DOI:
10.1039/C8BM01228H
(Paper)
Biomater. Sci., 2019,
7, 287-295
Polymer–antibiotic conjugates as antibacterial additives in dental resins†
Received
3rd October 2018
, Accepted 9th November 2018
First published on 12th November 2018
Abstract
Affecting the vast majority of human beings, dental caries is a premier concern of worldwide dental health. As the most commonly used restorative material to treat dental caries, resin-based composites (RBCs) lack antibacterial properties leading to quite limited restoration lifetimes. The objective of this study is to develop a polymer–antibiotic conjugate (PAC) as an effective antibacterial additive for RBCs. A monomer–antibiotic conjugate (MAC) with significant solubility was prepared by an esterification reaction of tert-butyloxycarbonyl (Boc)-protected ciprofloxacin (Cip) and 2-hydroxyethyl methacrylate (HEMA). The Cip-containing PAC with well-controlled molecular weight and composition was synthesized by reversible addition–fragmentation chain transfer (RAFT) copolymerization of the MAC with HEMA (1
:
3 molar ratio), followed by the removal of Boc from the resulting copolymer. The antibacterial dental resin was then prepared by incorporating the PAC into a commercial resin, and their properties and antibacterial performance against Streptococcus mutans were tested. In vitro experiments revealed a very slow release of Cip, which resulted in significant killing effectiveness against Streptococcus mutans nonetheless, as observed through zone of inhibition assessment and SEM imaging. The promising antibacterial properties of these resins indicate that incorporating a PAC as an additive is a valid strategy to generate antibacterial materials for dental applications.
1. Introduction
As the most common infectious disease worldwide, dental caries has remained a major oral health concern. In 2015, approximately 2.5 billion people were affected by untreated caries which gave an age-standardized prevalence of 34.1% all across the world.1 As the most commonly used restorative material over the past few decades, resin-based composites have shown serious shortcomings, particularly in terms of the restoration lifetime.2 Most of these failed restorations are caused by secondary caries or recurrent caries.3,4 The failure of resin-based composite restorations is typically the result of two major aspects: the degradation of the restorative composites caused by endogenous proteases and the bacterial invasion at the interface between dentin and the restorative composite.5,6 Research has shown that resin composites tend to accumulate more dental biofilms over time, which in turn enhances their degradation.7 Because of the lack of inhibition against cariogenic bacteria, such as Streptococcus mutans (S. mutans), recurrent caries evolves due to the secondary infection around the restoration margins even after the removal of the infected dentin and adequate restorative sealing.8,9
Over the past few decades, researchers have studied the incorporation of antibacterial agents within the composite resin materials to achieve effective inhibition against cariogenic bacterial growth.10 In general, composite materials consist of two major components: the resin matrix and the filler particles. The antimicrobial properties could be incorporated through either the resin matrix11 or the filler particles.12 In both cases, agents with antibacterial properties can be classified into two categories: released soluble agents and non-released stationary agents.
As the essential component providing strength, wear resistance, reduced shrinkage and shade stability to composite materials, glass fillers may constitute 70% to 90% of the total weight of specific dental composite formulations. Various strategies combining different antibacterial agents with the filler particles have been reported. Released antimicrobial agents such as fluoride,13,14 silver ions,12 and zinc oxide15 have been incorporated into the mixture in an attempt to achieve significant antimicrobial performance. However, as these agents are released, the generation of voids can negatively affect the mechanical properties of the composites. Burst release is another concern of this technique. Conversely, non-released agents, such as silver-supported fillers16 and 12-methacryloyloxydodecylpyridinium bromide (MDPB)-incorporated fillers17 are known to maintain remarkable mechanical properties after aging since the antimicrobial component is not released over time. However, the bacterial inhibition of these systems typically is not as good as those of the released agents, thus requiring a substantially higher weight percentage of the agent to achieve similar bacterial inhibition. Therefore, because a balance between the overall properties and antibacterial performance of the resulting material must be maintained, developing filler particles that exhibit great antimicrobial performance without compromising other material properties has been a persistent challenge. For instance, poor color stability has been an issue commonly reported for inorganic fillers containing metal ions.18
Modifications to the resin matrix have also been extensively investigated. Released soluble antibacterial agents, such as organic fluoride,19,20 chlorhexidine,21 and quaternary-ammonium salts (QAS),22 have been added into the resin matrix. Although these agents demonstrate considerable inhibition of bacterial growth around restorative composites, this approach has the main shortcoming of burst release, regardless of the agent, limiting their antibacterial effect to only the initial stage when they are first released. Furthermore, this strategy is not capable of incorporating significant amounts of highly effective antibiotics with poor solubility. As a topic with rising popularity in recent years, incorporation of non-releasing insoluble agents, especially macromolecular QAS, could overcome some of the disadvantages of the aforementioned approaches restricted by burst release, mechanical strength loss, polymeric shrinkage and insufficient bacterial inhibition. These polycations inhibit the growth of bacterial cells naturally as contact disinfectants by disturbing the negatively charged cell membrane.23–27 Nonetheless, there are still some limitations of this type of antimicrobial agent. It is difficult for these immobilized compounds to extend their antibacterial effects beyond the tooth–composite interface. Furthermore, with the occurrence of surface adsorption of protein, the surface inhibition effects further decrease.
Because all the aforementioned approaches have limitations that are intrinsically difficult to overcome, the development of new antibiotic restorative composites by incorporating novel antibacterial additives emerges as a highly attractive research direction. As an important type of drug delivery system, polymer–drug conjugates (PDCs)28–30 have been studied broadly, and various types of polymer–drug combinations have been developed, mainly for the treatment of different types of cancer. Compared with systemic circulation-based therapies directly using small molecule drugs, PDCs exhibit multiple advantages.30,31 PDCs not only provide protective environments for the drugs in biological systems, but they also lead to an increased effective blood circulation time, sustained drug release, and reduced side effects that may be derived from premature drug release. Moreover, relative to small molecule drugs, PDCs typically have higher solubility and can also be readily modified to enhance their biomedical functions.32,33 The polymer–drug conjugation linkage should be essentially stable in normal healthy biological environments, but vulnerable under disease-specific conditions (such as acidic pH, high enzymatic concentrations, etc.) so as to promote drug release when and where necessary.
