DOI:
10.1039/C8SC02677G
(Edge Article)
Chem. Sci., 2018,
9, 8446-8452
Thermo- and photo-modulation of exciplex fluorescence in a 3D spin crossover Hofmann-type coordination polymer†
Received
18th June 2018
, Accepted 7th September 2018
First published on 11th September 2018
Abstract
The search for bifunctional materials showing synergies between spin crossover (SCO) and luminescence has attracted substantial interest since they could be promising platforms for new switching electronic and optical technologies. In this context, we present the first three-dimensional FeII Hofmann-type coordination polymer exhibiting SCO properties and luminescence. The complex {FeII(bpben)[Au(CN)2]}@pyr (bpben = 1,4-bis(4-pyridyl)benzene) functionalized with pyrene (pyr) guests undergoes a cooperative multi-step SCO, which has been investigated by single crystal X-ray diffraction, single crystal UV-Vis absorption spectroscopy, and magnetic and calorimetric measurements. The resulting fluorescence from pyrene and exciplex emissions are controlled by the thermal and light irradiation (LIESST effect) dependence of the high/low-spin state population of FeII. Conversely, the SCO can be tracked by monitoring the fluorescence emission. This ON–OFF interplay between SCO and luminescence combined with the amenability of Hofmann-type materials to be processed at the nano-scale may be relevant for the generation of SCO-based sensors, actuators and spintronic devices.
Introduction
Iron(II) spin crossover (SCO) complexes are responsive materials that switch between high-spin (HS) and low-spin (LS) states triggered by external stimuli (temperature, pressure, light, and analytes). This binary HS ↔ LS behaviour entails strong electron–phonon coupling that involves drastic changes in the magnetic, electric, optical and mechanical properties of the SCO material when cooperative elastic interactions between the SCO centres are effective.1 Cooperative SCO properties have attracted substantial interest specially when combined in a synergistic way with other relevant physical properties (i.e. electronic transport, chirality, luminescence, host–guest chemistry, etc) because the intrinsic ON–OFF nature of SCO may be transferred to the second property. This fact, together with the amenability of the resulting functional material to be processed at micro- and nano-scale levels, has created important expectations for the discovery of new switching electronic and optical technologies.2
Luminescence is a relevant property that may be used as an internal probe to report upon the spin state of SCO centres and conversely the control of the spin state may be used to modulate the luminescent signal. In this respect, it has been stated that the search for synergies between SCO and luminescence could be an important platform for thermometry and thermal imaging technologies.3 Although, the interplay between SCO and luminescence was first reported 20 years ago,4 it has been more recently that this binomial has gained much attention. Two main approaches have been designed to combine SCO and fluorescence in the same material. A first approach introduces fluorescence as an extrinsic property by physically doping the targeted SCO complex with a fluorescent dye during the fabrication of thin films or nanoparticles.5–10 In the second approach, luminescence is an intrinsic property of the SCO complex. Only a reduced number of examples of this second approach have been reported so far, which include discrete mononuclear11–14 homodinuclear,15 heterodinuclear FeII–EuIII,4 heterotrinuclear FeII–PtII,16 and two 1-D coordination polymers.17,18
Hofmann-type FeII SCO coordination polymers (SCO-CP)19,20 have afforded excellent examples of thermo-21 and piezo-hysteretic behaviours,22 multistep cooperative transitions,23 relevant examples of porous systems where the SCO can be tuned by guest molecules favouring selective host–guest interactions,24 post-synthetic chemical activity25 and/or solid-state transformations.26 In addition, they have demonstrated to be excellent platforms to investigate the SCO properties at the nano-scale (nanocrystals and thin films) as potential components for the generation of SCO-based sensors, actuators and spintronic devices.27 In this context, and as a result of our systematic study on Hofmann-type FeII SCO-CP,19 here we report on the thermo- and photo-induced multi-stable spin transition of a pyrene based 3-D Hofmann clathrate monitored by photo-luminescence and on the corresponding thermo- and photo-modulation of the exciplex fluorescence emission. This study has been carried out based on single crystal UV-Vis absorption spectroscopy measurements, X-ray single crystal analysis at relevant temperatures and magnetic, photo-magnetic and calorimetric measurements on microcrystalline samples of the SCO-MOF {FeII(bpben)[Au(CN)2]}@pyr [1Fe@pyr] (bpben = 1,4-bis(4-pyridyl)benzene; pyr = pyrene). For comparative reasons the homologous isostructural ZnII derivative 1Zn@pyr was also synthesised and characterised and herein reported. As far as we know this is the first SCO-CP implemented with luminescence properties.
Results and discussion
Synthesis and structure
Pale-yellow (1Fe@pyr) or white (1Zn@pyr) crystalline samples were obtained by slow diffusion methods from stoichiometric amounts of MII(BF4)2·6H2O (MII = Fe, Zn), bpben and K[Au(CN)2] and an excess of pyrene dissolved in methanol (see the ESI†).
