DOI:
10.1039/C8AN01472H
(Paper)
Analyst, 2019,
144, 284-289
A sensitive and fast responsive fluorescent probe for imaging hypoxic tumors†
Received
1st August 2018
, Accepted 8th October 2018
First published on 16th October 2018
Abstract
Nitroreductase activities are positively associated with the hypoxic level of tumors, making it an attractive target for tumor detection. Herein, we have developed a 2,5-bis(methylsulfinyl)-1,4-diaminobenzene based probe (BBP), which is a nitroreductase (NTR) responsive fluorescent probe and can rapidly detect NTRs with high sensitivity and specificity. The BBP showed not only a selective response to NTRs over other biological reductants, but also high sensitivity to NTRs and could detect as low as 20 ng mL−1 NTRs. Furthermore, the BBP responded rapidly to NTRs in as fast as 10 minutes, enabling real-time monitoring of the production levels of NTRs. Most importantly, the BBP could identify NTR activities in 2D cell monolayers, 3D tumor spheroids, and even solid tumors in mice. Particularly, the BBP could monitor the early tumor formation and treatment response via measuring NTR activities. Overall, the BBP appears to be an ideal imaging probe for the detection of solid tumors, and possesses great potential in a broad range of diagnostic and therapeutic applications in the clinic.
Introduction
Hypoxia is a characteristic feature of a tumor microenvironment caused by an inadequate oxygen supply,1–3 and is closely associated with tumor initiation, malignant progression, metastasis and therapy resistance.4,5 Therefore, imaging the hypoxic state in tumors is of great importance for the diagnosis and treatment of malignant tumors.6 Under hypoxic conditions, tumor cells show increased endogenous nitroreductase (NTR) activities, making NTRs an attractive target for selectively and efficiently measuring hypoxia in tumor cells or tumor-derived tissue.7,8 Therefore, sensitive and rapid detection of NTR activities could be an ideal strategy for identifying solid tumors.
NTRs are a family of flavin-containing enzymes that catalyze one-electron reduction of nitro groups to an amine in the presence of nicotinamide adenine dinucleotide phosphate (NAD(P)H) as a cofactor.9,10 The NTR-catalyzed reduction eventually changes the physical and chemical properties of NTR substrates, allowing it to interface with imaging techniques, such as NMR,11,12 PET13–16 and fluorescence,17–20 to monitor NTR activities in vitro and in vivo. In comparison with other imaging methods, fluorescence imaging is an ideal tool for tracing the changes and activities of biological targets, thereby greatly facilitating NTR detection.17,21,22 Particularly, NTR-switchable fluorescent probes have received a lot of attention due to their exceptional sensitivity.23–27 However, most of the reported NTR probes lack the ability to achieve rapid and specific assessment of NTR activities, and especially, could not provide the necessary information for monitoring early tumor formation and treatment responses. Therefore, there is a great need for the development of a fluorescent switchable probe for selectively and effectively imaging NTRs in tumor.
Herein, we have developed a novel 2,5-bis(methylsulfinyl)-1,4-diaminobenzene based probe (BBP), which is designed to target the NTR-catalyzed one-electron reduction (shown in Fig. 1). The BBP is composed of a fluorophore 2,5-bis(methylsulfinyl)-1,4-diaminobenzene (BMeSI-p-A),28 conjugated with a 4-nitrobenzylcarbamate moiety. The protection changes the electronic parameters of amines (σp = −0.66) to carbamates (σp = −0.17), resulting in fairly weak fluorescence emission. However, the NTR-catalyzed reduction of 4-nitrobenzylcarbamate induces the cleavage of the carbamate linkage, and subsequently causes the release of free BMeSI-p-A, resulting in strong fluorescence emission (Fig. 1). This unique fluorescence “turn-on” feature greatly reduces the background interference and improves the detection sensitivity. The BBP also shows high specificity for NTRs over other reductases and biological reducing agents. Most importantly, the BBP can not only identify the hypoxic state in 2D cell monolayers and 3D tumor spheroids based on NTR expression profiles, but also monitor the early tumor formation and the treatment response in vivo, indicating its great potential for improving the treatment of tumors.
