DOI:
10.1039/C9DT01917K
(Paper)
Dalton Trans., 2019,
48, 10824-10833
Realizing emission color tuning, ratiometric optical thermometry and temperature-induced redshift investigation in novel Eu3+-doped Ba3La(VO4)3 phosphors†
Received
8th May 2019
, Accepted 4th June 2019
First published on 4th June 2019
Abstract
Rare earth-doped Ba3La(VO4)3 phosphors with tunable emitting colors were firstly explored and their photoluminescence properties were systematically investigated. The experimental results show that the Ba3La(VO4)3 phosphors exhibit high-brightness self-activated emission and enable us to sensitize the luminescence of rare earth activators. Under near-ultraviolet (UV) excitation, both the broadband emission from VO43− groups and the sharp peak emissions from Eu3+ ions are observed in Ba3La(VO4)3:Eu3+ phosphors. Modulation of the emitting color from green to red can be realized by adjusting the Eu3+ doping concentration, which is assigned to an efficient energy transfer from VO43− to Eu3+ ions. Notably, the optical thermometry of Ba3La(VO4)3:Eu3+ was characterized based on the fluorescence intensity ratio of VO43− and Eu3+ emissions in the 298–573 K range, with the maximum absolute and relative sensitivities of 0.0515 K−1 and 1.77% K−1 at 298 K. In addition, similar phenomena were observed in Sm3+ and Dy3+-doped Ba3La(VO4)3 phosphors. These results verify a feasible strategy for varying the emission color and realizing optical thermometry in the single-component phosphors by adjusting the energy transfer between the host and the activator. It provides new possibilities for the design of multifunctional materials for white light-emitting diodes and non-contact thermometry.
1. Introduction
In recent years, color tunable phosphors have been widely applied in full-color displays, light-emitting diodes (LEDs), optical display devices, and biological technologies owing to their merits of facile preparation, high-brightness color tunable emission, and excellent thermal stability.1–4 So far, two main approaches, single and co-doping lanthanide ions in a matrix, are used for the multicolor tunable emission property of phosphors.5–7 In these materials, the multicolor tunable emission could be realized through many different routes, including varying the wavelength of excitation,8 controlling the energy transfer from the host/sensitizer to the activator,9,10 adjusting the crystal field environment around the dopants,11 and changing the temperature12 and composition modulation.13 Color-tunable phosphors, however, are usually obtained by exploring complicated crystal structures with different dopants or using various excitation wavelengths in the above methods. Hence, we turned our attention to develop appropriate single-doped phosphors with single phase and simple crystal structure under an identical wavelength excitation.
For luminescent materials, vanadate compounds have attracted attention as self-activated phosphors and rare earth doping hosts due to their excellent chemical stability and favorable luminescence properties.14–16 The photoluminescence (PL) properties of Sm3+-doped Sr3La(VO4)3 and Eu3+-doped Ca7(VO4)4O phosphors with multicolor emissions were reported by Du et al.17,18 The crystal structure and luminescence properties of Ca4La(VO4)3O:Eu3+ phosphors were also investigated as well.19 All these phosphors exhibit self-activated efficient luminescence from 400 nm to 750 nm originating from VO43− groups, with high quantum yield. Furthermore, the PL results of Ba3(VO4)2:3xSm3+ microparticles revealed a bifunctional platform for optical thermometry and safety signs via energy transfer from the VO43− group to Sm3+ ions.20 However, as far as we know, few studies have been concentrated on Eu3+-doped and self-activated Ba3La(VO4)3 phosphors, especially emission color tuning and temperature sensing. Thus, the photoluminescence properties of Eu3+-doped Ba3La(VO4)3 phosphors have been further investigated.