Single crystal structure analysis of 1Fe@pyr and 1Zn@pyr at 120 and 280 K showed the non-centrosymmetric monoclinic space group Cc. Crystal data and structural parameters are given in Tables S1–S3 in the ESI.†
There are two crystallographically distinct octahedral [MIIN6] sites constituted of 4 equatorial [Au(CN)2]− groups and 2 axial bpben ligands (Fig. S1†). The almost linear [Au(CN)2]− groups bridge 4 equivalent [MIIN6] sites forming parallel stacks of {M4II[Au(CN)2]4}n 2D grids pillared by the bpben ligands, thus generating an open framework with pcu topology (Fig. 1). The wide {M4II[Au(CN)2]4} windows allow interpenetration of an identical framework made up of the other crystallographically independent [MIIN6] site (Fig. 1). This type of structure is common for {FeII(L)[Au(CN)2]} SCO compounds where L is a bis-monodentate pyridine-type ligand.19,20
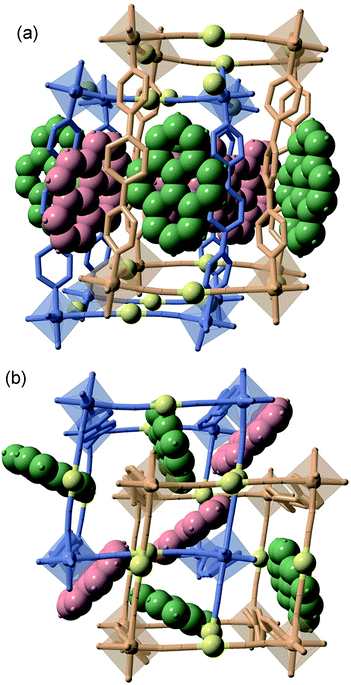 |
| Fig. 1 Two perpendicular perspective views ((a) side view and (b) top view) of the same fragment of the doubly interpenetrated frameworks 1M@pyr (MII = Fe, Zn) showing the bpben pillars, coordination centres and pyrene units. The two crystallographically distinct frameworks are represented in blue (network 1) and beige (network 2) colours, while the different pyrene molecules are depicted in red (pyr1) and green (pyr2) colours. Au centres are yellow spheres. | |
The two frameworks are held together by strong Au⋯Au interactions [average 3.0535(5) Å for 1Fe@pyr and 3.1086(9) Å for 1Zn@pyr at 120 K]. The average Fe–N bond length equal to 1.960 Å at 120 K and 2.154 Å at 280 K are typical of the FeII ion in the LS and HS states, respectively. Thus, the average bond length variation associated with the spin-state change, 0.2 Å, is consistent with the occurrence of a complete LS ↔ HS conversion. This is in turn reflected in a change of the unit cell volume of 444.7 Å3, which represents 6.7% of the HS unit cell volume or a volume change of 55.6 Å3 per FeII upon SCO. This is a typical value observed for Hofmann clathrates.19 It is worth noting that, in the same interval of temperatures, a unit cell volume variation of 1% (68.4 Å3) is observed for 1Zn@pyr, which essentially corresponds to thermal contraction/expansion of the crystal in the temperature interval 280–120 K where the average ZnII–N bond length remains practically constant at ca. 2.145 Å.
The available space generated by the two interpenetrating frameworks is filled by two crystallographically independent pyr guest molecules that do not interact significantly with each other. In contrast, they define a large number of short π–π interactions with the bpben ligands (Fig. 2 and Table S4†). The number and strength of these interactions are unequally distributed in each framework. The bpben1 pillars attached to the [Fe1N6] nodes (blue colour, Fig. 1 and 2) define with the corresponding pyr1 guests (red colour) a face-to-face type interaction with 22 short C⋯C contacts smaller than the sum of the van der Waals radius (ca. 3.7 Å) ranging in the interval [3.44–3.68 Å] at 280 K (Table S4, Fig. S2†). They markedly increase in number, up to 37 C⋯C contacts [3.30–3.66 Å], in the LS structure at 120 K (Fig. 2). It is worth noting that this increase in short π–π interactions occurs synchronically with a small in-plane rotation of the pyr1 by ca. 6.1° (Fig. S2†). In contrast, 22 [3.32–3.69 Å] and 12 [3.42–3.68 Å] short C⋯C contacts at 120 K and 280 K, respectively, are found between the bpben2 pillars attached to the [Fe2N6] nodes (beige colour, Fig. 1 and 2) and the corresponding pyr2 (green colour). This ca. 50% decrease of C⋯C interactions is due to parallel-displaced π–π interactions in the latter. Although similar considerations can be made for 1Zn@pyr, the larger ionic radius of ZnII with respect to FeII-LS is reflected in a slight reduction in the number of π–π interactions (Table S4-bis, Fig. S2†).