Based on the PDC concept with the use of antibiotics as specific therapeutic agents, polymer–antibiotic conjugates (PACs) have also been studied in recent years.34–42 For instance, Convertine and co-workers synthesized well-defined PACs containing ciprofloxacin (Cip; an antibiotic) moieties and poly(ethylene glycol) (PEG) side chains; these PACs can form water-dispersible nanoparticles in aqueous solutions, with tunable Cip release kinetics mediated by conjugation linkage chemistry.34,35 In principle, PACs inherit some of the general advantages of PDCs, and may have important potential applications for the treatment and prevention of various diseases caused by bacterial infections.
To the best of our knowledge, incorporation of PACs into dental resins to create antimicrobial dental restorative materials has not been reported to date. This strategy offers great promise as it represents an alternative approach to overcoming the inherent limitations associated with each of the aforementioned strategies. Specifically, as antibacterial additives, PACs exhibit a number of advantages including the following: (1) with the help of a selected polymer backbone with enhanced solubility in commercial resins, potent antibiotics with poor solubility could be conjugated, and the resulting PACs would still have considerable solubility; (2) because the polymer backbone of PACs may be synthesized by using monomers that have been utilized in commercial dental resins, incorporation of PACs is not likely to cause significant changes in the mechanical, physical and optical properties of the resultant antibacterial resins; (3) benefiting from the polymeric protection, slow and steady release of antibiotics can be expected to prolong the life of dental restorations while greatly suppressing unwanted adverse side effects; (4) the overall dosage of antibiotics can be controlled through the PAC amount, and the release kinetics can be adjusted through the stability of conjugation linkages; (5) the released antibiotics can be further spread in the local environment around the restoration site as opposed to only inhibiting bacterial growth at the composite–dentin interface; (6) unlike metal-containing agents, these metal free PACs are not expected to affect the color stability of the restorative resins; (7) the multifunctional polymers introduced by the PACs also allow more opportunities for considering additional modifications to target other desired properties of these restorative systems; (8) as additives, PACs are in principle compatible with other antibacterial strategies, and may interact synergistically; and (9) the PAC approach can be used by incorporation into both dental adhesives and restorative composites thus maximizing the delivery routes and, consequently, exposure.
Here we report our recent study of novel composite resins incorporating Cip-containing PACs as antibacterial agents (Scheme 1). Cip was selected in this study since it is a very commonly used broad spectrum antibiotic effective against a wide range of Gram positive and negative bacteria, while also providing multiple functional groups for modification.34,35 Selected as the optimal design of a monomer–antibiotic conjugate (MAC) based on remarkable solubility, HEMA-Cip-Boc (HCB) was synthesized through esterification between Boc-protected Cip (CB) and 2-hydroxyethyl methacrylate (HEMA). The final PAC, p(HC-co-HEMA), was obtained by reversible addition–fragmentation chain transfer (RAFT) copolymerization of MAC and HEMA, followed by the removal of the Boc group from the resulting copolymer. In vitro antibiotic release from the PAC was investigated. Using the PAC as an antibacterial additive, cured antibacterial resin discs were obtained and used for evaluation of their antibacterial performance against S. mutans by zone of inhibition (ZOI) tests and SEM imaging. The overall results suggest that the incorporation of the PAC into resin-based restorative materials is a promising strategy for the development of dental resins with sustained antibacterial properties.
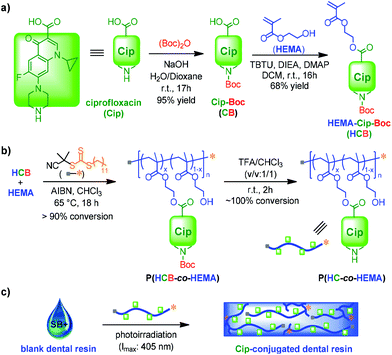 |
| Scheme 1 Synthesis of (a) a MAC (HCB, via Boc protection of Cip and esterification); (b) a PAC (p(HC-co-HEMA), via RAFT polymerization and Boc deprotection); and (c) PAC-incorporated dental resin by photocuring. | |
2. Results and discussion
2.1. Studies of MACs
Based on the molecular structure of Cip with carboxylic acid and secondary amine functionalities, three designs of MACs were evaluated (Fig. 1). A methacryloyl (MA)-based monomer unit was incorporated with Cip in each of the designs, because methacrylates are commonly used in dental resin formulations. The synthesis of HEMA–Cip (HC) was attempted at first by direct esterification of Cip with HEMA using various catalytic systems (Scheme S1†). However, due to the very low solubility of Cip in commonly used inert organic solvents, these direct esterification attempts were not successful. The modification of the secondary amino group of Cip using Boc was then explored. According to the literature,43 the addition of a Boc group to the amino group site not only provides protection by preventing amidation between the amino and carboxylic groups on Cip, but also breaks the tightly packed crystal structure of Cip and further enhances the solubility of the resulting product, Cip–Boc, in organic solvents such as dichloromethane (DCM) and chloroform (CHCl3). Following approaches reported in the literature,34,43 Cip–Boc was prepared with 95% yield by the reaction of Cip with (Boc)2O in a dioxane/water mixed solvent in the presence of sodium hydroxide at room temperature for 17 h; then the esterification of the Boc-modified Cip (Cip–Boc) with HEMA was performed in DCM catalyzed by O-(benzotriazol-1-yl)-N,N,N′,N′-tetramethyluronium tetrafluoro-borate (TBTU), N,N-diisopropylethylamine (DIEA) and 4-(dimethylamino)pyridine (DMAP), to give HEMA–Cip–Boc (HCB) in 68% yield (Scheme 1a). Subsequently, HEMA–Cip (HC) was readily obtained in 82% yield through the removal of the Boc group by treating HCB in a mixed solvent of DCM and trifluoroacetic acid (TFA; v/v = 1/1) for 2 h at room temperature (Scheme S2†). In addition, Cip–MA (CM), the MAC of Cip with the amine site modified with the MA group, was obtained in 50% yield by the amidation reaction of Cip with MA chloride in DCM in the presence of triethylamine at room temperature overnight (Scheme S3 and Fig. S1†).