Nowadays, the fluorescence intensity ratio (FIR) strategy of optical thermometry has attracted considerable interest for temperature sensors thanks to their satisfactory advantages, such as high sensitivity, noninvasion, and rapid response, etc. There are numerous reports on optical thermometric characterization based on the FIR method with co-doped rare earth ions, such as Eu3+/Tb3+, Pr3+/Tb3+ and Eu3+/Mn4+.21–23 The optical sensing performance using the FIR of VO43−/Eu3+ in LiSrVO4 phosphors was investigated in detail in our recent work.24 In addition, single-phased LuVO4:Eu3+ phosphors with color tuning emission were reported as well.25 Regrettably, the temperature sensing by manipulating the energy transfer from VO43− to Eu3+ in LuVO4:Eu3+ phosphors was not investigated yet. As far as we know, the considerable research efforts have been devoted to the vanadate phosphors for lighting and display, but these materials were relatively less studied as ratiometric thermometers.
In this study, the photoluminescence and temperature-dependent luminescence properties of Eu3+-doped Ba3La(VO4)3 phosphors were systematically investigated. Notably, the FIR of VO43−/Eu3+ could be served as a highly sensitive temperature sensor in the 298–573 K range with comparable sensitivity performance. In addition, it is found that the excitation spectra of Ba3La0.95(VO4)3:0.05Eu3+ monitoring at 617 nm emission of Eu3+ shift to lower energy with the increase of temperature. The possible temperature quenching mechanisms involved in the present system were discussed as well. It is expected that this work may provide a new perspective for designing multifunctional materials.
2. Experimental details
2.1 Sample preparation
All the studied samples of Ba3La1−x(VO4)3:xEu3+ (x = 0–0.05) phosphors were synthesized by a high temperature solid-state reaction method. The stoichiometries of Eu2O3, La2O3, BaCO3 and NH4VO3 as starting materials were mixed and well ground for about 30 min in an agate mortar. Then the mixtures were transferred to an alumina crucible and calcined at 500 °C for 4 h and subsequently at 1000 °C for 8 h in air. After the samples were cooled down to room temperature, they were ground into a fine powder for consequent measurements. Moreover, the Ba3La(VO4)3:Sm3+ and Ba3La(VO4)3:Dy3+ phosphors were synthesized in a similar procedure.
2.2 Characterization
The phase of samples was determined using an X-ray diffractometer XD-2 (PERSEE), with Cu–Kα radiation (λ = 1.5406 Å), operating at 36 kV and 20 mA. Photoluminescence spectra at room temperature were obtained using a high-resolution spectrofluorometer FLS 920 (Edinburgh Instruments) equipped with both continuous (450 W) and pulsed xenon lamps. High-temperature PL spectra were recorded using the same spectrofluorometer interfaced with a computer-controlled electrical furnace in the range of 298–573 K.
3. Results and discussion
3.1 Crystal structure analysis
Fig. 1(a) illustrates the XRD patterns of Ba3La1−x(VO4)3:xEu3+ (x = 0, 0.005, 0.01, 0.03, 0.05) phosphors. It can be found that nearly all the diffraction peaks of the studied samples can be indexed to the Ba3La(VO4)3 data (JCPDS no. 40-0111). Besides, there are new minor BaVO3 (JCPDS no. 26-0205) impurity phases (marked with *) observed in the XRD patterns of the samples, which are weak enough to be neglected in our research. Revealed by the enlarged view of XRD patterns at the most intense diffraction peaks (see Fig. 1(b)), it can be seen that the strongest peak (105) shifts gradually towards a higher angle with increasing Eu3+ concentration, which is due to the substitution with a smaller radius of Eu3+ (0.947 Å, coordinated number CN = 6) than that of La3+ (1.032 Å, CN = 6).26 When Eu3+ substituted La3+, the interplanar distance d value decreased. Therefore, the diffraction angle θ increased according to Bragg's law. It is verified that Eu3+ had been successfully entered into the Ba3La(VO4)3 lattice at the La3+ sites.
