DOI:
10.1039/C8CE01563E
(Communication)
CrystEngComm, 2019,
21, 23-29
Surfactant assisted self-assembly of Ag+ containing nanocrystals and their facet dependent photocatalytic activity†
Received
12th September 2018
, Accepted 14th November 2018
First published on 15th November 2018
Abstract
This work demonstrates a general route for synthesis of nanocatalysts containing silver ions using surfactants. A non-ionic surfactant Tween 80 has been used to initiate the nucleation and thereafter control the growth of silver nitroprusside (SINP) and silver phosphate (Ag3PO4) nanoparticles. Hexamine was added to study the influence of basic media on particle growth. Ag3PO4 nanoparticles with a size distribution of 30–50 nm and SINP nanoparticles with a size distribution of 30–40 nm have been obtained. A leaf-like morphology with exposed (11−1) and (022) facets has been obtained in nano SINP treated with hexamine. Evaluation of the Mulliken atomic charges by TDDFT calculations revealed maximum negative charge in the FeC5N cluster thus confirming its maximum localization of charges owing to the MLCT effect. The photocatalytic and electrocatalytic properties of the developed samples have been evaluated to establish the benefits of facet-tuning in nanoparticles. Thus the novelty of this work lies in the facet engineering of a coordinated metal complex (SINP) harnessing the MLCT effect to optimize photocatalytic activity.
1 Introduction
Since the dawn of the 21st century, photocatalysis has been applied in various fields to combat several issues. The ever increasing population and extensive industrialization have resulted in an energy crisis and severe environmental pollution. In this regard, photocatalysis has been applied to combat such challenges by applications in wastewater treatment,1 water splitting to produce hydrogen fuel2 and CO2 reduction to fuels.3 In a photocatalytic process, solar energy irradiation on a developed catalyst results in generation of charge carriers which are responsible for various redox reactions. With the advent of nanoscience, there have been numerous reports on development of nanoscale photocatalysts owing to enhanced redox reactivity resulting from quantum size effects,4 higher fractions of atoms at the surface of nanoparticles5 and higher surface area to volume ratios.6 Investigations have been carried out on manipulating structural properties at a nanoscale level for optimizing photocatalytic activity. There are primarily two constraints, which must be successfully addressed, on photocatalyst development. One is accomplishing visible light activity of the photocatalyst. Upon incidence of light energy equal to or greater than the band gap of the photocatalysts, the electrons in the valence band (VB) become excited and migrate to the conduction band (CB) thereby generating electron hole pairs. These electrons and holes initiate the redox reactions involved in photocatalysis. The generation of such electron hole pairs in the presence of visible light rather than UV light facilitates the applicability of the process. These generated electrons and holes have a tendency towards recombination, suppression of which is another constraint on photocatalyst development. Several techniques have been employed to suitably tune the band gap, electronic configuration and optical properties of developed photocatalysts, like metal doping,7 non-metal doping,8 semiconductor coupling,9 and newly developed techniques like metal ligand charge transfer (MLCT) sensitization10 and facet engineering.11 In this context, there have been several reports on development of Ag containing nanocrystalline compounds for photocatalytic activity. Ag2O has been synthesized by a simple solid state chemical reaction between AgNO3 and NaOH,12 Ag3VO4 powders have been synthesized by a precipitation method using AgNO3 and NH4VO3,13 while Ag3PO4 nanorods have been synthesized following laser ablation in a liquid technique.14 Thus a general procedure for the synthesis of Ag containing nanocrystals has not been demonstrated, which limits the scope of their applicability. Surfactants are amphiphilic compounds which have been used to control the nucleation and growth of several nanoparticles like SnO,15 TiO2,16 BiOCl–ZnO,17etc. The implementation of surfactants in the assembly of silver containing nanocompounds must be explored.