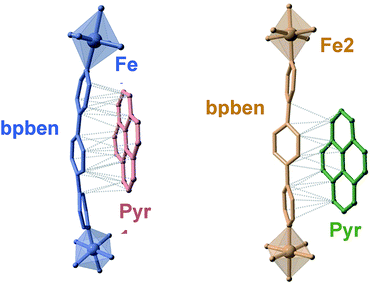 |
| Fig. 2 Crystallographically different bpben-pyrene pairs found in 1Fe@pyr displaying the intermolecular π–π interactions (dotted lines) between bpben and pyrene (only short π–π contacts smaller than the sum of the C⋯C van der Waals radius ca. 3.7 Å are shown). | |
A direct consequence of these strong π–π interactions is the bent geometry adopted by the bpben ligands. A similar situation was described for the SCO Hofmann clathrates {Fe(bpb)[MII(CN)4]}·2G, where the related rod-like bridging ligand bis(4-pyridyl)butadiyne (bpb) is markedly bent due to strong intermolecular interactions established with G = naphthalene or nitrobenzene molecules.28 For the titular compounds, we have estimated the magnitude of the bent in bpben measuring the distance of the pyridyl N atoms to the average plane defined by the central benzene ring (see Table S5a and Scheme I†). It is important to note that the bent, and consequently the distance to the average plane, is larger for bpben1 than for bpben2 due to the larger number and more direct π–π interactions with pyr in the former. In addition to this, the pyridyl groups are slightly rotated with respect to the central benzene ring (Table S5b†). Both, the bent distortion and the angles defined by the intersection of the benzene and pyridyl rings do not change significantly upon the spin state change of FeII. Furthermore, these distances and angles are consistent with those found for 1Zn@pyr. It is worth mentioning that the bent geometry found for the bpben pillars in the titular compounds strongly contrasts with the linear structure described previously for the solvate [Fe(bpben){Au(CN)2}2]·S with S = 1.5DMF·0.3EtOH·0.2C6H12l.29
Spin crossover behaviour
Magnetic, photo-magnetic and calorimetric studies.
The SCO was followed by magnetic measurements on bulk samples in the temperature interval 10–300 K (Fig. 3). At room temperature, the χMT product (χM = molar magnetic susceptibility and T = temperature) equal to 3.64 cm3 K mol−1 is consistent with the FeII ion in the HS state. On cooling at a temperature scan rate of 1 K min−1, χMT drops abruptly between 250 and 160 K, in three consecutive steps centred at 227, 195 and 180 K, to 0.14 cm3 K mol−1 indicating a practically complete transformation to the LS state. In the heating mode, the magnetic curve does not match the cooling curve showing the presence of hysteresis in the second and third steps ca. 5 K wide. The overall equilibrium temperature, T1/2, at which the HS and LS molar fractions are equal to 0.5 (γHS = γLS = 0.5; ΔG = 0) is estimated to be centred at 190 K. This multi-step SCO behaviour is reminiscent of the one previously observed for the homologue solvate [Fe(bpben){Au(CN)2}2]·S with S = 0.9 benzene, which undergoes a less steep three-step conversion centred at 214 K, 155 K and 141 K.29 The occurrence of multi-step SCO is a relatively uncommon event in SCO that results from a competition between ferro- and antiferro-elastic interactions driven by the difference of volume associated with the LS and HS centres.30 The steps involve stabilization of states characterized by different commensurate and/or incommensurate ordered fractional distributions of the HS/LS sites that often require symmetry breaking and that dramatically depend on subtle structural changes of the solid, which are difficult to control.31
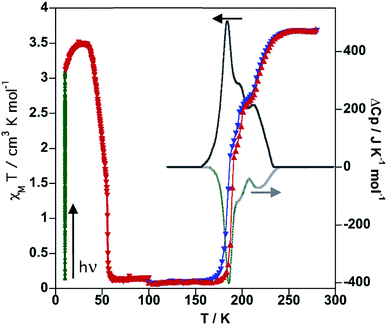 |
| Fig. 3 Magnetic, photomagnetic and calorimetric properties of 1Fe@pyr. Blue down triangles and red up triangles refer to cooling and heating modes, respectively. Green triangles show the increase of χMT upon irradiation at 10 K (LIESST effect), and red down triangles correspond to the heating from 10 K at 0.3 K min−1 to determine the TLIESST temperature. Grey curves correspond to the anomalous variations of the molar specific heat Cp caused by the SCO for the cooling and heating modes. | |
Irradiation of the sample with red light (λ = 633 nm) at 10 K photo-generates the metastable HS* state (light induced excited spin state trapping (LIESST),32 see the green line in Fig. 3).
The subsequent heating at 0.3 K min−1 in the dark shows an increase of χMT, associated with the zero-field splitting of the S = 2 HS state of FeII, reaching a maximum value of 3.50 cm3 K mol−1 in the temperature range 24–34 K, which suggests a quantitative population of the HS* state. Upon further heating the HS* relaxes back in two steps to the LS state with a characteristic TLIESST ≈ 50 K.33
The thermodynamic parameters obtained from differential scanning calorimetry were consistent with the χMT vs. T plot (grey lines in Fig. 3). The average enthalpy and entropy variations ΔH = 10.9 kJ mol−1 and ΔS = 56 J K−1 mol−1 are typical of FeII SCO compounds.