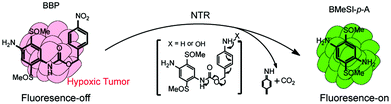 |
| Fig. 1 BBP possesses a unique fluorescence off–on feature, and can rapidly identify NTRs in solid tumors with high sensitivity and specificity. | |
Experimental section
Materials and instrument
All chemicals and solvents were purchased from Sigma and Aladdin Reagent Company without further purification. All solvents are of reagent grade unless otherwise indicated. Nitroreductases (NTRs) from Escherichia coli and NADH were purchased from Sigma Reagent Company. NTRs were dissolved in nanopure water and frozen immediately at −20 °C for storage. Human hepatocarcinoma HepG-2 cells and human hepatic L02 cells were obtained from the Chinese Academy of Sciences (Shanghai, China). Nude ICR mice were purchased from the Experimental Animal Center of Nanjing Medical University. All reactions were performed under an inert atmosphere of nitrogen (N2). 1H NMR spectra were recorded on a Varian Inova 500 (at 500 MHz and 126 MHz, respectively). NMR data were reported relative to internal CDCl3 (1H, δ = 7.26) and CDCl3 (13C, δ = 77.0). Data for 1H NMR spectra were reported as follows: chemical shift (δ ppm) (multiplicity, coupling constant (Hz), and integration). Multiplicity and qualifier abbreviations were as follows: s = singlet, d = doublet, t = triplet, q = quartet, m = multiplet and br = broad. HRMS were acquired from the NJU Mass Spectral Facility using electrospray ionization (ESI-TOF). A microplate reader (Tecan, 200 pro, USA) was used for measuring absorption and emission spectra. Confocal laser scanning microscopy (CLSM) (Zeiss, L710, Germany) was used for tumor imaging. All animal experiments were performed in accordance with the National Institute of Health Guidelines following the protocols, approved by the ethics committee at the Affiliated Drum Tower Hospital of Nanjing University Medical School.
Synthesis of the BBP
To a mixture of BMeSI-p-A (0.93 g, 4.0 mmol), triethylamine (5.1 mL, 4.0 g, 40.0 mmol), and N,N-dimethylpyridin-4-amine (1.1 g, 8.8 mmol) in DMF (10 mL) was added a solution of 4-nitrobenzyl carbonochloridate (1.0 g, 4.8 mmol) in DCM (1 mL). The reaction mixture was stirred for 6 hours at room temperature under an inert atmosphere of nitrogen (N2). The reaction was quenched by adding ice water (100 mL), and the mixture was extracted with CH2Cl2 (3 × 100 mL). The organic phase was combined, washed with water (10 mL) and brine (10 mL), and dried with anhydrous Na2SO4. The solvent was evaporated under vacuum, and the residue was purified by flash chromatography on silica (CH2Cl2/CH3OH, 6/1 → 4/1) to afford the BBP as a light yellow powder (0.99 g, 60.3%). 1H NMR (500 MHz, DMSO-d6, 35 °C): δ = 9.01 (s, 1H; NH), δ = 8.23 (d, 2H; nitrobenzyl), 7.48 (t, 4H; phenyl), 6.04 (s, 2H; NH2), 5.26 (s, 2H; CH2), 2.73 (s, 3H; CH3), 2.51 (s, 3H; CH3); HRMS (ESI): m/z calcd for C16H17N3O8S2 [M+]: 411.4539; found: 412.0657.
Fluorescence quantum yield measurements
Fluorescence quantum yield measurements were performed on a PerkinElmer LS 55 instrument, and quinine sulfate in 0.22 M NaOH was chosen as a fluorescence standard. The fluorescence quantum yield (Φfl) was obtained with the following equation (F denotes the fluorescence intensity at each wavelength and [F] was calculated by summation of the fluorescence intensity).