 |
| Fig. 1 Structural designs of MACs, including HC, HCB and CM. | |
Although each of the MACs possesses a monomer group, the likelihood that these can be incorporated, directly or indirectly (i.e. via PACs), within a dental resin depends on their solubility. Therefore, the solubility of these MACs was first investigated (Table 1). Neither of the MACs could be dissolved in 3M™ Adper™ Single Bond Plus adhesive (SB+), a commonly used commercial dental resin adhesive formulation. Their solubility in a variety of other commonly used solvents, including H2O, EtOH, pyridine, toluene, benzene, DCM, and chloroform, was also evaluated. Unfortunately, HC is insoluble and CM has only limited solubility (up to ∼10 mg mL−1) in the tested solvents, indicating that they may not be readily converted to PACs via solution polymerization. Interestingly, despite the poor solubility of HCB in most of the tested solvents, it showed high solubility (more than 200 mg mL−1) in both DCM and CHCl3. Thus, HCB can be used as a MAC for the preparation of PACs via solution polymerization when either DCM or CHCl3 is used as the solvent.
Table 1 Solubility of MACs at room temperaturea
MAC |
Solvent |
SB+ |
H2O |
EtOH |
Py |
MePh |
Bz |
DCM |
CHCl3 |
SB+: 3M™ Adper™ Single Bond Plus adhesive; Py: pyridine; Bz: benzene; NS: not soluble, i.e. no noticeable dissolution in the solvent; SS: slightly soluble (solubility < 5 mg mL−1).
|
HC |
NS |
NS |
NS |
SS |
NS |
NS |
NS |
NS |
HCB |
NS |
NS |
NS |
SS |
NS |
NS |
>200 mg mL−1 |
>200 mg mL−1 |
CM |
NS |
NS |
NS |
NS |
NS |
NS |
∼10 mg mL−1 |
∼10 mg mL−1 |
2.2. Synthesis of PACs
Because of the limited solubility of MACs in resin formulations, PACs with significant solubility in dental resin formulations were further prepared for use as antibacterial additives. Such PACs were synthesized via RAFT polymerization of MACs with HEMA. The RAFT approach was selected because it provides excellent structural control of polymers in a metal-free process under mild conditions.44 Moreover, the resulting well-defined PACs would have RAFT terminal groups capable of participating in the subsequent free radical polymerization reaction of the PAC-containing dental adhesives.45 HEMA was selected as the comonomer because it is a very commonly used monomer in dental resin formulations. A molar ratio of MAC to HEMA of 1
:
3 was designed to achieve both significant antibiotic wt% and good solubility/dispersity of the resulting PACs in HEMA-containing SB+. Because a high degree of polymerization (DP) unfavorably affects PAC solubility and leads to high viscosity of PAC-containing adhesive formulations, but a low DP causes difficulties in the recovery of PACs from polymerization systems, a moderate DP of 40 for the PACs was targeted.
RAFT copolymerization of HCB with HEMA was performed using 2-cyano-2-propyl dodecyl trithiocarbonate (CPDT) as the RAFT agent, and 2,2′-azobis(2-methylpropionitrile) (AIBN) as the initiator in chloroform at 65 °C for 18 h ([HCB]0
:
[HEMA]0
:
[CPDT]0
:
[AIBN]0 = 10
:
30
:
1
:
0.2; Scheme 1b). 1H NMR analysis in CDCl3 for the final polymerization mixture showed almost no signal for vinyl protons, indicating nearly 100% conversion of vinyl groups during the copolymerization. As a result, the copolymer composition can be expected to be very similar to the feed ratio of comonomers. Further 1H NMR analysis of the recovered polymer, p(HCB-co-HEMA), confirmed the molar ratio of 1
:
3 for HCB and HEMA units in the resulting polymer, as derived from the intensity of resonance of two aromatic protons of HCB at 7.6–8.6 ppm relative to that of other protons (Fig. 2). According to gel permeation chromatography (GPC) analysis, p(HCB-co-HEMA) has a number-average MW (Mn) of 11.5 kDa and a MW dispersity (Đ) of 1.53, relative to linear polystyrenes (Fig. 3). This experimental Mn value is in relatively close agreement with the calculated Mn of 9.6 kDa (corresponding to a total DP of 40), suggesting good MW control. The Đ value of the PAC is somewhat higher than the typical Đ range for polymers prepared by the RAFT process, presumably due to the steric effect of bulky HCB on polymerization. For the application of a PAC as an antimicrobial additive in dental resins in this research, the slightly high Đ value is not a concern. Thereafter, this Boc-protected PAC, in short P-PAC, is expressed as p(HCB0.25-co-HEMA0.75)40.