Facet engineering is a technique used to tailor the atomic level defects of nanoscale photocatalysts so that the facets exhibiting maximum photocatalytic activity mainly participate in the photocatalytic process. The facets influence the photocatalytic activity by determining the surface atomic arrangement, surface electronic band structures and localization of electrons and holes on different facets resulting in enhanced charge separation.18 There have been several reports on facet engineering of several photocatalytic nanocrystals like ZnO,19,20 TiO2,21 Ag2WO4,22etc. In all these works, the facets exhibiting the highest photocatalytic activity have been reported. However, there have been no reports on facet engineering of a composite photocatalyst implementing MLCT. The presence of the MLCT effect in silver nitroprusside has been demonstrated in our previous work.23
This work aims to establish a general procedure for the synthesis of Ag containing nanocrystals by a surfactant assisted method. The role of surfactants in controlling the development of Ag2[Fe(CN)5NO] and Ag3PO4 crystals has been demonstrated. XRD/FESEM/EIS/PL spectroscopy characterization techniques have been conducted on the synthesized samples to analyze their morphological and electronic properties. The photocatalytic activities of the developed samples have been determined by degradation of clioquinol solution in the presence of visible light. The development of the (11−1) and (022) facets in nano silver nitroprusside (nano SINP) with a leaf-like morphology and their influence on photocatalytic activity have also been discussed. TDDFT calculations have been carried out to calculate the Mulliken atomic charges of each cluster to predict the sites for photocatalytic activity in the prepared samples.
2 Experimental
2.1 Synthesis
SINP synthesis following the ion exchange route was reported in our previous work.23 The same method was employed in this work with the addition of a surfactant for developing SINP nanocrystals. A surfactant Tween 80 (0.05 g) was first dissolved in water for surfactant assisted assembly of SINP powder. Then aqueous solutions of sodium nitroprusside (Na2[Fe(CN)5NO], Merck, 99%) and silver nitrate (AgNO3, Merck, 99%) were added to the Tween 80 solution. The resulting mixture was stirred under sonication for 1 hour. Hexamine was added to maintain the pH as alkaline media. A light pink colour precipitate was observed which was centrifuged 3 times thoroughly with deionized water and then dried at 70 °C under vacuum for 3 hours. A sample without hexamine addition was also prepared for investigating the influence of hexamine on nanocrystal development. Silver phosphate has been prepared by a surfactant assisted precipitation method. 0.05 M solution of sodium phosphate salt (Na3PO4, Merck, 96%) was first prepared. The solution was added dropwise to a 0.1 M AgNO3 aqueous solution in which 0.05 g of Tween 20 had been previously dissolved. Hexamine was then added to maintain the pH as alkaline media. The yellow suspension was heated at 80 °C for 1 hour with continuous stirring and then filtered, rinsed with distilled water 3 times, dried overnight, and heated at 100 °C for 1 hour. The Ag3PO4 crystals were thus obtained. Ag3PO4 nanocrystals were also prepared using the same procedure without addition of the surfactant, for comparison with nano Ag3PO4 crystals.
2.2 Characterization
Distinctive portrayal techniques were utilized to obtain insights into the inorganic framework of the synthesized samples. Powder X-ray diffraction (PXRD) patterns of the samples were recorded on a PANalytical diffractometer (model: PW-305/60) with Cu-Kα radiation (40 kV and 30 mA) in the 2θ range of 10 °C to 60 °C. The obtained diffractogram was then compared with published files from the Joint Committee on Powder Diffraction Standards (JCPDS) and Cambridge Crystallographic Data Centre (CCDC) to identify the crystalline phases. Field emission scanning electron microscopy (FESEM-ZEOL) was used to observe the surface morphologies of the prepared samples. High resolution transmission electron microscopy (HR-TEM) was used to further explore the surface morphology. A JEM-2100 HRTEM (JEOL, Japan) with a point to point resolution of 0.195 nm and a lattice resolution of 0.14 nm was used. Photoluminescence spectra in the visible range were recorded with a Hamamatsu R928 photomultiplier detector. A He–Cd laser (325 nm) was used as the excitation source and a TRIAX-320 monochromator was used to transmit selected wavelengths of light. Absorption spectra were recorded on a LAMBDA 25 UV/VIS spectrophotometer (Perkin Elmer, India).