Φsamplefl = ΦstandardflAbsstandard[Fsample]/Abssample[Fstandard] |
Detection of NTRs
All spectroscopic measurements were performed in PBS (10 mM, pH 7.4), and in accordance with the following procedure. Different concentrations of the BBP in PBS (100 μL) were added into a 96-well plate (flat black and transparent bottom), followed by the addition of NADH (final concentration, 100 μM) and an appropriate volume of NTRs. The reaction solution was incubated at 37 °C, and the fluorescence intensity (Ex/Em = 375/520 nm) was measured using the microplate reader (Tecan, 200 pro, USA). Meanwhile, a mixed solution of BBP and NADH in PBS without NTRs was prepared as the control.
Identification of NTR levels in hypoxic tumor cells
HepG-2 and L02 cells were incubated with 4 μM of BBP in FBS-free 1640 medium at 37 °C for 30 minutes, and washed three times with PBS to remove the extracellular BBP. After this, the cells were further incubated in a humidified incubator under hypoxic conditions for 4 hours. An Anaero Pack-Anaero (Mitsubishi Gas Chemical Co. Inc., Japan) was used to provide hypoxic cell culture environments with 1% oxygen concentration. For the control group, HepG-2 and L02 cells were incubated under normoxic conditions (95% air and 5% CO2). Fluorescence was measured by CLSM (Zeiss, L710, Germany).
Detection of hypoxic conditions in three-dimensional (3D) tumor spheroids
3D tumor spheroids of HepG-2 cells were developed by a liquid overlay method.29 The tumor spheroids were allowed to grow up to the diameter of about 300–400 μm for 7 days at 37 °C in the presence of 5% CO2. The uniform and compact tumor spheroids were selected, and incubated with 4 μM of the BBP for 30 minutes. The tumor spheroids were washed three times with PBS, and the fluorescence images of the tumor spheroids were obtained by CLSM.
Identification of hypoxic levels in tumors
Nude ICR mice (6–8 weeks, 18–22 g) were purchased from the Experimental Animal Center of Nanjing Medical University. To set up the tumor xenograft models, the ICR mice were subcutaneously inoculated with 1 × 107 HepG-2 cells. When the average tumor volumes reached 500 mm3, the BBP was injected into the tumor tissues. After one hour post-injection of the BBP, tumors were collected, and sliced at different layers from the top to the middle by cryotomy. The tumor sections were then imaged by CLSM.
Results and discussion
Design and synthesis of the BBP
An ideal fluorescent probe for NTRs should possess some outstanding features, such as high sensitivity and specificity, short response time, and optimized features for minimizing the auto-fluorescence of biological matter. Here, we designed a novel NTR responsive probe, named BBP, which is composed of fluorescent moiety BMeSI-p-A conjugated with an NTR-responding group via a cleavable linkage (Fig. 1). Fluorescent dye BMeSI-p-A and the nitro-benzylcarbamate moiety were chosen as the fluorescence-reporting unit and NTR-responsive unit, respectively. BMeSI-p-A has some unique properties. BMeSI-p-A is a hydrophilic fluorescent dye, and therefore, it greatly improves the water solubility and cell permeability of the BBP. In addition, BMeSI-p-A is a green fluorescent dye with a high fluorescence quantum yield, whereas it shows a very weak fluorescence signal after conjugating with 4-nitrobenzylcarbamate. However, NTR-catalyzed reduction of the BBP can trigger the release of free BMeSI-p-A, enabling it to be visualized easily. This fluorescence “turn-on” feature of the BBP greatly enhances its detection limit. The synthetic methodology for the BBP is outlined in Fig. S1.†
Absorption and emission spectra of the BBP
BMeSI-p-A has a typical push–pull electronic structure, endowing it with high fluorescence quantum yield (Φ = 18.21%, Table S1†). In contrast, the BBP showed a very weak fluorescence intensity (Φ = 0.09%, Table S1†), because the nitro group induced an electron-transfer process and quenched the fluorescence emission.30 The BBP showed high reactivity towards NTRs due to its strong intermolecular interactions such as hydrogen bonding, π–π stacking interactions and a hydrophobic effect. However, the NTR-catalyzed reduction of the BBP induced the release of free BMeSI-p-A, resulting in strong fluorescence (Fig. 1). Therefore, the BBP possessed a unique NTR-initiated fluorescence “turn-on” feature.