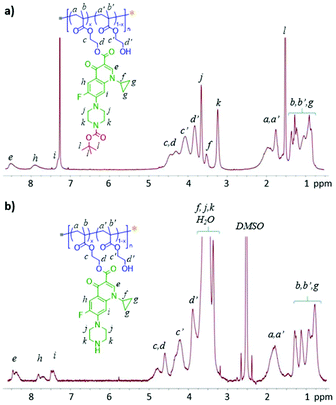 |
| Fig. 2 500 mHz 1H NMR spectra of PACs: (a) p(HCB0.25-co-HEMA0.75)40 in CDCl3 and (b) p(HC0.25-co-HEMA0.75)40 in DMSO-d6. The 1H NMR results verified the presence of conjugated Cip moieties in the PACs. | |
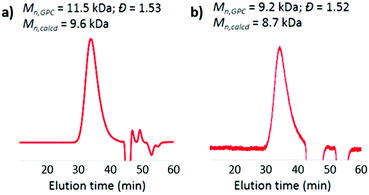 |
| Fig. 3 DMF GPC curves of PACs: (a) p(HCB0.25-co-HEMA0.75)40 and (b) p(HC0.25-co-HEMA0.75)40. The GPC results verified the well-controlled synthesis of PACs. | |
The removal of the Boc group from p(HCB0.25-co-HEMA0.75)40 was readily achieved by treating it with DCM/TFA (v/v = 1/1) for 2 h. The resulting unprotected PAC p(HC0.25-co-HEMA0.75)40, in short UP-PAC, was obtained in quantitative yield. It has limited solubility in CHCl3, but remains soluble in DMF and DMSO. 1H NMR analysis in DMSO-d6 confirmed the complete removal of Boc groups by the essential absence of its characteristic resonances at 1.5 ppm (Fig. 2b). Furthermore, the 1
:
3 molar ratio of HC and HEMA units in the polymer was confirmed, as derived from the intensity of resonance of three aromatic protons of HCB at 7.3–8.6 ppm relative to that of other protons. GPC analysis revealed that p(HCB0.25-co-HEMA0.75)40 has a Mn of 9.2 kDa and a Đ of 1.52, relative to linear polystyrenes (Fig. 3b). Its experimental Mn is in close agreement with the calculated Mn of 8.7 kDa. Its Đ is also almost the same as the Đ of the precursor (i.e., P-PAC). According to the consistency in terms of Mn and Đ before and after the deprotection step, we conclude that side reactions during deprotection were absent or negligible.
2.3. Preparation and characterization of PAC-incorporated resins
The PACs with well-controlled Mn values and compositions were used for the preparation of PAC-incorporated resins, using SB+ as the base resin formulation (Scheme 1c). The liquid formulations of mixed PACs with SB+, with 1.84 wt% of Cip, were prepared first. After ultrasonication, the mixture of P-PAC and SB+ formed a transparent viscous solution, while the mixture of UP-PAC and SB+ resulted in a slightly opaque solution, presumably due to the somewhat decreased solubility of UP-PAC relative to P-PAC in SB+. Resin discs were obtained by curing the P-PAC-incorporated SB+ and UP-PAC-incorporated SB+, as well as SB+ alone as a control. During the curing process, monomers/crosslinkers in SB+ were polymerized via a radical mechanism; in the case with PAC addition, the PACs with a RAFT-functionality at ω-terminals served as macro chain-transfer agents, and therefore, were covalently linked with the SB+ matrix during curing.46 The degree of conversion (DC) of vinyl groups is an important parameter in determining the final physical, mechanical and biological properties of photo-activated composite resins. Similar to what is observed with conventional dental resins, a high DC of the resins was confirmed in both cases by FT-IR analysis of the discs based on the significantly reduced vinyl absorption at 1620–1640 cm−1 after photocuring.47,48 The PAC-incorporated resin discs exhibited an opaque white color similar to that of the cured blank SB+ resin, and no color change was observed for either of these discs during the storage time. In addition, no significant change of the surface hydrophilicity of PAC-incorporated resin discs relative to the blank discs was observed (Fig. S2†).
2.4. Antibiotic release study
Because the release mechanism of the Cip moieties from these PACs, both P-PAC and UP-PAC, is through hydrolysis of the ester bond between Cip and HEMA units, the release rate of the Cip moiety would be expected to be somewhat similar between the two PACs. Because of the much higher solubility of Cip–Boc relative to Cip, P-PAC with releasable Cip–Boc moieties was used as the representative sample in the release study via an incubation-based approach. A thin film of P-PAC (with a thickness of 6 μm) was prepared by solution casting, and then incubated in pH 7.4 PBS buffer at 37 °C. After a minor burst release of ∼2% of Cip–Boc within day 1, a slow and sustained release of Cip–Boc from day 1 to day 25 was observed with approximately 5.12% total release measured at day 25, the longest time period studied to date (Fig. 4). The initial burst release might correspond to the diffusion of free Cip–Boc formed during the preparation of the P-PAC film, and the subsequent slow release should be ascribed to the gradual cleavage of the ester conjugation linkages of P-PAC. Such slow release of the antibiotic is desirable as it would ensure that the PAC may remain effective for long periods of time after being incorporated within the dental resins. Although the release rate of PAC-incorporated SB+ resin discs could not be quantified by the same method because some of the components in the SB+ gave signals at 275 nm (which overlapped with the characteristic peak of the antibiotic), an even slower release rate of the antibiotic from the PAC-incorporated resins is expected due to the high diffusion resistance from the resin matrix. Although an in vivo antibiotic release study has not been performed to date, somewhat faster antibiotic release under in vivo conditions may be predicted because the presence of biological enzymes and differential pH ranges in the oral cavity could catalyze the hydrolysis of ester bonds.