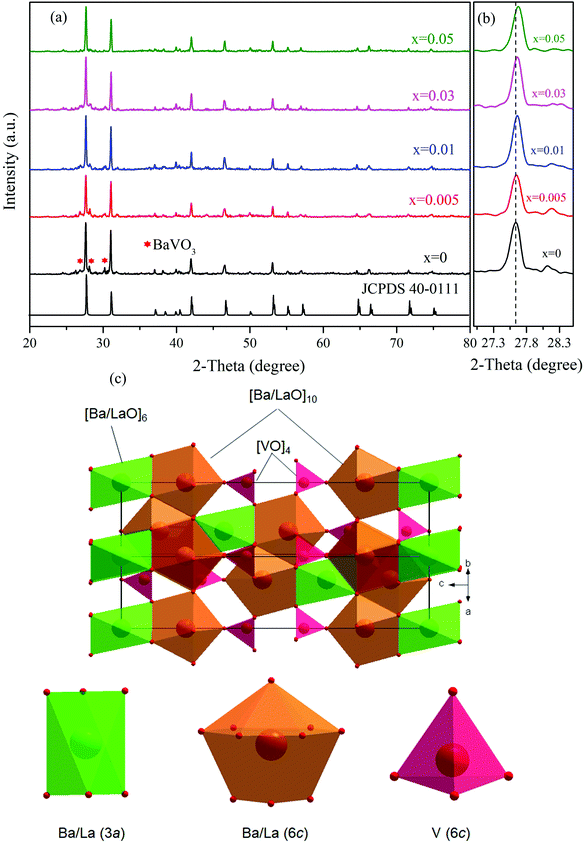 |
| Fig. 1 (a) XRD patterns of Ba3La1−x(VO4)3:xEu3+ (x = 0–0.05) samples and the standard XRD data of Ba3La(VO4)3 (JCPDS no. 40-0111). (b) Partially enlarged XRD patterns of Ba3La1−x(VO4)3:xEu3+ (x = 0–0.05). (c) Schematic illustration of the Ba3La(VO4)3 unit-cell structure and the coordination environments of Ba/La and V. | |
The Ba3La(VO4)3 phosphor crystallizes in the monoclinic phase with space group R
m, as determined by the XRD measurement. The standard values for the crystal are a = 5.75271 Å, c = 21.04729 Å and V = 603.21 Å3. The crystal structure in Fig. 1(c) reveals that there are two different kinds of lattice sites for Ba and La cations, surrounded by six oxygen ions in an octahedron configuration and ten oxygen ions in a polyhedron with 13 faces. Moreover, the V atoms are coordinated by four oxygen atoms in a tetrahedral configuration.27
3.2 Room-temperature luminescence properties of the Ba3La(VO4)3 host
The self-activated host optical properties are fascinating for luminescent materials. The excitation of the photoluminescence (PLE) and PL spectra of Ba3La(VO4)3 phosphors is presented in Fig. 2(a). The PLE spectrum exhibits a broad band, in the range of 250–380 nm, which can be decomposed into two sub-bands peaking at 313 nm (Ex1: 1A1 → 1T2) and 340 nm (Ex2: 1A1 → 1T1) attributing to the V5+ → O2− charge transfer (CT) transitions of VO43− groups.28 Under 320 nm excitation, the PL spectrum exhibits a broad emission band (400 nm to 650 nm) with the maximum emission peaking at 500 nm. This emission band is ascribed to the CT transitions of the VO43− groups.29 Meanwhile, the emission band can be well de-convoluted into two Gaussian peaks, which are attributed to the 3T2 → 1A1 (Em1 = 488 nm) and 3T1 → 1A1 (Em2 = 530 nm) transitions of VO43− groups as shown in Fig. 2(b) as Em1 and Em2, respectively. It can be seen that Ba3La(VO4)3 is an effective self-activated host, which could exhibit intense green light. It is noted that the PL and PLE spectra of Ba3La(VO4)3 are very similar to those of Sr3La(VO4)3, except that the maximum of the excitation and emission bands is shifted to longer wavelengths (320 nm and 500 nm in Ba3La(VO4)3 compared with 350 nm and 520 nm in Sr3La(VO4)3.27
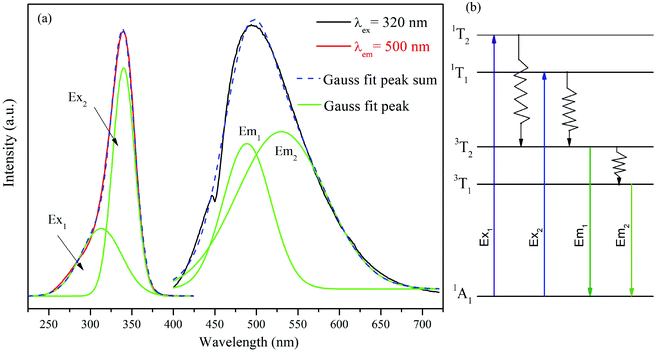 |
| Fig. 2 (a) The excitation and emission spectra of the Ba3La(VO4)3 samples; the green bands named Em1 and Em2 are obtained by the Gaussian fitting of the emission spectrum and the green bands named Ex1 and Ex2 are obtained by the Gaussian fitting of the excitation spectrum. (b) Schematic illustration of excitation and emission processes for VO43−. | |
3.3 Room-temperature luminescence properties of Eu3+-doped Ba3La(VO4)3 phosphors