2.3 Photocatalytic experiment
The photocatalytic activity of the prepared samples was investigated by photocatalytic degradation of clioquinol (CLQ) in a 500 ml cylindrical glass reactor. A CFL lamp (λ > 400 nm, Philips, India) with a cutoff filter was used as the light source. It was placed inside a quartz tube mounted centrally on the pivot of the reactor. The photodegradation took place inside the annular space and a peristaltic pump at the base of the air supplied moderate air of 0.5 LPM to foment the reaction liquid. A 20 ppm solution of CLQ was prepared and 0.05 g of the prepared nanocatalysts were dispersed in it. At first, the solution was vigorously treated with air for 2 hours in the reactor in the absence of light to reach adsorption–desorption equilibrium. Thereafter light was applied and 3 ml of the sample was collected at an interval of 15 minutes and centrifuged at 10
000 rpm to separate the dispersed nanocatalysts. The absorbance of the samples was checked using a UV-VIS spectrophotometer and the concentration was obtained from a previously prepared calibration curve.
2.4 Photoelectrochemical studies
The photoelectrochemical studies were conducted on a conventional three electrode cell. The working electrode was prepared by fabricating a 30 mg sample sonicated with 9 ml ethanol and 1 ml Nafion on an ITO glass cell with a surface area of 1 cm2. A Ag/AgCl electrode and a Pt wire were used as the reference electrode and counter electrode, respectively. A 300 W xenon lamp with λ > 400 nm served as the light source. The photoelectrochemical cell was connected to a CHI643B electrochemical workstation with 1.0 M KOH solution as an electrolyte.
3 Results and discussion
3.1 PXRD characterization
The powder XRD patterns of the synthesized silver nitroprusside and Ag3PO4 samples were recorded to determine their crystallinity and lattice structures. The X-ray diffractogram of the nano SINP sample is presented in Fig. 1a. The peaks at 13.16°, 13.8° and 19.1° correspond to the (10−1), (010) and (11−1) facets of monoclinic silver nitroprusside in the space group Pc (JCPDS no. 520366: a = 7.4254 Å, b = 6.4121 Å, c = 11.8729 Å, β = 115.16°). These facets represent the coordination bond between the Ag atom and nitroprusside anion through the N end of the CN ligand. This bond and the unlinked NO ligand have been depicted in the crystal structure presented in Fig. 5. The XRD patterns of the as-synthesized nano SINP and SINP samples synthesized in our previous work23 have been compared. We can observe broader peaks with no shift in the peak position in the case of nano SINP, resulting in higher values of FWHM. This indicates smaller crystallite sizes in nano SINP, following the Scherrer equation.24 The XRD patterns show a marked change upon addition of hexamine, with the appearance of a broad peak at 37.8°, which corresponds to the (022) facet, and a broader peak at 19.1° corresponding to the (11−1) facet. From the intensities of the peaks, it can be estimated that the (11−1) and (022) facets are present in equal percentages. It can be seen that these facets are present at right angles upon calculating the theoretical interplanar angle between the facets using the following equation: | 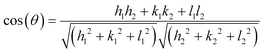 | (1) |
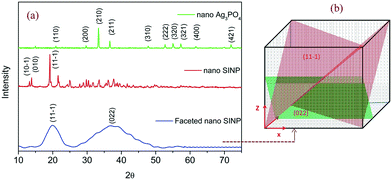 |
| Fig. 1 a) XRD patterns of nano Ag3PO4, nano SINP and faceted nano SINP. b) Facets of SINP in a unit cell. | |
This has been further validated by HRTEM analysis which revealed an angle of 90° between the lattice fringes of the (11−1) and (022) facets, as discussed later. Thus we can conclude the development of nanocrystals with exposed (11−1) and (022) facets upon hexamine addition, as shown in the schematic presented in Fig. 1b.