The photophysical analyses of the BBP were systemically performed. Fig. 2a shows that the main absorption peaks of the BBP and BMeSI-p-A appeared at 348 nm and 375 nm, respectively. However, the absorption of the BBP shifted and overlapped with BMeSI-p-A after reacting with NTRs, indicating that BMeSI-p-A was successfully regenerated from the BBP. In addition, NTR-catalyzed release of BMeSI-p-A was further confirmed by LC-MS analysis, and 80% of BMeSI-p-A was recovered from the BBP within 10 minutes (Fig. S2† and Fig. 2b). Furthermore, the BBP showed a very weak fluorescence intensity under excitation at 375 nm, which was at least two orders of magnitude lower than that of BMeSI-p-A, approving our hypothesis of the fluorescence quenching effect of the 4-nitrobenzyl moiety on BMeSI-p-A. However, the BBP showed a dramatic increase in the fluorescence intensity (more than 200-fold) after it reacted with NTRs in the presence of NADH (Fig. 2b), demonstrating that it has a unique “turn-on” feature associated with NTRs.
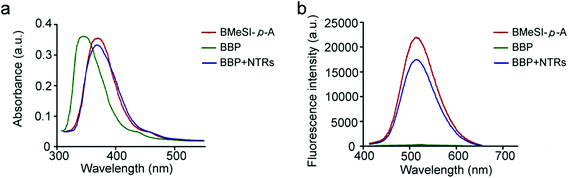 |
| Fig. 2 Spectroscopic measurements of the BBP. (a) UV-vis absorption spectra of BMeSI-p-A (5 μM), BBP (5 μM) and the reaction mixture of BBP (5 μM), NTRs (5 μg mL−1) and NADH (100 μM). (b) The fluorescence spectra of BMeSI-p-A (5 μM), BBP (5 μM), and the reaction mixture of BBP (5 μM), NTRs (5 μg mL−1) and NADH (100 μM). All measurements (Ex/Em = 375/520 nm) were performed at 37 °C in 10 mM PBS. | |
Optimal conditions for the detection of NTRs
To achieve sensitive detection of NTRs, the conditions for the BBP to detect NTRs were optimized. The stability experiment was first performed, and the BBP appeared to be quite stable in the serum for a period of at least one week at 37 °C (Fig. S3†). Furthermore, the effects of pH and temperature on the stability of the BBP in the physiological environment were investigated. Changing the pH from 6 to 8 and the temperature from 35 to 40 °C had no significant effects on the absorbance and emission of the BBP (Fig. S4 and S5†), suggesting that the BBP has good stability. Furthermore, the response time of the NTR-catalyzed reduction of the BBP was systemically investigated, and time-dependent signal changes were collected every 1 minute within 30 minutes. As shown in Fig. 3a, the BBP in the absence of NTRs (control) showed very low fluorescence intensity over long periods of time. In contrast, a progressive increase in the fluorescence intensity was immediately detected within 1 minute, and the strongest fluorescence was reached within 10 minutes, indicating that the BBP shows a fast response to NTRs. Based on the above studies, the detection of NTRs was optimized to physiological conditions at 37 °C in PBS buffer (pH = 7.4, 10 mM) for 10 minutes.