As for the Eu3+-doped Ba3La(VO4)3 phosphors, we take Ba3La0.99(VO4)3:0.01Eu3+ as an example. The excitation spectrum monitored at 617 nm corresponding to the 5D0 → 7F2 transition of Eu3+ consists of two distinct regions as shown in Fig. 3. In the UV spectral region (250–350 nm), the broad absorption band located at 320 nm is assigned to the V5+ → O2− CT band as described above. Besides, the relatively weak sharp lines in the range of 370–500 nm are ascribed to the 4f–4f transitions of Eu3+ ions. According to the Dieke diagram, these transitions are identified and located at 360, 378, 391, 412, 463 and 531 nm, which are due to the intra-configurational transitions of Eu3+:7F0 → 5G4, 7F0 → 5L6, 7F0 → 5D3, 7F0 → 5D2 and 7F0 → 5D1, respectively.30 The observation of the V5+ → O2− CT band in the excitation spectra of Eu3+ implies that energy transfer from VO43− groups to Eu3+ occurs in Ba3La0.99(VO4)3:0.01Eu3+. In addition, it is worth noting that the host absorption from VO43− groups dominates in the excitation spectrum, illustrating that the studied phosphors can be efficiently excited by the near UV chips.
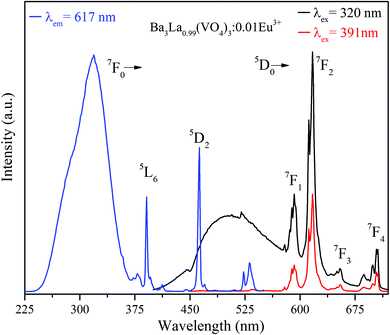 |
| Fig. 3 Excitation spectrum of Ba3La0.99(VO4)3:0.01Eu3+ phosphors monitored at 617 nm and PL emission spectra of Ba3La0.99(VO4)3:0.01Eu3+ phosphors excited at 320 and 391 nm, respectively. | |
The emission spectra of Ba3La0.99(VO4)3:0.01Eu3+ upon excitation of 320 and 391 nm are also presented in Fig. 3. At room temperature, the sample shows intense green and red luminescence from host and Eu3+ (peaking at 592, 619, 655 and 704 nm) by exciting into the CT band, whereas sharp emission red emission from Eu3+ can be only observed under 391 nm excitation. It is well known to us that the transition of 5D0 → 7F1 (592 nm) belongs to the magnetic dipole transition of Eu3+, which is less influenced by the surrounding environment and obeys the transition selection rule of Δl = 0, Δs = 0. However, the 5D0 → 7F2 (617 nm) transition is a typical electric dipole transition and is sensitive to the site symmetry of Eu3+ ions.31 The emission intensity ratio of 5D0 → 7F2/5D0 → 7F1 transitions can be applied as a good determination for the site symmetry of the rare-earth ion in the structure.32 The intensity of 5D0 → 7F2 transition is 2.5 times that of 5D0 → 7F1 transition in the case of Ba3La0.99(VO4)3:0.01Eu3+. It implies that Eu3+ ions occupy the low-symmetry lattice sites without inversion symmetry. The energy transfer efficiency ηET from VO43− to Eu3+ can be evaluated by the following equation:33
|  | (1) |
where
I0 and
I represent the emission intensities of the Ba
3La(VO
4)
3 host and Ba
3La
0.99(VO
4)
3:0.01Eu
3+ phosphors, respectively. Accordingly,
ηET can be determined to be about 66.34%.