The XRD patterns of the as-synthesized nano Ag3PO4 are presented in Fig. 1a. The strong peaks observed at 20.83°, 29.66°, 33.27°, 36.55°, 42.47°, 47.78°, 52.68°, 54.98°, 57.24°, 61.60°, 65.87°, 70.03° and 72.3° correspond to the (110), (200), (210), (211), (220), (310), (222), (320), (321), (400), (411), (420) and (421) crystal planes of cubic phase Ag3PO4, respectively (CCDC No. 1007043; space group, P
3n; a = 6.0110 Å). Ag3PO4 has a body centered cubic structure with a lattice parameter of 6.011 Å. An isolated regular PO4 tetrahedron (P–O distance 1.539 Å) forms the body centered cubic lattice while the Ag atoms are distributed on the surface on the cube forming Ag–O–P bonds, as shown in the crystal structure presented in Fig. 5. This crystal structure was confirmed by the XRD data. Similar to what was observed in the case of SINP, nano Ag3PO4 powders showed broader peaks with no shift in the peak position, indicating smaller crystallite sizes.
3.2 Morphological characterization
FESEM and HRTEM images have been used to investigate the morphological structures of the synthesized samples. From the images in Fig. 2 the development of nanoparticles upon surfactant addition can be clearly observed. The Ag3PO4 nanoparticles are presented in Fig. 2a–c. The average particle size of nano Ag3PO4 crystals has been found to be 30–50 nm, while higher magnification revealed a lattice fringe with a lattice d spacing of 2.7 Å corresponding to the (210) facet of cubic Ag3PO4. The corresponding SAED patterns confirmed their highly crystalline nature. No change in morphology was observed after hexamine addition. The development of nano SINP upon addition of the surfactant is clearly observable in Fig. 2d. The average particle size of nano SINP was found to be 30–40 nm, while higher magnification revealed a lattice fringe with a lattice d spacing of 4.6 Å corresponding to the (11−1) facet of monoclinic SINP. A change in morphology was observed upon hexamine addition. From Fig. 2g, we can observe the development of a nanoleaf with lattice fringes crossing each other. An intersection of lattice fringes with d-spacings of 4.6 Å and 2.4 Å at right angles was observed, corresponding to the (11−1) and (022) facets, respectively (Fig. 2i). This reveals a particular leaf-like crystal growth pattern with mainly exposed (11−1) and (022) facets. This particular orientation can be confirmed from the X-ray diffractograms which revealed the presence of the (11−1) and (022) facets in approximately equal percentages at right angles to each other, as calculated theoretically. It can thus be concluded that basic media control the crystal development by activating the nucleation sites for particular facets of the nano SINP.
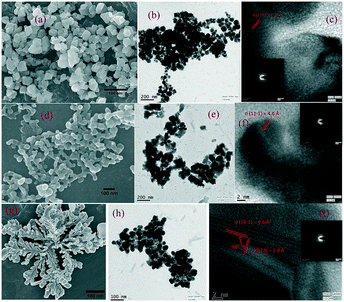 |
| Fig. 2 FESEM, HRTEM and lattice fringes including SAED patterns of nano Ag3PO4 (a–c), nano SINP (d–f), and faceted SINP (g–i). | |
3.3 Proposed mechanism for surfactant assisted nanoparticle formation
A possible mechanism for assembly of SINP and Ag3PO4 nanoparticles has been discussed here, following the schematic presented in Fig. 3. Tween 80 is a non-ionic surfactant with hydrophilic polyether groups. The Ag+ ions from AgNO3 interact with the polyether oxygen atoms in the C–O–C groups and a surface binding reaction takes place due to lone pairs of electrons on the oxygen atoms. Upon dissolution of sodium nitroprusside (Na2[Fe(CN)5NO]), the [Fe(CN)5NO]2− ions react with the bound Ag+ ions. Thus SINP nuclei are formed around the polyether oxygen atoms on the surfactant chains. This is followed by layer by layer assembly of the SINP nuclei resulting in the formation of SINP nanoparticles. These surfactant chains prevent agglomeration of SINP nanoparticles thus ensuring that the nanoparticles maintain their dimensions. The surfactants are removed by thorough washing with water, resulting in discrete SINP nanoparticles. The addition of hexamine creates a basic medium which results in the development of a nano leaf-like morphology with exposed (11−1) and (022) facets. It can be inferred that the lone pair of electrons on the nitrogen atom of the Lewis base hexamine plays an active role in controlling the orientation of the nanoparticles. Ag3PO4 nanoparticles are formed in a similar manner whereby the PO43− ions react with surface bound Ag+ ions resulting in discrete Ag3PO4 nanoparticles.