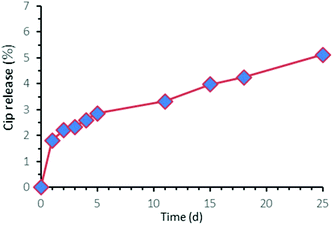 |
| Fig. 4 Cip release (in a Boc-protected form, i.e. Cip–Boc) from the P-PAC film incubated in pH 7.4 PBS buffer at 37 °C, as monitored by UV-Vis analysis. Slow and sustained Cip release was observed after day 1. | |
2.5. Zone of inhibition
Six groups of samples were used in the zone of inhibition assessment via the disk diffusion method.41,49,50 According to the order of images in Fig. 5, the sample groups are (a) DMSO solution of P-PAC in paper discs, (b) DMSO solution of UP-PAC in paper discs, (c) DMSO in paper discs as the control, (d) P-PAC-incorporated SB+ resin discs, (e) UP-PAC-incorporated SB+ resin discs, and (f) blank SB+ resin discs as the control. Each paper disc was loaded with 20 μL of DMSO solution or pure DMSO. Each resin disc had a mass of 50 mg. There was 1.84 wt% of conjugated Cip in each of the PAC-containing DMSO solutions (groups a–b) or resin discs (groups d–e). Note that positive controls with the same concentration of free Cip could not be used due to the poor solubility of free Cip (<1 mg mL−1 in DMSO), but significant antimicrobial activity of free Cip against S. mutans has been demonstrated by us (Fig. S3†). After incubation of the six groups of samples with S. mutans on brain heart infusion (BHI) agar plates at 37 °C for 24 h, their antibacterial performance against S. mutans was visualized by assessing the formation, or not, of a halo corresponding to a clear zone of bacterial inhibition. Among all of the PAC-containing groups (a–b and d–e), small but visible inhibition zones were only observed around the paper discs loaded with the DMSO solution of UP-PAC (Fig. 5b), indicating that the Cip released from the UP-PAC solution reached an antimicrobially effective concentration due to the antimicrobial efficacy of unprotected Cip. No noticeable zone of inhibition could be observed for the paper discs loaded with the DMSO solution of P-PAC (Fig. 5a), presumably due to the suppressed antibacterial potency of Cip–Boc relative to unprotected Cip. The absence of visible zones of inhibition for UP-PAC-incorporated resin discs (Fig. 5e) can be ascribed to the diffusion resistance from the resin matrix, which may have made the amount of Cip released from the discs too small to inhibit bacterial growth noticeably beyond the disc surface. The UP-PAC-incorporated resin might still be antimicrobial, and more significant inhibition should be expected on the disc surface, which corresponds to the surface of restorative composites in direct contact with the tooth substrates for in vivo dental filling applications.
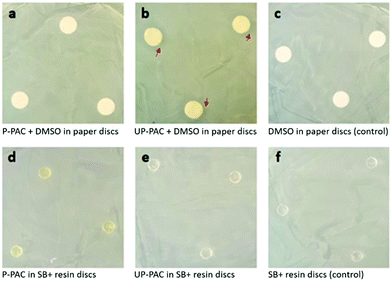 |
| Fig. 5 Images of the zone of inhibition assessment against S. mutans. Only UP-PAC + DMSO in paper discs (image b) resulted in visible inhibition zones, as indicated by red arrows. | |
2.6. Surface inhibition of resin discs by SEM imaging
In order to verify whether the UP-PAC-incorporated resin discs were antimicrobial, additional discs of UP-PAC-incorporated resin and SB+ control were prepared and incubated with S. mutans in broth for 18 h. SEM imaging was then used to evaluate the inhibition of bacterial growth on the cured resin disc surfaces. While well-defined, regular-shaped bacteria were observed on the blank SB+ disc surface (Fig. 6a), damaged bacteria with irregular morphologies were observed on the UP-PAC-incorporated resin disc (Fig. 6b). Such results indicate that S. mutans were effectively killed by the released Cip from the resin matrix.41,51–54 Combined with the slow Cip release kinetics, a slow and sustained Cip release system with sufficient antimicrobial effectiveness against S. mutans can be deduced.
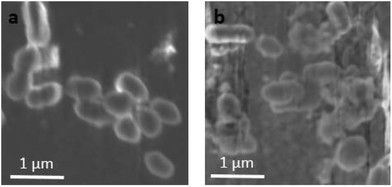 |
| Fig. 6 SEM images of S. mutans cultured on (a) an SB+ disc (control; showing well-defined, regular-shaped bacteria), and (b) UP-PAC-incorporated resin disc (showing damaged bacteria with irregular morphologies). | |
3. Experimental
3.1. Chemical characterization
All 1H NMR spectra were recorded at 500 MHz on a Varian INOVA-500 spectrometer maintained at 25 °C. FT-IR spectra were recorded on a Bruker VERTEX 70 FT-IR spectrometer. UV-vis spectra were recorded using a Shimadzu UV-2600 UV-VIS spectrophotometer.
3.2. Materials
Cip (>98%, HPLC grade), HEMA (98%), di-tert-butyl dicarbonate (Boc2O, >99%), DMAP (99%, prilled), azobisisobutyronitrile (AIBN, 98%), CPDT (97%, HPLC grade), TBTU (95%), DIEA (99%), and TFA (99%) were purchased from Sigma-Aldrich. Sodium hydroxide (pellets, certified ACS), dioxane (HPLC grade), chloroform (HPLC grade) and DCM (HPLC grade) were purchased from Fisher Scientific. 3M™ Adper™ Single Bond Plus adhesive (SB+) was purchased from 3M ESPE. CB and HCB (i.e. MAC) were prepared following literature methods, respectively.34,43
3.3. Synthesis of p(HCB0.25-co-HEMA0.75)40
In a typical reaction, HCB (200 mg, 0.37 mmol.), HEMA (144 mg, 1.10 mmol; the trace amount of the inhibitor was removed by passing through an alumina column), CPDT (12.7 mg, 0.037 mmol) and AIBN (1.2 mg, 0.007 mmol) were dissolved in CHCl3 and added into a reaction vial. After purging with N2 for 60 min, the vial was resealed and placed in a preheated oil bath at 65 °C for 18 h under stirring. The polymerization was quenched by plunging the vial into an ice bath and the system was then opened to air. An aliquot was taken for NMR and GPC analyses to check the conversion before work-up. The rest of the mixture was dissolved in CHCl3, and then precipitated in cold diethyl ether three times. The crude polymer was collected and dried overnight under vacuum at room temperature, to obtain 350 mg p(HCB0.25-co-HEMA0.75)40, i.e. P-PAC, in nearly quantitative yield. Its 1H NMR spectrum is shown in Fig. 2a.