In order to investigate the influence of the Eu3+ concentrations on the PL properties of the Ba3La1−x(VO4)3:xEu3+ (x = 0.005–0.05) phosphors, their PL spectra were recorded as well, as shown in Fig. 4(a). Under 320 nm excitation, the luminescence intensities of Eu3+ continuously increase with the increasing Eu3+ concentration up to 5 mol%. The values of CIE chromaticity coordinates and images of Ba3La1−x(VO4)3:xEu3+ phosphors under 365 nm UV lamp excitation are presented in Fig. 4(b). Obviously, it was observed that the emission color of the phosphor can be modified by controlling the Eu3+ concentration. As shown in the CIE coordinate diagram in Fig. 4(b), the emitting color can be gradually modulated from green to orange and then to yellowish brown and finally red. The images of the sample in Fig. 4(b) also show that the emission color can be tuned from green to red. Correspondingly, the CIE coordinates can be tuned from (0.2442, 0.3946) to (0.6077, 0.3499) with the increase of the Eu3+ concentration, which is attributed to the VO43− → Eu3+ energy transfer.
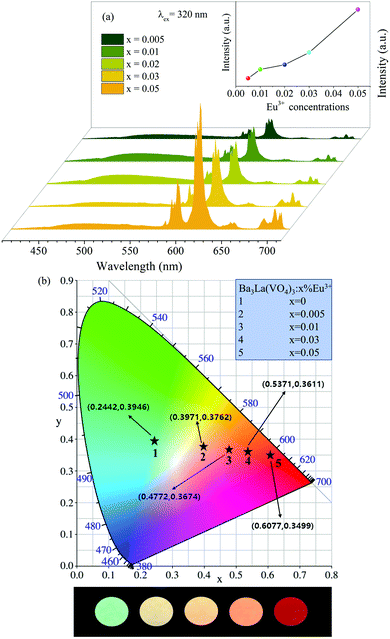 |
| Fig. 4 (a) PL spectra of the Ba3La1−x(VO4)3:xEu3+(x = 0.005–0.05) samples excited at 320 nm. (b) CIE chromaticity coordinates and images of Ba3La1−x(VO4)3:xEu3+ (x = 0.005–0.05) phosphors. | |
In order to further understand the energy transfer process, the decay lifetime of (VO4)3− in the Ba3La1−x(VO4)3:xEu3+ phosphors is shown in Fig. 5. It can be found that the lifetime of the samples changes a little with the various Eu3+ concentrations. This phenomenon indicates that the energy transfer process between (VO4)3− and Eu3+ is not due to cross relaxation between 3T1,3T2 → 1A1 of (VO4)3− and 7F0 → 5DJ(J = 0, 1 or 2) of Eu3+, but because of the exchange or super exchange mechanism reported by H. J. Seo et al.34,35 Similar phenomena have been reported previously for Sr3La(VO4)3:Eu3+ phosphors.27
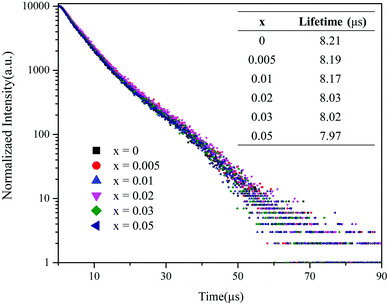 |
| Fig. 5 Decay curves (λex = 320 nm, λem = 500 nm) of (VO4)3− in the Ba3La1−x(VO4)3:xEu3+ phosphors. The inset shows the luminescence lifetime for the phosphors with different Eu3+ concentrations. | |
It is worth mentioning that the Ba3La(VO4)3 host can also sensitize the other rare earths activators, such as Sm3+ and Dy3+. The PL properties are dependent on the Sm3+ or Dy3+ concentration and the temperature, as shown in Fig. S1 and S2.† Further work on other RE3+-doped Ba3La(VO4)3 phosphors is being carried out in our further study.