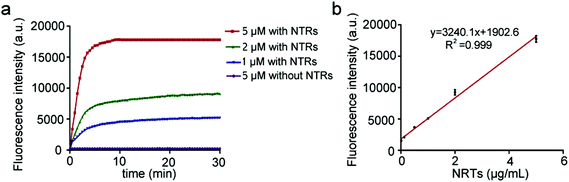 |
| Fig. 3 Ability of the BBP to detect NTRs. (a) The fluorescence spectra of the mixture of BBP (5 μM) reacting with different concentrations of NTRs in the presence of NADH (100 μM). (b) A linear correlation between the fluorescence intensity and different concentrations of NTRs. All measurements (Ex/Em = 375/520 nm) were performed at 37 °C in 10 mM PBS. | |
In vitro detection of NTRs
To fully profile the abilities of the BBP to detect NTRs, their sensitivity, specificity and kinetics were systemically investigated. A series of biological species in living systems including salts (NaCl and CaCl2), small biomolecules (Cys), bio-macromolecules (BSA) and enzymes (Trypsin) were utilized to test the specificity of the BBP. Fig. S6† shows that there was no significant fluorescence enhancement observed from a mixed solution of BBP, NADH and biological species, demonstrating that the BBP was inert to biological interference in living systems. In addition, dicoumarin, an inhibitor of NTRs, significantly inhibited the response of the BBP to NTRs, which demonstrates that the fluorescence off–on response of the BBP was associated with NTR activities.31 These results confirm that the BBP shows high specificity for NTRs, and selectively targets the NTR-reduction mechanism.
The ability of NTRs to reduce the BBP was further evaluated. Fig. S7† demonstrates that the BBP showed different responses to high concentrations of NTRs, which could greatly shorten the time span, and increase the plateau of the fluorescence intensity. In addition, the BBP exhibited a remarkable sensitivity to NTRs, and could detect as low as 20 ng mL−1 NTRs. More importantly, a linear regression of the fluorescence plateau against different concentrations of NTRs was drawn with a coefficient of determination (R2 = 0.999) (Fig. 3b), indicating that the BBP could be utilized to quantify the levels of NTRs in vitro and in vivo. The kinetics of the NTR-catalyzed reduction of the BBP was also investigated. Under the optimized conditions, a Lineweaver–Burk double-reciprocal plot of the reciprocal of rate (V, the initial reaction rate) versus the reciprocal of the concentration of the BBP (C) was established (Fig. S8†). The calculated values of the apparent Michaelis–Menten constant Km and maximum rate Vmax were 1.37 min−1 and 0.03 μmol (L min)−1, respectively, indicating that the BBP is a sensitive probe for NTRs. All these unique properties allow the BBP to rapidly detect a wide range of NTR-related diseases.
Evaluation of the cytotoxicity of the BBP
To develop novel specific imaging probes, biocompatibility should also be taken into consideration, which eventually determines the scope of applications. The cytotoxicity of the BBP to HepG-2 and L02 cells was tested using the standard MTT assay. The cells were incubated with the BBP at 37 °C under normoxic and hypoxic conditions (20% and 1% O2, respectively). Fig. S9† shows that no significant cytotoxic effects were observed with the BBP even at high concentrations (up to 500 μM), suggesting that the BBP has good biocompatibility and is suitable for in vivo applications.
The capacity of the BBP for the intracellular NTR imaging
We further investigated if the BBP could distinguish hypoxic tumor cells from other normal cells in vitro. Fig. 4a shows that both HepG-2 and L02 exhibited negligible fluorescence intensities under the normoxic conditions. However, when the cells were incubated under the hypoxic conditions,32,33 HepG-2 showed a great increase in the fluorescence intensity compared to L02, indicating that the BBP can discriminate hypoxic tumor cells from healthy cells based on the NTR expression levels.
