●) nm and 328 (○) nm, measured at 263–313 K. All spectra were measured in dichloromethane. | |
We investigated the effect of temperature on complexation-induced CD through VT measurements (263–313 K) for a complex of 1a·(R,R)-3, which was obtained by mixing in a ratio of 1
:
12 in dichloromethane (Fig. 6c). At 263 K, only small Cotton effects were found. Above 273 K, Cotton effects gradually increased with an increase in temperature. These results were explained as follows. At lower temperatures, a meso-like form was dominant in a complexed state. As the temperature was increased, double-helical forms could contribute to the equilibrium even though they were energetically disfavored.
The results of titration experiments at different temperatures showed that this system can be considered an advanced allosteric system, where chiroptical signals are sigmoidally enhanced with addition of a guest18 as well as an increase in temperature (Fig. 6d and S6†). This seems consistent with the fact that a turning point was also found in titration experiments by NMR or UV spectroscopy.
As a control, similar experiments were performed with two-layer 2 (Fig. S7†). We confirmed that dynamic helical forms with (M)- or (P)-helicity were present in solution through the observation of mirrored Cotton effects that were induced in the absorption region of 2 upon the addition of chiral guest 3. These Cotton effects were attenuated at elevated temperatures, as is often seen in conventional systems.4–6
Finally, we describe the results with three-layer (R,R,R)-1b, in which a three-fold chiral auxiliary group (R) is attached to one of the two planes of TPEB (Fig. 1). In the CD spectrum of (R,R,R)-1b, a couple of small and negatively-signed Cotton effects were observed in two regions of absorption (Fig. S8a†). The spectrum showed almost no change with a change in temperature (Fig. S8b†). Cotton effects in a shorter wavelength region were attributed to a local chiral environment around the auxiliary itself, since they were observed in the spectra of both substructures TPEB (R,R,R)-8b13a and HPEB (R,R,R)-9b.13d The Cotton effects in a longer wavelength region were small, which could be explained as follows. The intramolecular transmission of chirality (R) to dynamic helical forms of 1b may not have been particularly effective due to a local conformational preference for a nonhelical form in the bridge. If so, the molecule should be optically less-active regardless of any global conformation of double-helical forms or a meso-like form.
Although the Cotton effects in the CD spectrum of (R,R,R)-1b itself were small, they were greatly enhanced in the presence of a chiral ditopic guest (S,S)-3 (Fig. 7a). The induced Cotton effects were similar to those for a complex 1a·(S,S)-3. The antipodal guest did not induce mirror images, but showed nearly identical Cotton effects (Fig. S9†). These results showed that double-helical forms were present in a complexed state, and the helical-sense preference was controlled by the internal chirality (R) associated with 1b regardless of the external chirality of a guest. For a successful transmission of chirality, it would be essential for the binding site to adopt local dynamic helical forms with (m)- or (p)-helicity in the bridge, which were generated by complexation with a ditopic guest. These Cotton effects did not decrease with temperature (Fig. 7b).
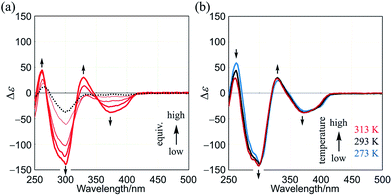 |
| Fig. 7 (a) CD spectra of (R,R,R)-1b (6.3 × 10−5 M) in the presence or absence of (S,S)-3 [0 (1b only, black dashed line), 1, 3, 6 and 12 equiv. of (S,S)-3 (red solid lines)], measured at 293 K; (b) VT CD spectra of (R,R,R)-1b in the presence of (S,S)-3 (12 equiv.), measured at 273, 293 and 313 K. All spectra were measured in dichloromethane. | |
Conclusions
We have demonstrated the effect of temperature on complexation-induced Cotton effects of three-layer cyclophanes. We found an unconventional enhancement of CICD at elevated temperatures. The three-layer cyclophanes adopted different global conformations: double-helical forms with pairs of identical helicity [(M,M) or (P,P)], and a meso-like form with a pair of different helicity [(M,P)]. In addition, twisting of planes generated several local conformations in the bridge [(m)-, (p)- and (n)]. A preferred sense of double-helical forms was brought about through the supramolecular transmission of chirality in the bridge. An energetical relationship among these conformations played an essential role.
In the absence of any guest molecule, a locally nonhelical form was favored in the bridge, as shown by VT NMR and/or CD spectra. Alternatively, local helical forms could be present in a complexed state, and a transmission of chirality was enabled. However, dynamic helical forms are not believed to be dominant in a complexed state. If they had been, CICD would have been attenuated at elevated temperatures, as is seen in most conventional systems. Fortunately, in the current system, a desirable relationship was attained in a complexed state of the three-layer cyclophane with dualistic dynamic helicity.