Single crystal UV-Vis spectra.
1Fe@pyr displays a reversible thermo-chromic change between pale-yellow (HS) and red (LS). Consequently, the SCO behaviour was monitored on a single crystal through UV-Vis absorption spectra in the temperature range 300–10 K (cooling and heating modes) with temperature scan rates of 1 and 5 K min−1 while recording the spectra every 1 and 5 min (see Fig. 4a, S3 and S4,† respectively). When cooling from 300 K to 10 K, the band centred at 800 nm associated with the HS state bleaches while a new band attributed to the LS state arises centred at 532 nm when cooling to 10 K. Considering the thickness of the crystal, 50 ± 1 μm, and a concentration of Fe of around 1.6 M, a typical value of ferrous spin crossover systems, an extinction coefficient, ε, equal to 7 and 125 M−1 cm−1 was found, respectively, for the 800 and 532 nm bands. These values are consistent with the d–d transitions 5T2 → 5E and 1A1 → 1T1 characteristic of the HS and LS states of FeII, respectively.
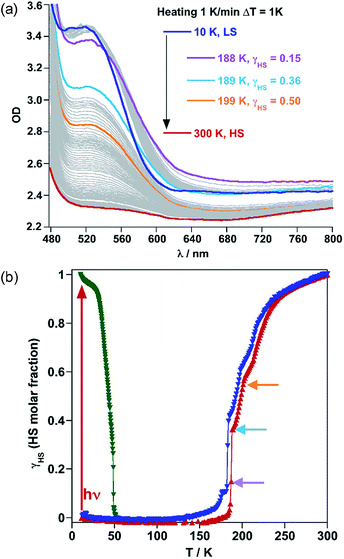 |
| Fig. 4 SCO properties of 1Fe@pyr monitored by using single crystal UV-Vis spectra: (a) temperature variable UV-Vis absorption spectrum in the cooling mode and (b) HS molar fraction of the thermal and light induced SCO. | |
Following the optical density difference between the maximum of the LS band at 532 nm and the tail of this band at 650 nm, the evolution of the HS molar fraction (γHS) with temperature was stablished by using Vergard's law (eqn (1)).
| γHS = (ODLS − ODT)/(ODLS − ODHS), | (1) |
where OD
LS is the optical density of the LS state, OD
HS is the optical density of the HS state at 300 K and OD
T is the optical density at a given temperature. The optical density (OD) is corrected from an eventual baseline jump or shift by taking the difference between the OD at 532 nm and the OD at 650 nm, where there is no noticeable absorption in the two states. The resulting SCO profile displays three main steps centred at 230, 195 and 184 K (
Fig. 4b). Irradiation of the crystal with green light (
λ = 532 nm) at 10 K quantitatively photo-generates the metastable HS* state. The thermal stability of HS* was examined following the spectra when heating the crystal in the dark at 0.3 K min
−1 from 10 to 60 K (
Fig. 4b and S5
†). The thermal relaxation HS* → LS was characterized by a
TLIESST = 45 K. Both, thermal- and photo-induced SCO behaviours agree reasonably well with the complementary magnetic measurements.
Synergy between fluorescence and spin crossover
The crystal structure of 1Fe@pyr reveals that the pyr guest molecules and bpben bridging ligands lie in an environment that is fully consistent to afford exciplex emission through π–π interactions.34 Consistently with this, the excitation spectrum recorded at room temperature at 520 nm, shows a set of four different main bands, two of them highly resolved and centred at 254 and 332 nm. The other two bands are rather broad and centred at around 350 and 400 nm. Likewise, the emission spectrum of the sample has been recorded between 350 and 700 nm after excitation at 332 nm (Fig. S6†). The two characteristic bands of the monomer and excimer of pyrene are centred at 400 and 500 nm, respectively. In order to study the influence of the thermal spin transition on the luminescence properties, the sample was cooled down to 10 K at 5 K min−1 and the luminescence spectra were recorded in the cooling and heating modes (Fig. 5a and S7†). Upon cooling to 190 K from room temperature, the intensity of both peaks, excimer and monomer, slightly increases whereas with further cooling in the region of the spin transition at around 235–155 K the intensity of the monomer signal increases significantly concomitantly with the quenching of the excimer's signal (Fig. 5a). The thermal dependence of fluorescence for the non-SCO isostructural compound 1Zn@pyr after excitation at 332 nm shows two main maxima centred at 439 and 484 nm (Fig. 5b), whose intensity does not change significantly in the 235–155 K interval where 1Fe@pyr displays the SCO behaviour (see Fig. 5c). Clearly the