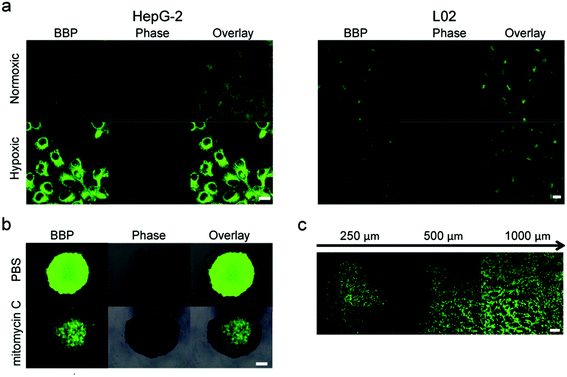 |
| Fig. 4 The imaging of NTRs in hypoxic tumors with the BBP. (a) Fluorescence imaging of cells under different oxygen concentrations. The HepG-2 and L02 cells were treated with a 4 μM hypoxic probe BBP for 30 minutes, and cultured under normoxic (20% pO2) or hypoxic (1% pO2) conditions for one hour, followed by imaging by CLSM. Scale bar indicates 10 μm. (b) Fluorescence imaging of 3D HepG-2 tumor spheroids pretreated with PBS or mitomycin C. The scale bar indicates 50 μm. (c) In vivo imaging of HepG-2 tumor-bearing mice. Mice were intratumorally injected with the BBP (2 mg kg−1), and tumors were collected one hour later. The frozen tumor sections were observed at different depths by CLSM. The scale bar indicates 100 μm. | |
In comparison with 2D culture of tumor cells, three-dimensional tumor spheroids closely mimic the heterogeneity and microenvironment of tumors. In addition, 3D-cultured cells also have different gene and protein expression profiles compared to 2D-cultured cells, leading to different behaviors in cellular processes including growth and proliferation, migration and invasion. Particularly, 3D tumor spheroids can generate a hypoxic microenvironment, which eventually stimulates the overexpression of NTR-like intracellular reductases.34 We therefore investigated if the BBP could image the hypoxic state in 3D tumor spheroids. Tumor spheroids of HepG-2 cells were treated with the BBP under a high hypoxic status for 30 minutes, and the fluorescence intensity was imaged with CLSM. As shown in Fig. 4b, the whole spheroids showed strong green fluorescence, indicating that the BBP exhibits good tumor penetration and can detect the hypoxic levels of whole tumors. In addition, the spheroid center showed a higher fluorescence intensity than the spheroid periphery, indicating that the tumor cells in spheroids have different hypoxic states and display different NTR expression profiles. Since hypoxia plays a key role in tumor initiation, progression, angiogenesis and migration, the ability of the BBP to detect the expression patterns of NTRs provides us a powerful tool for studying the mechanisms of tumor development.
We also performed additional experiments to identify if the BBP could evaluate the therapeutic efficacy of anticancer agents targeting hypoxia. HepG-2 spheroids were treated with mitomycin C (1 μM), a clinically used bioreductive drug.35,36 After 24 hours, the hypoxic state of HepG-2 spheroids was imaged with the BBP. Fig. 4b shows that mitomycin C pretreated spheroids showed very low fluorescence, compared to the control. These results suggest that the fluorescence signals generated from the BBP were associated with the hypoxic state of tumor cells, and therefore, the BBP could evaluate the therapeutic effects of anticancer drugs.
In vivo imaging of NTRs in hypoxic tumors with the BBP
Finally, we performed the experiments to investigate the ability of the BBP to detect NTRs in HepG-2 tumor-bearing mice. A solution of the BBP in PBS was directly injected into tumor tissues. One hour after injection, tumors were collected and sliced at different layers from the top to the middle by cryotomy, followed by imaging with CLSM. Fig. 4d shows that a strong fluorescence signal was observed in whole tumor tissues, and no fluorescence signal was found in the adjacent healthy tissues. In addition, the strong fluorescence intensity was observed at the depth of 250 μm, and fluorescence was even detected at the depth of 1000 μm below the tumor surface. Therefore, the BBP can effectively image the hypoxic state in vivo, and monitor tumor formation and development.
Conclusions
In summary, we have developed a nitroreductase responsive fluorescent probe BBP for selectively identifying the hypoxic state in solid tumors. The BBP appeared to be an ideal imaging probe due to its numerous advantages, such as rapid responses, good sensitivity, high specificity, practical pharmacokinetics, and last but not least, low cytotoxicity. The BBP showed excellent specificity to NTRs, and had a rapid response time toward NTRs, allowing the procurement of the tumor growth information. Particularly, the BBP could distinguish hypoxic tumor cells from other normal cells based on NTR production profiles. With all the advantageous features, the BBP could identify the early tumor formation and treatment response. Therefore, there is no doubt that the BBP will play a key role in the battle against malignant tumors.