3.4. Synthesis of p(HC0.25-co-HEMA0.75)40
In a typical reaction, p(HCB0.25-co-HEMA0.75)40 (100 mg) was dissolved in 2 mL DCM/TFA (v/v = 1/1) under N2 protection. After the reaction for 2 h, low boiling point components of the reaction mixture were removed under reduced pressure, and the resulting mixture was precipitated in cold diethyl ether three times to obtain the crude polymer with some remaining TFA. After further removal of TFA by an azeotropic distillation with toluene, p(HC0.25-co-HEMA0.75)40, i.e. UP-PAC, was obtained quantitatively (91 mg, yield = 100%). Its 1H NMR spectrum is shown in Fig. 2b.
3.5. Preparation of PAC-incorporated dental resin discs
The PAC-incorporated resins were prepared by adding 12.7 mg P-PAC or 11.34 mg UP-PAC into 233 mg SB+, followed by 1 h of ultrasonication. Then 50 μL of the resulting mixture was taken and transferred to a mold (5 × 1 mm) for the curing process under irradiation with LED light with a λmax of 405 nm for 30 s to form the resin disc.
3.6.
In vitro Cip release from a p(HCB-co-HEMA) film
A thin film (with a thickness of 6 μm) of p(HCB0.25-co-HEMA0.75)40, i.e. P-PAC, was prepared by solution casting on a flat Petri dish using CHCl3 as the solvent followed by gentle evaporation under N2 protection. The PAC film-coated Petri dish was then incubated in 100 mL of pH 7.4 PBS buffer at 37 °C. Aliquots were taken out periodically for release analysis based on the absorbance of released Cip–Boc at 275 nm, and they were then poured back for further incubation. A standard calibration curve to correlate the Cip–Boc concentration and absorbance at 275 nm was generated by measuring the absorbance at 275 nm of a series of Cip–Boc solutions in pH 7.4 PBS buffer with various predetermined concentrations.
3.7. Zone of inhibition and bacterial growth assays
Experimental groups were set as follows: (1) a solution of 12.7 mg P-PAC in 200 μL DMSO; (2) a solution of 11.34 mg UP-PAC in 200 μL DMSO; (3) pure DMSO as a negative control; (4) a P-PAC-incorporated resin disc (concentration: 12.7 mg P-PAC per 200 mg SB+); (5) a UP-PAC-incorporated resin disc (concentration: 11.34 mg UP-PAC per 200 mg SB+); and (6) a blank SB+ disc only as a negative control. Triplicate measurements were performed in each group.
Considering the greater diffusion resistance of the overall drug release process in the PAC-incorporated discs relative to the PAC solutions, the amounts of effective Cip moieties in the PAC-added discs were about 2.5 times those in the solution cases. For different PACs in the same media, the molar concentrations of the Cip moieties are the same. For groups 1–3, in each case, 20 μL of the corresponding solution was loaded on a 5 mm × 1 mm paper disc followed with drying. For groups 4–6, in each case, 50 mg of PAC-incorporated SB+ resins were used to prepare a polymerized disc according to the method specified in section 2.6.
S. mutans strain ATCC 25175 was grown on BHI agar plates (Sigma) overnight anaerobically at 37 °C followed by subculture into liquid BHI broth for assay. For groups 1–3, after sterilizing and drying of the paper discs, 5 μL of the corresponding DMSO solution was added on each paper disc followed by drying in a fume hood; then the dry paper discs were placed on BHI agar plates that were spread with mid-log S. mutans culture (100 μl of OD 0.3) to cover the surface. For groups 4–6, resin discs were placed in the plates after being sterilized for 48 h. All 6 plates, each with 3 discs of the same group, were then incubated anaerobically for 24 h. Subsequently, the plates were removed from the incubator, and the inhibition zones were recorded.
For liquid growth assays, S. mutans grown overnight in BHI was diluted 1
:
100 into 200 μL of BHI containing varying concentrations of Cip in a 96 well plate and incubated anaerobically at 37 °C. The optical density (at λ = 600 nm) was measured every 2 h and after 24 h to monitor bacterial growth.
3.8. SEM imaging of incubated resin disc surfaces
Experimental groups were set as follows: (1) UP-PAC-incorporated resin formulation (concentration: 11.34 mg UP-PAC per 200 mg SB+); and (2) adhesive (SB+) only as a negative control. Multiple samples were prepared for each group. After the photo-curing, the two sets of discs were sterilized using EtOH and allowed to dry for 48 h. Each disc was submerged in 1 mL S. mutans broth culture (OD 0.3) for 18 h anaerobically in a 24 well plate. The discs were then removed from the wells and washed 3 times with sterile PBS buffer, and fixed in 2.5% glutaraldehyde for 4 h, followed by dehydration in a graded series of aqueous EtOH solutions (30%, 50%, 75%, 85%, 95%, and 100%), 10 min in each. The discs were removed from the dehydration bath, further coated in hexamethyldisilizane (HMDS) and allowed to completely dry in a vacuum drier overnight followed by SEM imaging the next day. SEM images were obtained using a Carl Zeiss AURIGA CrossBeam Focused Ion Beam Electron Microscope (FIB-SEM).