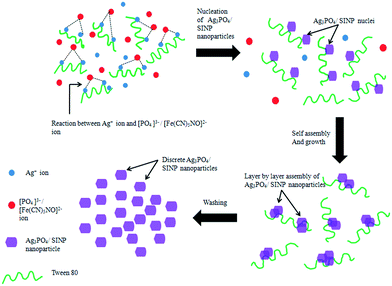 |
| Fig. 3 Mechanism for surfactant assisted assembly of SINP nanoparticles. | |
3.4 Photoluminescence spectra
The photoluminescence spectra of the prepared samples were recorded, to assess the electron hole recombination and gain insights into the defect density of the samples. From Fig. 4a we can observe two peaks at 775 nm and 687 nm in the case of the SINP samples, after Gaussian fitting. This can be attributed to more than one optically active site in the SINP sample which arises from significant atomic level defects in the complex coordination compound. Such characteristic deformities in the lattice structures may arise from the presence of Ag ions in the Fe sublattice, the presence of Fe ions in the Ag sublattice, and vacant sites in both Ag and Fe sublattices. We can observe a significant reduction in PL intensity for faceted nano SINP indicating lesser electron hole recombination and better charge separation in the case of nanoparticles with exposed (11−1) and (022) facets. The PL spectra of Ag3PO4 samples reveal a peak at 460 nm which can be attributed to characteristic blue emissions from the tetrahedral PO4 clusters.25 Similar to SINP, Ag3PO4 nanoparticles exhibit a marked reduction in PL intensity which can be attributed to more efficient charge in the case of nanoparticles. The PL spectrum of SINP in general shows lower intensity than that of Ag3PO4 indicating better charge separation due to the MLCT effect in SINP.
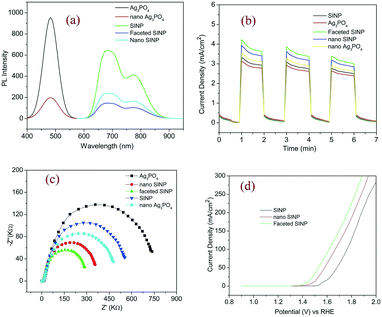 |
| Fig. 4 a) PL spectra, b) transient photocurrent response, c) Nyquist plots and d) LSV plots. | |
3.5 Photocurrent response
A photocurrent response test was conducted using electrodes fabricated with the prepared samples to estimate their photocurrent generation capabilities and charge separation efficiency. From the photocurrent density vs. time response plots in Fig. 4b, we can observe the maximum photocurrent generation for faceted nano SINP thus confirming its least electron hole recombination, as inferred from the PL spectra. The photocurrent density of the nano SINP sample is 1.3 times that of SINP, while the photocurrent density of faceted nano SINP is 1.05 times greater than that of nano SINP, indicating improved localization of charges and charge separation on the exposed (11−1) and (022) facets. Similarly nano Ag3PO4 exhibits 1.2 times higher photocurrent density than Ag3PO4. Thus it can be concluded that the nanoparticle synthesis results in a reconstruction of the electronic band structures resulting in enhanced electron hole separation ability. All the synthesized samples showed a similar response in the second run and slightly reduced response in the third run, revealing their structural and electronic stability.