Conflicts of interest
There are no conflicts to declare.
Notes and references
-
(a) X. Yan, F. Wang, B. Zheng and F. Huang, Chem. Soc. Rev., 2012, 41, 6042 RSC;
(b) Y. Pei, A. B. Lowe and P. J. Roth, Macromol. Rapid Commun., 2017, 38, 1600528 CrossRef PubMed.
-
(a) D. Roy, W. L. A. Brooks and B. S. Sumerlin, Chem. Soc. Rev., 2013, 42, 7214 RSC;
(b) Y. Kotsuchibashi, M. Ebara, T. Aoyagi and R. Narain, Polymers, 2016, 8, 380 CrossRef.
-
(a) S. M. Morrow, A. J. Bissette and S. P. Fletcher, Nat. Nanotechnol., 2017, 12, 410 CrossRef CAS PubMed;
(b) E. Yashima, N. Ousaka, D. Taura, K. Shimomura, T. Ikai and K. Maeda, Chem. Rev., 2016, 116, 13752 CrossRef CAS PubMed.
-
(a) R. B. Prince, L. Brunsveld, E. W. Meijer and J. S. Moore, Angew. Chem., Int. Ed., 2000, 39, 228 CrossRef CAS;
(b) V. Setnička, M. Urbanová, K. Volka, S. Nampally and J.-M. Lehn, Chem.–Eur. J., 2006, 12, 8735 CrossRef PubMed;
(c) T. Mizutani, S. Yagi, T. Morinaga, T. Nomura, T. Takagishi, S. Kitagawa and H. Ogoshi, J. Am. Chem. Soc., 1999, 121, 754 CrossRef CAS;
(d) H. Goto, H. Katagiri, Y. Furusho and E. Yashima, J. Am. Chem. Soc., 2006, 128, 7176 CrossRef CAS PubMed;
(e) J. Yan, K. Liu, W. Li, H. Shi and A. Zhang, Macromolecules, 2016, 49, 510 CrossRef.
-
(a) S. J. George, Ž. Tomović, M. M. J. Smulders, T. F. A. de Greef, P. E. L. G. Leclère, E. W. Meijer and A. P. H. J. Schenning, Angew. Chem., Int. Ed., 2007, 46, 8206 CrossRef PubMed;
(b) T. Shiraki, A. Dawn, Y. Tsuchiya and S. Shinkai, J. Am. Chem. Soc., 2010, 132, 13928 CrossRef PubMed;
(c) H. Yamada, Z.-Q. Wu, Y. Furusho and E. Yashima, J. Am. Chem. Soc., 2012, 134, 9506 CrossRef PubMed;
(d) R. Sakai, I. Otsuka, T. Satoh, R. Kakuchi, H. Kaga and T. Kakuchi, Macromolecules, 2006, 39, 4032 CrossRef.
-
(a) E. T. Chernick, G. Börzsönyi, C. Steiner, M. Ammon, D. Gessner, S. Frühbeißer, F. Gröhn, S. Maier and R. R. Tykwinski, Angew. Chem., Int. Ed., 2014, 53, 310 CrossRef PubMed;
(b) T. Luo and K. L. Kiick, J. Am. Chem. Soc., 2015, 137, 15362 CrossRef PubMed.
-
(a) G. Kwak and T. Masuda, Polymer, 2002, 43, 665 CrossRef;
(b) N. Saito, H. Kobayashi and M. Yamaguchi, Chem. Sci., 2016, 7, 3574 RSC.
-
(a) B. Kersting, M. Meyer, R. E. Powers and K. N. Raymond, J. Am. Chem. Soc., 1996, 118, 7221 CrossRef;
(b) P. R. Ashton, S. E. Boyd, S. Menzer, D. Pasini, F. M. Raymo, N. Spencer, J. F. Stoddart, A. J. P. White, D. J. Williams and P. G. Wyatt, Chem.–Eur. J., 1998, 4, 299 CrossRef;
(c) K. Utsumi, T. Kawase and M. Oda, Chem. Lett., 2003, 32, 412 CrossRef;
(d) T. Kawase, Y. Daifuku, Y. Hirao, K. Matsumoto, H. Kurata and T. Kubo, C. R. Chim., 2009, 12, 403 CrossRef CAS.