4. Conclusions
A novel approach for developing antimicrobial resin-based dental restorative materials was demonstrated by incorporating a PAC into a commercially available dental resin matrix. MACs with distinct molecular structures were synthesized. With significant solubility, HCB was selected as the representative MAC for PAC synthesis by RAFT copolymerization. Well-defined PACs with well-controlled molecular weights were obtained as p(HCB0.25-co-HEMA0.75)40 and p(HC0.25-co-HEMA0.75)40. Antimicrobial PAC-containing dental resins were then successfully prepared by adding p(HC0.25-co-HEMA0.75)40 into the commercial resin, SB+. This new PAC-containing resin further exhibited promising performance, with potential for long-term inhibition of S. mutans growth. The desirable properties of the resulting PAC-incorporated resin material included slow release of Cip for promoting long-lasting antibacterial effects, and surface bacteria killing indicating potent antimicrobial effectiveness even at low concentrations. Overall, the reported PAC-incorporation strategy for the development of antibacterial dental resins shows great potential to overcome some of the major shortcomings of currently available restorative antimicrobial materials, such as burst release with a consequent short effective time, and insufficient antibiotic potency. While the antibacterial performance of the PAC-incorporated dental resins was revealed qualitatively in this work, quantitative assessment of their antibacterial efficacy (zone of inhibition and growth studies) will be pursued in our future studies.
Conflicts of interest
There are no conflicts to declare.
Acknowledgements
This research was supported by the Innovative Micro-Programs Accelerating Collaboration in Themes (IMPACT) program of the University at Buffalo. R. Zhang was partly supported by the National Science Foundation (DMR-1609914). N. Hao was supported by the Shandong Province Higher Educational Science and Technology Program (Grant No. J18KB056).
References
- N. Kassebaum, A. Smith, E. Bernabé, T. Fleming, A. Reynolds, T. Vos, C. Murray, W. Marcenes and GBD 2015 Oral Health Collaborators, J. Dent. Res., 2017, 96, 380–387 CrossRef CAS PubMed.
- US Department of Health and Human Services, 2014, NIH Publication no. 00-4713.
- R. L. Sakaguchi, Dent. Mater., 2005, 21, 3–6 CrossRef PubMed.
- P. E. Murray, L. J. Windsor, T. W. Smyth, A. A. Hafez and C. F. Cox, Crit. Rev. Oral Biol. Med., 2002, 13, 509–520 CrossRef.
- D. H. Pashley, F. R. Tay, C. Yiu, M. Hashimoto, L. Breschi, R. M. Carvalho and S. Ito, J. Dent. Res., 2004, 83, 216–221 CrossRef CAS PubMed.
- C. Sabatini and D. H. Pashley, Am. J. Dent., 2014, 27, 203–214 Search PubMed.
- N. Beyth, I. Yudovin-Fearber, A. J. Domb and E. I. Weiss, Quintessence Int., 2010, 41, 827–835 Search PubMed.
- L. Bjorndal and T. Larsen, Caries Res., 2000, 34, 502–508 CrossRef CAS PubMed.
- K. L. Weerheijm, C. M. Kreulen, J. J. de Soet, H. J. Groen and W. E. van Amerongen, Caries Res., 1999, 33, 130–134 CrossRef CAS PubMed.
- N. Beyth, S. Farah, A. J. Domb and E. I. Weiss, React. Funct. Polym., 2014, 75, 81–88 CrossRef CAS.
- S. Imazato and J. McCabe, J. Dent. Res., 1994, 73, 1641–1645 CrossRef CAS PubMed.
- K. Yamamoto, S. Ohashi, M. Aono, T. Kokubo, I. Yamada and J. Yamauchi, Dent. Mater., 1996, 12, 227–229 CrossRef CAS PubMed.
- X. Xu and J. O. Burgess, Biomaterials, 2003, 24, 2451–2461 CrossRef CAS PubMed.
- A. Yap, E. Khor and S. Foo, Oper. Dent., 1999, 24, 297–305 CAS.
- C. G. Spencer, P. M. Campbell, P. H. Buschang, J. Cai and A. L. Honeyman, Angle Orthod., 2009, 79, 317–322 CrossRef PubMed.
- K. Yoshida, M. Tanagawa and M. Atsuta, J. Biomed. Mater. Res., 1999, 47, 516–522 CrossRef CAS PubMed.
- S. Imazato, N. Ebi, Y. Takahashi, T. Kaneko, S. Ebisu and R. R. Russell, Biomaterials, 2003, 24, 3605–3609 CrossRef CAS PubMed.
- S. Imazato, Dent. Mater., 2003, 19, 449–457 CrossRef CAS PubMed.
- J. Hicks, F. Garcia-Godoy, K. Donly and C. Flaitz, J. Calif. Dent. Assoc., 2003, 31, 229–246 Search PubMed.
- A. Wiegand, W. Buchalla and T. Attin, Dent. Mater., 2007, 23, 343–362 CrossRef CAS PubMed.
- K. Anusavice, N.-Z. Zhang and C. Shen, J. Dent. Res., 2006, 85, 950–954 CrossRef CAS PubMed.
- V. Sehgal, V. S. Shetty, S. Mogra, G. Bhat, M. Eipe, S. Jacob and L. Prabu, Am. J. Orthod. Dentofacial Orthop., 2007, 131, 525–529 CrossRef PubMed.
- S. Imazato, A. Ehara, M. Torii and S. Ebisu, J. Dent. Res., 1998, 26, 267–271 CrossRef CAS.
- T. Thome, M. P. Mayer, S. Imazato, V. R. Geraldo-Martins and M. M. Marques, J. Dent., 2009, 37, 705–711 CrossRef CAS PubMed.
- J. C. Tiller, C.-J. Liao, K. Lewis and A. M. Klibanov, Proc. Natl. Acad. Sci. U. S. A., 2001, 98, 5981–5985 CrossRef CAS PubMed.