3.6 Electrochemical impedance spectroscopy
EIS was conducted on the prepared samples to estimate their impedances to photocurrent generation. From the Nyquist plots presented in Fig. 4c, we can observe the minimum arc radius in the case of faceted nano SINP particles indicating their minimum impedance to charge separation resulting in the highest drift current. This is followed by the arc radii of nano SINP, nano Ag3PO4, SINP, and Ag3PO4 in ascending order. This results in the highest drift current generation in faceted nano SINP, followed by nano SINP, nano Ag3PO4, SINP and Ag3PO4, which is in agreement with the transient photocurrent responses. Thus it can be confirmed that the maximum charge separation took place in the case of faceted nano SINP, resulting in its minimum impedance and highest photocurrent generation. The highest photocurrent generation in the case of faceted nano SINP can be attributed to the creation of more active reaction sites on the (11−1) and (022) facets. The exposure of these facets results in a more tuned alignment of the electronic band configurations, by increasing the sites for hole generation (oxidation) and electron trapping (reduction). This suppression of electronic hole recombination, along with the MLCT effect, leads to the highest drift current generation in nano SINP.
3.7 Electrocatalytic oxygen evolution
Linear sweep voltammetry (LSV) was conducted on the synthesized faceted nano SINP and SINP samples to compare their oxygen evolution potentials and electrocatalytic properties. The experiments were performed at a scanning rate of 100 mV s−1 with 1 M KOH as an electrolyte. From the LSV plots presented in Fig. 4d, we can observe an OER onset potential at 1.6 V (vs. RHE) with a measured current density of 60 mA cm−2 for SINP and an overpotential of 370 mV. In the case of nano SINP, spontaneous oxygen evolution is found to occur at 1.5 V with an overpotential of 270 mV, while faceted nano SINP exhibits oxygen evolution at 1.4 V with an overpotential of 170 mV. This reveals improved electrocatalytic properties in the case of faceted nano SINP. Oxygen evolution occurs in SINP due to a reaction between the unlinked NO+ ligand in SINP and OH− ions obtained from the electrolyte. Thereby a complex NO2
anion is formed which rejects an electron to produce oxygen. The reactions have been presented below:
3.8 Mulliken orbital analysis
The Mulliken atomic charges of each atom in both Ag3PO4 and Ag2[Fe(CN)5NO] have been calculated theoretically, as shown in Fig. 5. The Mulliken atomic charges have been calculated using an ab initio, DFT and semi-empirical SCF-MO package.26 Cluster charges were calculated to predict the sites for maximum localization of charges. Cluster charges for FeC5N were calculated by EFe + EC1 + EC2 + EC3 + EC4 + EC5 + EN. Similarly the cluster charges for Ag3O–P and PO4 in the Ag3PO4 samples were calculated. We can see the octahedral FeC5N carries maximum negative charge resulting in maximum sites for redox reaction. This explains the higher photocatalytic activity of Ag2[Fe(CN)5NO], compared to Ag3PO4, as discussed later. The highest negative charge in FeC5N can be attributed to the maximum charge separation caused by the MLCT effect in SINP samples.
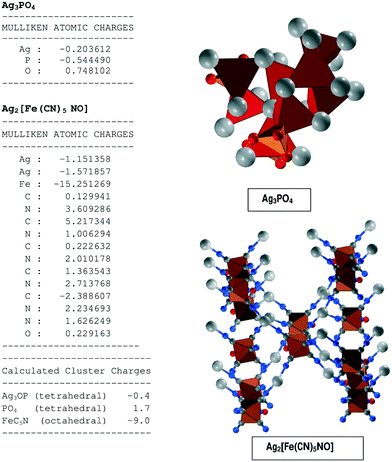 |
| Fig. 5 Mulliken orbital analysis. | |
4 Photocatalytic activity test
The photocatalytic activities of the developed samples have been tested by visible light degradation of clioquinol in the photocatalytic experimental setup as described earlier. The degradation of clioquinol as a function of time is presented in Fig. 6. All the samples exhibited visible light activity since the band gaps of both Ag3PO4 (2.45 eV)27 and SINP (1.65 eV)23 can be harvested by visible light. The enhancement of photocatalytic activity upon formation of nanoparticles is evident from the plots. It can be seen that the faceted nano SINP achieved the maximum degradation of above 95%, followed by nano SINP which achieved 95% degradation. This was followed by nano Ag3PO4 which achieved around 90% degradation, while SINP and Ag3PO4 powders achieved the least degradation percentages of 82% and 78%, respectively. The higher degradation percentage in nano SINP compared to that in Ag3PO4 can be attributed to the MLCT effect in SINP resulting in enhanced charge separation. This results in the presence of more holes and electrons on the SINP surface enabling improved redox reactions. The modified nano SINP samples with exposed (11−1) and (022) facets showed the maximum degradation owing to the enhanced charge localization on the engineered facets. This can also be due to the maximum density of surface uncoordinated atoms on the exposed facets28 leading to suitable electronic reconfiguration for maximum charge separation. It can be observed that the photocatalytic degradation follows a pseudo first order reaction owing to the linear fit between ln(C0/C) and t (Fig. 6b).