-
(a) P. Blanchard, N. Svenstrup, J. Rault-Berthelot, A. Riou and J. Becher, Eur. J. Org. Chem., 1998, 1743 CrossRef CAS;
(b) M. M. Naseer, D.-X. Wang, L. Zhao and M.-X. Wang, Eur. J. Org. Chem., 2014, 7895 CrossRef CAS;
(c) N. Hafezi, J. M. Holcroft, K. J. Hartlieb, E. J. Dale, N. A. Vermeulen, C. L. Stern, A. A. Sarjeant and J. F. Stoddart, Angew. Chem., Int. Ed., 2015, 54, 456 CAS;
(d) Q. Wang, C. Yu, H. Long, Y. Du, Y. Jin and W. Zhang, Angew. Chem., Int. Ed., 2015, 54, 7550 CrossRef CAS PubMed;
(e) M. Servalli, N. Trapp, M. Wörle and F.-G. Klärner, J. Org. Chem., 2016, 81, 2572 CrossRef CAS PubMed.
-
(a) J. K. Klosterman, Y. Yamauchi and M. Fujita, Chem. Soc. Rev., 2009, 38, 1714 RSC;
(b) A. J. Hubert, J. Chem. Soc. C, 1967, 13 RSC;
(c) N. Sendhoff, W. Kißener, F. Vögtle, S. Franken and H. Puff, Chem. Ber., 1988, 121, 2179 CrossRef CAS;
(d) K. Yano, S. Matsuda, K. Tani, K. Yamamoto and H. Matsubara, Bull. Chem. Soc. Jpn., 1999, 72, 2111 CrossRef CAS;
(e) A. Muranaka, M. Shibahara, M. Watanabe, T. Matsumoto, T. Shinmyozu and N. Kobayashi, J. Org. Chem., 2008, 73, 9125 CrossRef CAS PubMed;
(f) D. Sakamaki, A. Ito, K. Tanaka, K. Furukawa, T. Kato and M. Shiro, Angew. Chem., Int. Ed., 2015, 51, 8281 CrossRef PubMed;
(g) F. Ren, K. J. Day and C. S. Hartley, Angew. Chem., Int. Ed., 2016, 55, 8620 CrossRef CAS PubMed;
(h) K. Yamashita, M. Ikeda, M. Takeuchi and S. Shinkai, Chem. Lett., 2003, 32, 264 CrossRef CAS;
(i) H.-G. Jin, X. Jiang, I. A. Kühne, S. Clair, V. Monnier, C. Chendo, G. Novitchi, A. K. Powell, K. M. Kadish and T. S. Balaban, Inorg. Chem., 2017, 56, 4864 CrossRef CAS PubMed.
-
(a) M. A. Mateos-Timoneda, M. Crego-Calama and D. N. Reinhoudt, Chem.–Eur. J., 2006, 12, 2630 CrossRef CAS PubMed;
(b) H. Y. Lee, J. Park, M. S. Lah and J.-I. Hong, Chem. Commun., 2007, 5013 RSC;
(c) H. Lee, T. H. Noh and O.-S. Jung, Angew. Chem., Int. Ed., 2013, 52, 11790 CrossRef CAS PubMed;
(d) J. R. Néabo, S. Rondeau-Gagné, C. Vigier-Carrière and J.-F. Morin, Langmuir, 2013, 29, 3446 CrossRef PubMed.
-
(a) Y. Rubin, T. C. Parker, S. I. Khan, C. L. Holliman and S. W. McElvany, J. Am. Chem. Soc., 1996, 118, 5308 CrossRef CAS;
(b) P. Rajakumar and M. Srisailas, Tetrahedron Lett., 2002, 43, 1909 CrossRef CAS;
(c) Y. Wu, M. Frasconi, D. M. Gardner, P. R. McGonigal, S. T. Schneebeli, M. R. Wasielewski and J. F. Stoddart, Angew. Chem., Int. Ed., 2014, 53, 9476 CrossRef CAS PubMed;
(d) C. Zhang, Q. Wang, H. Long and W. Zhang, J. Am. Chem. Soc., 2011, 133, 20995 CrossRef CAS PubMed.
-
(a) R. Katoono, K. Fujiwara and T. Suzuki, Chem. Commun., 2014, 50, 5438 RSC;
(b) R. Katoono, S. Kawai and T. Suzuki, Chem. Sci., 2016, 7, 3240 RSC;
(c) R. Katoono, K. Kusaka, S. Kawai, Y. Tanaka, K. Hanada, T. Nehira, K. Fujiwara and T. Suzuki, Org. Biomol. Chem., 2014, 12, 9532 RSC;
(d) R. Katoono, H. Kawai, M. Ohkita, K. Fujiwara and T. Suzuki, Chem. Commun., 2013, 49, 10352 RSC.