Conflicts of interest
There are no conflicts to declare.
Acknowledgements
This work was supported by the Thousand Talents Program for Young Researchers, the National Natural Science Foundation of China (Grant No. 21472090), the National Key Research and Development Program of China (No. 2018YFB1105400), and Fundamental Research Funds for Central Universities.
References
- A. L. Harris, Nat. Rev. Cancer, 2002, 2, 38 CrossRef CAS PubMed.
- F. He, X. Deng, B. Wen, Y. Liu, X. Sun, L. Xing, A. Minami, Y. Huang, Q. Chen and P. B. Zanzonico, Cancer Res., 2008, 68, 8597–8606 CrossRef CAS PubMed.
- W. R. Wilson and M. P. Hay, Nat. Rev. Cancer, 2011, 11, 393 CrossRef CAS PubMed.
- E. Shinohara and A. Maity, Curr. Mol. Med., 2009, 9, 1034–1045 CrossRef CAS PubMed.
- K. J. Williams, M. R. Albertella, B. Fitzpatrick, P. M. Loadman, S. D. Shnyder, E. C. Chinje, B. A. Telfer, C. R. Dunk, P. A. Harris and I. J. Stratford, Mol. Cancer Ther., 2009, 8, 3266–3275 CrossRef CAS PubMed.
- N. K. Tafreshi, M. C. Lloyd, J. B. Proemsey, M. M. Bui, J. Kim, R. J. Gillies and D. L. Morse, Mol. Imaging. Biol., 2016, 18, 219–231 CrossRef CAS PubMed.
- W. R. Wilson and M. P. Hay, Nat. Rev. Cancer, 2011, 11, 393 CrossRef CAS PubMed.
- N. K. Tafreshi, M. C. Lloyd, J. B. Proemsey, M. M. Bui, J. Kim, R. J. Gillies and D. L. Morse, Mol. Imaging. Biol., 2016, 18, 219–231 CrossRef CAS PubMed.
- Z. C. Symons and N. C. Bruce, Nat. Prod. Rep., 2006, 23, 845–850 RSC.
- H. H. Nguyen-Tran, G. W. Zheng, X. H. Qian and J. H. Xu, Chem. Commun., 2014, 50, 2861 RSC.
- K. Matsuo, R. Kamada, K. Mizusawa, H. Imai, Y. Takayama, M. Narazaki, T. Matsuda, Y. Takaoka and I. Hamachi, Chemistry, 2014, 19, 12875–12883 CrossRef PubMed.
- K. Tanabe, H. Harada, M. Narazaki, K. Tanaka, K. Inafuku, H. Komatsu, T. Ito, H. Yamada, Y. Chujo and T. Matsuda, J. Am. Chem. Soc., 2009, 131, 15982–15983 CrossRef CAS PubMed.
- J. N. Copp, A. M. Mowday, E. M. Williams, C. P. Guise, A. Ashoorzadeh, A. V. Sharrock, J. U. Flanagan, J. B. Smaill, A. V. Patterson and D. F. Ackerley, Cell Chem. Biol., 2017, 24, 391–403 CrossRef CAS PubMed.
- G. Smith, L. Carroll and E. O. Aboagye, Mol. Imaging Biol., 2012, 14, 653–666 CrossRef PubMed.
- X. Ning, W. Seo, S. Lee, K. Takemiya, M. Rafi, X. Feng, D. Weiss, X. Wang, L. Williams and V. M. Camp, Angew. Chem., Int. Ed., 2014, 53, 14096–14101 CrossRef CAS PubMed.
- R. A. Bissell, A. P. d. Silva, H. Q. N. Gunaratne, P. L. M. Lynch, G. E. M. Maguire, C. P. McCoy and K. R. A. S. Sandanayake, Analyst, 2009, 134, 2385–2393 RSC.
- Y. Li, Y. Sun, J. Li, Q. Su, W. Yuan, Y. Dai, C. Han, Q. Wang, W. Feng and F. Li, J. Am. Chem. Soc., 2015, 137, 6407–6416 CrossRef CAS PubMed.