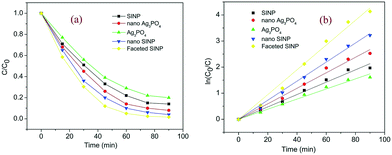 |
| Fig. 6 a) Photocatalytic activity of prepared samples and b) degradation kinetics of clioquinol. | |
5 Conclusion
The role of surfactants in the assembly of nanoparticles containing silver atoms has been discussed here. A non-ionic surfactant Tween 80 has been used to control the nucleation and growth of silver nitroprusside (SINP) and Ag3PO4 nanoparticles. The morphology of nano SINP has been further modified by hexamine addition which resulted in the growth and exposure of the (11−1) and (022) facets. The FESEM images confirmed the development of nanoparticles upon surfactant addition, while the HRTEM images revealed an intersection of lattice fringes in the nano SINP treated with hexamine, which confirmed its exposed (11−1) and (022) facets. The crystalline structures of the developed nano SINP and nano Ag3PO4 have been established from the peaks obtained in their X-ray diffractograms. These have been compared with the X-ray diffractograms of SINP and Ag3PO4 powders to establish the higher crystallinity in the case of the nanoparticles. A mechanism for the surfactant assisted assembly of nanoparticles has been proposed, from which it can be concluded that the binding of nuclei to the surfactant chains prevents agglomeration and subsequently enables growth of nanoparticles by layer by layer assembly. The PL spectra of the synthesized samples showed minimum electron hole recombination for the faceted nano SINP owing to both improved charge localization on the exposed facets and the MLCT effect. This has been further validated by the transient photocurrent response and Nyquist plots of the synthesized samples. The maximum current density and minimum impedance obtained for the faceted nano SINP are in well agreement with our findings. The photocatalytic activities of the developed samples have been compared by degradation of an aqueous solution of clioquinol in the presence of visible light. The maximum degradation (97%) achieved by faceted nano SINP demonstrates the presence of a more optimized electronic configuration in the (11−1) and (022) facets, which results in more active sites for redox reactions. The electrocatalytic properties of the faceted nano SINP and nano SINP have been established by a LSV test which revealed an OER onset potential of 1.5 eV and 1.4 eV for the nano SINP and faceted nano SINP, respectively. The Mulliken atomic charges of the synthesized samples have been calculated by TDDFT calculations to establish the maximum localization of charges on the nano SINP samples owing to the MLCT effect. Thus a general procedure for nanoparticle synthesis and the benefits of facet engineering for improved photocatalytic and electrocatalytic properties in nanocrystals have been established.
Conflicts of interest
There are no conflicts to declare.
References
- J. Carbajo and A. Bahamonde, Mol. Catal., 2017, 434, 167–174 CrossRef CAS
.
- V. Preethi and S. Kanmani, Mater. Sci. Semicond. Process., 2013, 16, 561–575 CrossRef CAS
.
- J. Hong, W. Zhang, J. Ren and R. Xu, Anal. Methods, 2013, 5, 1073–1356 RSC
.