spin transition properties of 1Fe@pyr have a strong influence on the photoluminescence properties. This observation is directly related to the absorption properties of the compound and the emission properties of the pyrene. The arising of the LS absorption band at 532 nm (Fig. 4a) upon cooling quenches the emission of the excimer signal of pyrene at 478 nm (Fig. 5a) due to the radiative energy transfer (emission–reabsorption) from the excited state of the pyrene to the MLCT-3d (1A1 → 1T1) band of the FeII complex in the LS state. The signal at 392 nm, that abruptly increases below the spin transition, overlaps with the MLCT-3d (1A1 → 1T1) transition band of the compound at any temperature. From the ratio between the intensity at 392 nm and 478 nm while heating and cooling at 5 K min−1 the thermal evolution of the normalized HS molar fraction (γHS)norm has been obtained. The thermal spin transition stablished based on the luminescence properties of 1Fe@pyr follows reasonably well the SCO profile obtained by absorption spectroscopy and magnetic measurements (Fig. 6). The differences observed, particularly at low temperatures, can be ascribed, on one hand, to the much more rapid temperature scan rate used for recording the SCO by luminescence and, on the other hand, to the difficulties to separate the increasing signal of the monomer emission from that of the exciplex as temperature decreases. In spite of this, the average equilibrium temperature at which (γHS)norm = 0.5, T1/2 = 189.5 K, centred in the middle of a hysteresis loop ca. 5 K wide, is virtually the same as that observed from magnetism and absorption spectroscopy. In a recent study, likely inspired by the complex [Fe(abpt)2(NCS)2] with abpt = 4-amino-3,5-bis(pyridine-2-yl)-1,2,4-triazole,35 pyrene was covalently coupled by condensation of 1-pyrenecarboxaldehyde with abpt to afford (pyrene-1-yl)-N-(3,5-di(pyridin-2-yl)-4H-1,2,4-triazol-4-yl)methanimine (L).14 The resulting [Fe(L)2(NCS)2] compound undergoes a SCO in the interval 200–350 K with T1/2 = 267 K. The pyrene moieties interact with each other defining four face-to-face π–π interactions (3.66–3.85 Å). The lack of exciplex emission well separated from the intrinsic emission of pyrene moieties precluded from delineating a neat correlation between the thermal SCO and fluorescence profiles in this compound. However, the obvious differences observed in the temperature dependence of fluorescence between [Fe(L)2(NCS)2] and the free ligand L, associated with a resonance energy transfer process similar to that observed for 1Fe@pyr, made possible to infer a clear influence of the SCO on the fluorescence. In particular, the partial population (ca. 21%) of the photo-generated HS* LIESST state of [Fe(L)2(NCS)2] at 10 K also demonstrates this influence on the fluorescence.
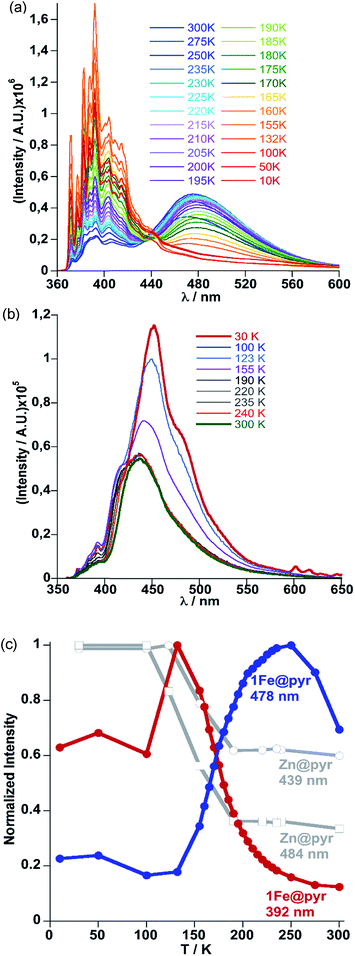 |
| Fig. 5 (a) Evolution of fluorescence spectra with temperature upon cooling from 300 K to 10 K at 5 K min−1 for 1Fe@pyr. (b) Evolution of the luminescence spectra with temperature upon cooling from 300 K to 10 K at 5 K min−1 for 1Zn@pyr. (c) Thermal dependence of normalized intensity of the monomer and excimer signals at 392 nm (red) and 478 nm (blue) for 1Fe@pyr and 439 nm and 484 nm (grey) for 1Zn@pyr. | |
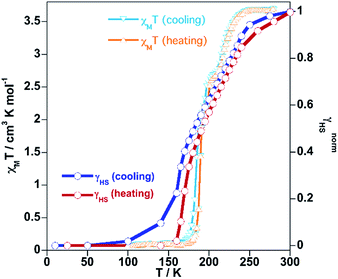 |
| Fig. 6 Comparison of the SCO profiles obtained from magnetism and fluorescence for 1Fe@pyr. | |
Similarly, photo-excitation of the titular compound 1Fe@pyr from LS to HS* (LIESST effect) has been preliminary followed by luminescence. In Fig. 7 the fluorescence spectra at 10 K before and after irradiation of the sample at 520 nm at two different irradiation times are compared. Interestingly, the fluorescence of the monomer decreases concomitantly with an increase of the excimer signal due to the excitation of the LS state into the metastable HS* state. In this sense, further analysis could be performed in order to study the cooperativity of the system thought LIESST and HS to LS relaxations at low temperature by taking advantage of the photoluminescence properties of pyrene.