- Z. Li, X. He, Z. Wang, R. Yang, W. Shi and H. Ma, Biosens. Bioelectron., 2015, 63, 112–116 CrossRef CAS PubMed.
- J. E. De, J. J. Keating, S. A. Kularatne, J. Jiang, R. Judy, J. Predina, S. Nie, P. Low and S. Singhal, Int. J. Mol. Imaging, 2015, 2015, 469047 Search PubMed.
- X. Ning, S. Lee, Z. Wang, D. Kim, B. Stubblefield, E. Gilbert and N. Murthy, Nat. Mater., 2011, 10, 602–607 CrossRef CAS PubMed.
- L. Cui, Y. Zhong, W. Zhu, Y. Xu, Q. Du, X. Wang, X. Qian and Y. Xiao, Org. Lett., 2011, 13, 928 CrossRef CAS PubMed.
- M. Stanton, M. Cronin, P. Lehouritis and M. Tangney, Curr. Gene Ther., 2015, 15, 277–288 CrossRef CAS PubMed.
- J. Xu, S. Sun, Q. Li, Y. Yue, Y. Li and S. Shao, Analyst, 2015, 140, 574–581 RSC.
- J. Yuan, Y. Q. Xu, N. N. Zhou, R. Wang, X. H. Qian and Y. F. Xu, RSC Adv., 2014, 4, 56207–56210 RSC.
- J. Bae, L. E. Mcnamara, M. A. Nael, F. Mahdi, R. J. Doerksen, N. I. Hammer and S. Jo, Chem. Commun., 2015, 51, 12787–12790 RSC.
- D. Yang, H. Y. Tian, T. N. Zang, M. Li, Y. Zhou and J. F. Zhang, Sci. Rep., 2017, 7, 9174 CrossRef PubMed.
- H.-C. Huang, K.-L. Wang, S.-T. Huang, H.-Y. Lin and C.-M. Lin, Biosens. Bioelectron., 2011, 26, 3511–3516 CrossRef CAS PubMed.
- T. Beppu, K. Tomiguchi, A. Masuhara, Y. J. Pu and H. Katagiri, Angew. Chem., Int. Ed., 2015, 54, 7332–7335 CrossRef CAS PubMed.
- C. Ju, R. Mo, J. Xue, L. Zhang, Z. Zhao, L. Xue, Q. Ping and C. Zhang, Angew. Chem., Int. Ed., 2014, 53, 6253–6258 CrossRef CAS PubMed.
- A. Harriman, L. J. Mallon, G. Ulrich and R. Ziessel, ChemPhysChem, 2007, 8, 1207–1214 CrossRef CAS PubMed.
- E. Johansson, G. N. Parkinson, W. A. Denny and S. Neidle, J. Med. Chem., 2003, 46, 4009–4020 CrossRef CAS PubMed.
- G. Arteel, R. Thurman, J. Yates and J. Raleigh, Br. J. Cancer, 1995, 72, 889 CrossRef CAS PubMed.
- P. F. Searle, M. J. Chen, L. Hu, P. R. Race, A. L. Lovering, J. I. Grove, C. Guise, M. Jaberipour, N. D. James and V. Mautner, Clin. Exp. Pharmacol. Physiol., 2004, 31, 811–816 CrossRef CAS PubMed.
- L.-B. Weiswald, D. Bellet and V. Dangles-Marie, Neoplasia, 2015, 17, 1–15 CrossRef PubMed.
- O. Sirenko, T. Mitlo, J. Hesley, S. Luke, W. Owens and E. F. Cromwell, Assay Drug Dev. Technol., 2015, 13, 402–414 CrossRef CAS PubMed.
- F. M. de Groot, E. W. Damen and H. W. Scheeren, Curr. Med. Chem., 2001, 8, 1093–1122 CrossRef CAS PubMed.
Footnote |
† Electronic supplementary information (ESI) available. See DOI: 10.1039/c8an01472h |
|
This journal is © The Royal Society of Chemistry 2019 |
Click here to see how this site uses Cookies. View our privacy policy here.