- V. Kamat and B. Patrick, J. Phys. Chem., 1992, 96, 6829–6834 CrossRef
.
- A. Henglein, Chem. Rev., 1989, 89, 1861–1873 CrossRef CAS
.
- A. Hoffman, H. Yee and M. Hoffmann, J. Phys. Chem., 1992, 13, 5546–5552 CrossRef
.
- J. Bloh, R. Dillert and D. Bahnemann, J. Phys. Chem. C, 2012, 116, 25558–25562 CrossRef CAS
.
- R. Marschall and L. Wang, Catal. Today, 2014, 225, 111–135 CrossRef CAS
.
- J. Xu, W. Wang, S. Sun and W. Lu, Appl. Catal., B, 2012, 126–132 CrossRef CAS
.
- G. Zhang, G. Kim and W. Choi, Energy Environ. Sci., 2014, 7, 954–966 RSC
.
- G. Liu, J. Yu, G. Qing and H. Cheng, Chem. Commun., 2011, 47, 6763–6783 RSC
.
- H. Xu, J. Xie, W. Jia, G. Wu and Y. Cao, J. Colloid Interface Sci., 2018, 516, 511–521 CrossRef CAS PubMed
.
- R. Konta, H. Kato, H. Kobayashib and A. Kudo, Phys. Chem. Chem. Phys., 2003, 5, 3061–3065 RSC
.
- Z. Lin, J. Li, Z. Zheng, J. Yan, P. Liu, C. Wang and G. Yang, ACS Nano, 2015, 9, 7256–7265 CrossRef CAS PubMed
.
- B. Haspulat, M. Sarbel and H. Kams, Arabian J. Chem., 2017 DOI:10.1016/j.arabjc.2017.02.004
, in press.
- D. Liao and B. Liao, J. Photochem. Photobiol., A, 2007, 187, 363–369 CrossRef CAS
.
- R. Halvaeifard and S. Sharifnia, Korean J. Chem. Eng., 2018, 35, 770–776 CrossRef CAS
.
- Y. Peng and S. Tsang, Nano Today, 2018, 18, 15–34 CrossRef CAS
.
- E. Debroye, J. Loon, H. Yuan, K. Janssen, Z. Lou, S. Kim, T. Majima and M. Roeffaers, J. Phys. Chem. Lett., 2017, 8, 340–346 CrossRef CAS PubMed
.
- X. Liu, L. Ye, Y. Liu, S. Li and X. Ji, Sci. Rep., 2016, 6, 38474 CrossRef CAS PubMed
.
- S. Gao, W. Wang, Y. Ni, C. Lu and Z. Xu, J. Alloys Compd., 2015, 647, 981–988 CrossRef CAS
.
- R. Roca, J. C. Sczancoski, I. C. Nogueira, M. Fabbro, H. Alves and L. Cavalcante, Catal. Sci. Technol., 2015, 5, 4091–4107 RSC
.
- S. Rahut, A. Bharti and J. Basu, Catal. Sci. Technol., 2017, 7, 6092–6100 RSC
.
- A. Patterson, Phys. Rev., 1939, 56, 978–982 CrossRef CAS
.
- G. Botelho, J. Sczancoski, J. Andres, L. Gracia and E. Longo, J. Phys. Chem. C, 2015, 119, 6293–6306 CrossRef CAS
.
-
B. Tejerina, https://nanohub.org/resources/uvspec, 2014
.
- J. Liu, X. Fu, S. Chen and Y. Zhu, Appl. Phys. Lett., 2011, 99, 191903 CrossRef
.
- X. Wang, M. Liu, Z. Zhou and L. Guo, J. Phys. Chem. C, 2015, 119, 20555–20560 CrossRef CAS
.
Footnote |
† Electronic supplementary information (ESI) available. See DOI: 10.1039/c8ce01563e |
|
This journal is © The Royal Society of Chemistry 2019 |
Click here to see how this site uses Cookies. View our privacy policy here.