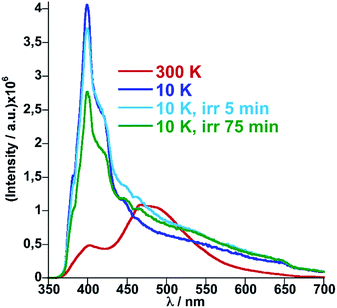 |
| Fig. 7 Fluorescence spectra of 1Fe@pyr at 10 K before and after irradiation at 520 nm. | |
Conclusions
In summary, we have successfully synthesized an unprecedented 3D Hofmann-type FeII SCO-CP functionalized with pyrene as a fluorescent guest. Strong π–π interactions between pyrene and the pillars of the pcu SCO framework result in exciplex emission. Both monomer and exciplex fluorescence are dependent on the spin state of the FeII nodes which in turn is controlled by temperature and light irradiation (LIESST effect), thereby resulting in ON–OFF switching of the luminescent signal. Implementing the prolific family of Hofmann-type SCO-MOFs with luminescence properties offers new opportunities for designing and probing devices at micro- and nano-scales for sensing and information processing. In this sense, incorporation of a luminescent source in the pillars of the framework is a further desired step. Our preliminary results in this direction are very promising and will be reported elsewhere in due course.
Conflicts of interest
There are no conflicts to declare.
Acknowledgements
This work was supported by the Spanish Ministerio de Economia y Competitividad (MINECO), FEDER (CTQ2013-46275-P and CTQ2016-78341-P), Unidad de Excelencia María de Maeztu (MDM-2015-0538), Generalitat Valenciana (PROMETEO/2016/147) and the Swiss National Science Foundation (Project number 200021-169033).
Notes and references
- See for example:
(a)
Spin Crossover in Transition Metal Compounds I-III. Top. Curr. Chem., P. Gütlich and H. A. Goodwin, 2004, vol. 233–235 Search PubMed;
(b) J. A. Real, A. B. Gaspar and M. C. Muñoz, Dalton Trans., 2005, 2062 RSC;
(c) A. Bousseksou, G. Molnár, L. Salmon and W. Nicolazzi, Chem. Soc. Rev., 2011, 40, 3313 RSC;
(d)
Spin-crossover materials: properties and applications, ed. M. A. Halcrow, John Wiley & Sons, 2013 Search PubMed.
-
(a) M. Cavallini, I. Bergenti, S. Milita, J. C. Kengne, D. Gentili, G. Ruani, I. Salitros, V. Meded and M. Ruben, Langmuir, 2011, 27, 4076 CrossRef PubMed;
(b)
P. N. Martinho, C. Rajnak and M. Ruben, in Spin-Crossover Materials: Properties and Applications, ed. M. A. Halcrow, Wiley, 2013, pp. 376–404 Search PubMed;
(c) G. Molnar, S. Rat, L. Salmon, W. Nicolazzi and A. Bousseksou, Adv. Mater., 2018, 30, 17003862 CrossRef PubMed.
-
H. J. Shepherd, C. M. Quintero, G. Molnar, L. Salmon and A. Bousseksou, in Luminescent spin-crossover materials, John Wiley & Sons Ltd., 2013, p. 347 Search PubMed.
- C. Piguet, E. Rivara-Minten, G. Bernardinelli, J.-C. Buenzli and G. Hopfgartner, J. Chem. Soc., Dalton Trans., 1997, 421 RSC.
- M. Matsuda, H. Isozaki and H. Tajima, Thin Solid Films, 2008, 517, 1465 CrossRef.
- L. Salmon, G. Molnár, D. Zitouni, C. Quintero, C. Bergaud, J.-C. Micheau and A. Bousseksou, J. Mater. Chem., 2010, 20, 5499 RSC.
- S. Titos-Padilla, J. M. Herrera, X.-W. Chen, J. J. Delgado and E. Colacio, Angew. Chem., Int. Ed., 2011, 50, 3290 CrossRef PubMed.
- C. M. Quintero, I. A. Gurals’kiy, L. Salmon, G. Molnar, C. Bergaud and A. Bousseksou, J. Mater. Chem., 2012, 22, 3745 RSC.
- I. Suleimanov, O. Kraieva, J. Sánchez-Costa, I. O. Fritsky, G. Molnár, L. Salmon and A. Bousseksou, J. Mater. Chem. C, 2015, 3, 5026 RSC.
- I. Suleimanov, O. Kraieva, G. Molnár, L. Salmon and A. Bousseksou, Chem. Commun., 2015, 51, 15098 RSC.
- M. Hasegawa, F. Renz, T. Hara, Y. Kikuchi, Y. Fukuda, J. Okubo, T. Hoshi and W. Linert, Chem. Phys., 2002, 277, 21 CrossRef.
- H. Matzukizono, K. Kuroiwa and N. Kimikuza, Chem. Lett., 2008, 37, 446 CrossRef.