- J. C. Tiller, S. B. Lee, K. Lewis and A. M. Klibanov, Biotechnol. Bioeng., 2002, 79, 465–471 CrossRef CAS PubMed.
- H. Li, H. Bao, K. X. Bok, C.-Y. Lee, B. Li, M. T. Zin and L. Kang, Biomater. Sci., 2016, 4, 299–309 RSC.
- R. Tong and J. Cheng, J. Macromol. Sci., Polym. Rev., 2007, 47, 345–381 CAS.
- J. Khandare and T. Minko, Prog. Polym. Sci., 2006, 31, 359–397 CrossRef CAS.
- R. Duncan, Nat. Rev. Cancer, 2006, 6, 688–701 CrossRef CAS PubMed.
- C. Zhu, L. Liu, Q. Yang, F. Lv and S. Wang, Chem. Rev., 2012, 112, 4687–4735 CrossRef CAS PubMed.
- Q. Yin, L. Tang, K. Cai, X. Yang, L. Yin, Y. Zhang, L. W. Dobrucki, W. G. Helferich, T. M. Fan and J. Cheng, Biomater. Sci., 2018, 6, 1189–1200 RSC.
- Y. Ou, K. Chen, H. Cai, H. Zhang, Q. Gong, J. Wang, W. Chen and K. Luo, Biomater. Sci., 2018, 6, 1177–1188 RSC.
- D. Das, S. Srinivasan, A. M. Kelly, D. Y. Chiu, B. K. Daugherty, D. M. Ratner, P. S. Stayton and A. J. Convertine, Polym. Chem., 2016, 7, 826–837 RSC.
- D. Das, J. Chen, S. Srinivasan, A. M. Kelly, B. Lee, H.-N. Son, F. Radella, T. E. West, D. M. Ratner, A. J. Convertine, S. J. Skerrett and P. S. Stayton, Mol. Pharmaceutics, 2017, 14, 1988–1997 CrossRef CAS PubMed.
- H. Yang and S. T. Lopina, J. Biomater. Sci., Polym. Ed., 2003, 14, 1043–1056 CrossRef CAS PubMed.
- R. Satchi-Fainaro, H. Hailu, J. W. Davies, C. Summerford and R. Duncan, Bioconjugate Chem., 2003, 14, 797–804 CrossRef CAS PubMed.
- H. Namazi and A. Kanani, Carbohydr. Polym., 2009, 76, 46–50 CrossRef CAS.
- M. Schmidt, L. K. Bast, F. Lanfer, L. Richter, E. Hennes, R. Seymen, C. Krumm and J. C. Tiller, Bioconjugate Chem., 2017, 28, 2440–2451 CrossRef CAS PubMed.
- B. Cao, F. Xiao, D. Xing and X. Hu, Small, 2018, 1802008 CrossRef PubMed.
- S. P. Parwe, P. N. Chaudhari, K. K. Mohite, B. S. Selukar, S. S. Nande and B. Garnaik, Int. J. Nanomed., 2014, 9, 1463–1477 Search PubMed.
- N. Doroshenko, S. Rimmer, R. Hoskins, P. Garg, T. Swift, H. Spencer, R. M. Lord, M. Katsikogianni, D. Pownall, S. MacNeil, C. W. I. Douglas and J. Shepherd, Biomater. Sci., 2018, 6, 2101–2109 RSC.
- U. Tehler, J. H. Fagerberg, R. Svensson, M. Larhed, P. Artursson and C. A. Bergström, J. Med. Chem., 2013, 56, 2690–2694 CrossRef CAS PubMed.
- G. Moad, E. Rizzardo and S. H. Thang, Aust. J. Chem., 2005, 58, 379–410 CrossRef CAS.
- J. Ma, C. Cheng, G. Sun and K. L. Wooley, Macromolecules, 2008, 41(23), 9080–9089 CrossRef CAS PubMed.
- H. Y. Park, C. J. Kloxin, M. F. Fordney and C. N. Bowman, Dent. Mater., 2012, 28, 888–893 CrossRef CAS PubMed.
- Y. Li, E. Themistou, B. P. Das, L. Christian-Tabak, J. Zou, M. Tsianou and C. Cheng, Chem. Commun., 2011, 47, 11697–11699 RSC.
- M. Zhang, M. A. Puska, M. G. Botelho, E. S. Säilynoja and J. P. Matinlinna, J. Oral Sci., 2016, 58, 15–22 CrossRef CAS PubMed.
- P. Pageni, P. Yang, Y. P. Chen, Y. Huang, M. Bam, T. Zhu, M. Nagarkatti, B. C. Benicewicz, A. W. Decho and C. Tang, Biomacromolecules, 2018, 19, 417–425 CrossRef CAS PubMed.
- K.-T. Huang, Y.-L. Fang, P.-S. Hsieh, C.-C. Li, N.-T. Dai and C.-J. Huang, Biomater. Sci., 2017, 6, 1072–1081 RSC.
- M. T. P. Albuquerque, M. C. Valera, C. S. Moreira, E. Bresciani, R. M. D. Melo and M. C. Bottino, J. Endod., 2015, 41, 710–714 CrossRef PubMed.
- H. Zengin and A. H. Baysal, Molecules, 2014, 19, 17773–17798 CrossRef PubMed.
- A. Punia, A. Mancuso, P. Banerjee and N. L. Yang, ACS Macro Lett., 2015, 4, 426–430 CrossRef CAS.
- W. Qian, J. Qiu, J. Su and X. Liu, Biomater. Sci., 2018, 6, 304–313 RSC.
Footnote |
† Electronic supplementary information (ESI) available. See DOI: 10.1039/c8bm01228h |
|
This journal is © The Royal Society of Chemistry 2019 |
Click here to see how this site uses Cookies. View our privacy policy here.