- Although there has been much interest in the subject of chirality, meso compounds have not received much attention due to their inactivity with respect to chiroptical properties.
-
(a) P. J. Chmielewski, L. Szterenberg and M. Siczek, Chem.–Eur. J., 2011, 17, 1009 CrossRef CAS PubMed;
(b) A. Meyer, K. Schlögl, U. Lerch and E. Vogel, Chem. Ber., 1988, 121, 917 CrossRef CAS;
(c) T. Ueda, T. Adachi, K. Sumiya and T. Yoshida, J. Chem. Soc., Chem. Commun., 1995, 935 RSC;
(d) L. Ernst and L. Wittkowski, Eur. J. Org. Chem., 1999, 1653 CrossRef CAS;
(e) W. Tochtermann, D. Kuckling, C. Meints, J. Kraus and G. Bringmann, Tetrahedron, 2003, 59, 7791 CrossRef CAS;
(f) T. Ogoshi, D. Yamafuji, T. Aoki, K. Kitajima, T. Yamagishi, Y. Hayashi and S. Kawauchi, Chem.–Eur. J., 2012, 18, 7493 CrossRef CAS PubMed;
(g) B. Kiupel, C. Niederalt, M. Nieger, S. Grimme and F. Vögtle, Angew. Chem., Int. Ed., 1998, 37, 3031 CrossRef CAS;
(h) R. S. Walters, C. M. Kraml, N. Byrne, D. M. Ho, Q. Qin, F. J. Coughlin, S. Bernhard and R. A. Pascal Jr, J. Am. Chem. Soc., 2008, 130, 16435 CrossRef CAS PubMed;
(i) J. M. Cross, R. V. Fennessy, L. P. Harding, C. R. Rice, M. J. Hardie and C. Slater, Chem. Commun., 2013, 49, 11290 RSC;
(j) X. Liu, P. Yu, L. Xu, J. Yang, J. Shi, Z. Wang, Y. Cheng and H. Wang, J. Org. Chem., 2013, 78, 6316 CrossRef CAS PubMed.
- Disrotatory twisting of the two amide carbonyls leads to a local nonhelical form (n) in the bridge. When the binding site adopted this form, the transmission of chirality was not effective (Fig. S1†).
-
S. Akine, TitrationFit, program for analyses of host–guest complexation, Kanazawa University, Kanazawa, Japan, 2013 Search PubMed.
-
(a) S. Shinkai, M. Ikeda, A. Sugasaki and M. Takeuchi, Acc. Chem. Res., 2001, 34, 494 CrossRef PubMed;
(b) M. Takeuchi, M. Ikeda, A. Sugasaki and S. Shinkai, Acc. Chem. Res., 2001, 34, 865 CrossRef CAS PubMed;
(c) J. S. Park, F. L. Derf, C. M. Bejger, V. M. Lynch, J. L. Sessler, K. A. Nielsen, C. Johnsen and J. O. Jeppesen, Chem.–Eur. J., 2010, 16, 848 CrossRef CAS PubMed;
(d) X. He, X.-B. Xu, X. Wang and L. Zhao, Chem. Commun., 2013, 49, 7153 RSC;
(e) D. Bardelang, G. Casano, F. Poulhès, H. Karoui, J. Filippini, A. Rockenbauer, R. Rosas, V. Monnier, D. Siri, A. Gaudel-Siri, O. Ouari and P. Tordo, J. Am. Chem. Soc., 2014, 136, 17570 CrossRef CAS PubMed;
(f) K.-I. Hong, H. Yoon and W.-D. Jang, Chem. Commun., 2015, 51, 7486 RSC.
- Although the phenylene protons HC and HC′ are no longer inherently exchangeable, here we considered them as an average for this plot due to a difference in patterns before (singlet) and after (doublet ×2 to be expected) the appearance of a turning point.
- K. Sakajiri, T. Sugisaki, K. Moriya and S. Kutsumizu, Org. Biomol. Chem., 2009, 7, 3757 CAS.
Footnote |
† Electronic supplementary information (ESI) available: Details of energy-minimized structures, NMR and CD spectroscopic data (Fig. S1–S9), and experimental details of new compound synthesis (Schemes S1 and S2). See DOI: 10.1039/c7sc05242a |
|
This journal is © The Royal Society of Chemistry 2018 |
Click here to see how this site uses Cookies. View our privacy policy here.