- A. Santoro, L. J. Kershaw Cook, R. Kulmaczewski, S. A. Barrett, O. Cespedes and M. A. Halcrow, Inorg. Chem., 2015, 54, 682 CrossRef PubMed.
- J.-L. Wang, Q. Liu, Y.-S. Meng, X. Liu, H. Zheng, Q. Shi, C.-Y. Duan and T. Liu, Chem. Sci., 2018, 9, 2892 RSC.
- Y. Garcia, F. Robert, A. D. Naik, G. Zhou, B. Tinant, K. Robeyns, S. Michotte and L. Piraux, J. Am. Chem. Soc., 2011, 133, 15850 CrossRef PubMed.
- B. Schafer, T. Bauer, I. Faus, J. A. Wolny, F. Dahms, O. Fuhr, S. Lebedkin, H.-C. Wille, K. Schlage, K. Chevalier, F. Rupp, R. Diller, V. Schünemann, M. M. Kappes and M. Ruben, Dalton Trans., 2017, 46, 2289 RSC.
- C.-F. Wang, R.-F. Li, X.-Y. Chen, R.-J. Wei, L.-S. Zheng and J. Tao, Angew. Chem., Int. Ed., 2015, 54, 1574 CrossRef PubMed.
- C. Lochenie, K. Schötz, F. Panzer, H. Kurz, B. Maier, F. Puchtler, S. Agarwal, A. Köhler and B. Weber, J. Am. Chem. Soc., 2018, 140, 700 CrossRef PubMed.
- M. C. Muñoz and J. A. Real, Coord. Chem. Rev., 2011, 255, 2068 CrossRef.
- Z.-P. Ni, J.-L. Liu, M. N. Hoque, W. Liu, J.-Y. Li, Y.-C. Chen and M. L. Tong, Coord. Chem. Rev., 2017, 335, 28 CrossRef.
- V. Niel, J. M. Martínez-Agudo, M. C. Muñoz, A. B. Gaspar and J. A. Real, Inorg. Chem., 2001, 40, 3838 CrossRef PubMed.
- A. Galet, A. B. Gaspar, M. C. Muñoz, G. V. Bukin, G. Levchenko and J. A. Real, Adv. Mater., 2005, 17, 2949 CrossRef.
-
(a) N. F. Sciortino, K. R. Scherl-Gruenwald, G. Chastanet, G. J. Halder, K. W. Chapman, J. F. Létard and C. J. Kepert, Angew. Chem., Int. Ed., 2012, 51, 10154 CrossRef PubMed;
(b) M. J. Murphy, K. A. Zenere, F. Ragon, P. D. Southon, C. J. Kepert and S. M. Neville, J. Am. Chem. Soc., 2017, 139, 1330 CrossRef PubMed.
-
(a) M. Ohba, K. Yoneda, G. Agustí, M. C. Muñoz, A. B. Gaspar, J. A. Real, M. Yamasaki, H. Ando, Y. Nakao, S. Sakaki and S. Kitagawa, Angew. Chem., Int. Ed., 2009, 48, 4767 CrossRef PubMed;
(b) G. Agustí, R. Ohtani, K. Yoneda, A. B. Gaspar, M. Ohba, J. F. Sánchez-Royo, M. C. Muñoz, S. Kitagawa and J. A. Real, Angew. Chem., Int. Ed., 2009, 48, 8944 CrossRef PubMed;
(c) P. D. Southon, L. Liu, E. A. Fellows, D. J. Price, G. J. Halder, K. W. Chapman, B. Moubaraki, K. S. Murray, J. F. Létard and C. J. Kepert, J. Am. Chem. Soc., 2009, 131, 10998 CrossRef PubMed;
(d) R. Ohtani, K. Yoneda, S. Furukawa, N. Horike, S. Kitagawa, A. B. Gaspar, M. C. Muñoz, J. A. Real and M. Ohba, J. Am. Chem. Soc., 2011, 133, 8600 CrossRef PubMed.
- J. E. Clements, J. R. Price, S. M. Neville and C. J. Kepert, Angew. Chem., Int. Ed., 2014, 53, 10164 CrossRef PubMed.
- V. Niel, A. L. Thompson, M. C. Muñoz, A. Galet, A. E. Goeta and J. A. Real, Angew. Chem., Int. Ed., 2003, 42, 3760 CrossRef PubMed.
-
(a) V. Meded, A. Bagrets, K. Fink, R. Chandrasekar, M. Ruben, F. Evers, A. Bernand-Mantel, J. S. Seldenthuis, A. Beukman and H. S. J. van der Zant, Phys. Rev. B: Condens. Matter Mater. Phys., 2011, 83, 245415 CrossRef;
(b) F. Prins, M. Monrabal-Capilla, E. A. Osorio, E. Coronado and H. S. J. van der Zant, Adv. Mater., 2011, 23, 1545 CrossRef PubMed;
(c) M. Cavallini, I. Bergenti, S. Milita, J. C. Kengne, D. Gentili, G. Ruani, I. Salitros, V. Meded and M. Ruben, Langmuir, 2011, 27, 4076 CrossRef PubMed;
(d) T. Miyamachi, M. Gruber, V. Davesne, M. Bowen, S. Boukari, L. Joly, F. Scheurer, G. Rogez, T. K. Yamada, P. Ohresser, E. Beaurepaire and W. Wulfhekel, Nat. Commun., 2012, 3, 938 CrossRef PubMed;
(e)
P. N. Martinho, C. Rajnak and M. Ruben, in Spin-Crossover Materials: Properties and Applications, ed. M. A. Halcrow, Wiley, 2013, p. 376 and references therein Search PubMed;
(f) H. J. Shepherd, G. Molnár, W. Nicolazzi, L. Salmon and A. Bousseksou, Eur. J. Inorg. Chem., 2013, 653 CrossRef;
(g) A. Rotaru, J. Dugay, R. P. Tan, I. A. Guralskiy, L. Salmon, P. Demont, J. Carrey, G. Molnár, M. Respaud and A. Bousseksou, Adv. Mater., 2013, 25, 1745 CrossRef PubMed;
(h) I. A. Gural’skiy, C. M. Quintero, J. Sánchez-Costa, P. Demont, G. Molnár, L. Salmon, H. J. Shepherd and A. Bousseksou, J. Mater. Chem. C, 2014, 2, 2949 RSC;
(i) A. C. Aragonés, D. Aravena, J. I. Cerdá, Z. Acís-Castillo, H. Li, J. A. Real, F. Sanz, J. Hihath, E. Ruiz and I. Díez-Pérez, Nano Lett., 2016, 16, 218 CrossRef PubMed.
-
(a) L. Piñeiro-López, M. Seredyuk, M. C. Muñoz and J. A. Real, Chem. Commun., 2014, 50, 1833 RSC;
(b) L. Piñeiro-López, F. J. Valverde-Muñoz, M. Seredyuk, M. C. Muñoz, M. Haukka and J. A. Real, Inorg. Chem., 2017, 56, 7038 CrossRef PubMed.
- J.-Y. Li, C.-T. He, Y.-C. Chen, Z.-M. Zhang, W. Liu, Z.-P. Ni and M. L. Tong, J. Mater. Chem. C, 2015, 3, 7830 RSC.
-
(a) E. Trzop, D. Zhang, L. Piñeiro-Lopez, F. J. Valverde-Muñoz, M. C. Muñoz, L. Palatinus, L. Guerin, H. Cailleau, J. A. Real and E. Collet, Angew. Chem., Int. Ed., 2016, 55, 1 CrossRef;
(b) J. E. Clements, J. R. Price, S. M. Neville and C. J. Kepert, Angew. Chem., Int. Ed., 2016, 55, 15105 CrossRef PubMed;
(c) D. Zhang, E. Trzop, F. J. Valverde-Muñoz, L. Piñeiro-López, M. C. Muñoz, E. Collet and J. A. Real, Cryst. Growth Des., 2017, 17, 2736 CrossRef;
(d) N. F. Sciortino, K. A. Zenere, M. E. Corrigan, G. J. Halder, G. Chastanet, J. F. Létard, C. J. Kepert and S. M. Neville, Chem. Sci., 2017, 8, 70 RSC;
(e) M. J. Murphy, K. A. Zenere, F. Ragon, P. D. Southon, C. J. Kepert and S. M. Neville, J. Am. Chem. Soc., 2017, 139, 1330 CrossRef PubMed.
- N. Ortega-Villar, M. C. Muñoz and J. A. Real, Magnetochemistry, 2016, 2, 16 CrossRef.
- S. Decurtins, P. Gütlich, P. C. Köhler, H. Spiering and A. Hauser, Chem. Phys. Lett., 1984, 105, 1 CrossRef.
- J. F. Létard, P. Guionneau, L. Rabardel, J. A. K. Howard, A. Goeta, D. Chasseau and O. Kahn, Inorg. Chem., 1998, 87, 4432 CrossRef.
- B. D. Wagner, G. J. McManus, B. Moulton and M. Zaworotko, Chem. Commun., 2002, 2176 RSC.
-
(a) N. Moliner, A. B. Gaspar, M. C. Muñoz, S. Létard, J. F. Létard, X. Solans, R. Burriel, M. Castro, O. Kahn and J. A. Real, Inorg. Chim. Acta, 1999, 291, 279 CrossRef;
(b) A. B. Gaspar, M. C. Muñoz, N. Moliner, V. Ksenofontov, G. Levchenko, P. Gütlich and J. A. Real, Monatsh. Chem., 2003, 134, 285 CrossRef.
Footnotes |
† Electronic supplementary information (ESI) available: Experimental information, and synthesis and characterisation of materials (PDF). CCDC 1847351–1847353 & 1862017. For ESI and crystallographic data in CIF or other electronic format see DOI: 10.1039/c8sc02677g |
‡ These authors contributed equally. |
|
This journal is © The Royal Society of Chemistry 2018 |
Click here to see how this site uses Cookies. View our privacy policy here.