The possible mechanisms involved in the energy transfer process and emission color tuning were proposed, as shown in Fig. 6. When Ba3La1−x(VO4)3:xEu3+ is excited by 320 nm into the VO43− groups, electrons are first excited from the ground state to the excited state. Then the excited electrons relax to the lower excited level, resulting in radiative green emission and eventually return to the ground state. When the Eu3+ ions are doped into it, energy absorbed by VO43− groups can be partially transferred to Eu3+via the nonradiative resonant process. This process can populate the excited levels of 5D0 through the nonradiative resonant process and subsequently give rise to the radiative transitions from 5D0 to 7FJ (J = 0, 1, 2, 3, 4). The energy transfer efficiency can be manipulated by adjusting the doping concentration of Eu3+ ions, and thus the red and green emission intensity ratio can be tuned, which enables us to control the emission color from green to red (see Fig. 6).
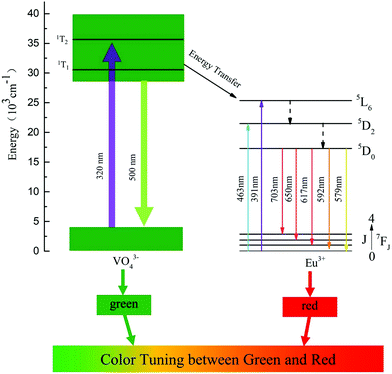 |
| Fig. 6 Energy transfer scheme for the color tuning Ba3La1−x(VO4)3:xEu3+ phosphors. | |
3.4 Temperature-sensing performance of Eu3+-doped Ba3La(VO4)3 phosphors
Traditional ratiometric luminescent thermometry materials are based on thermally coupled levels (TCLs) of rare earth ions, such as Er3+ (2H11/2/4S3/2), Eu3+(5D1/5D0), Nd3+ (4F5/2/4F3/2), Tm3+ (3F2,3/3H4), Dy3+ (4I15/2/4F9/2), Ho3+ (5S2/5F4), etc.36 In such thermometry materials, the smaller TCL gap is better for absolute sensitivity (SA) and is detrimental to relative sensitivity (SR). In addition, a smaller energy gap will result in overlapping of their two emission peaks, which is unfavorable for the detection of the emission peak signal. Another strategy for the luminescent temperature sensing method is utilizing two different rare earth ions as two luminescence centers. However, this method is mostly used for temperatures below 320 K.22 Hence, the FIR method based on single rare earth ion doping is a hopeful approach for optical thermometric characterization in a wide temperature range. In this work, the FIR of the V5+ → O2− CT band and the f–f transition of Eu3+ are dramatically changed with the increase of temperature. The ratiometric temperature sensing was studied based on the temperature dependence of emission spectra, shown in Fig. 7(a). As depicted in Fig. 7(a), the profile of the emission spectrum hardly changes with increasing temperature, whereas the FIR of the VO43− group and Eu3+ ions decreases gradually owing to the thermal quenching effect by the thermal activation via the crossover point of the excited and ground levels.37 The activation energy ΔE can be obtained according to the Arrhenius equation as described below:38 | 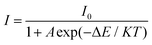 | (2) |
where I0 and I are the emission intensities at initial temperature and measured temperature T, respectively, A is a constant and K denotes the Boltzmann constant. According to eqn (2), a plot of ln[(I0/I) − 1] versus 1/KT and a slope of its linear fitting should give the activation energy (ΔE) corresponding to the thermal quenching effect of Eu3+, as depicted in Fig. 7(b), which shows the ΔE of 0.257 eV upon 320 nm excitation.
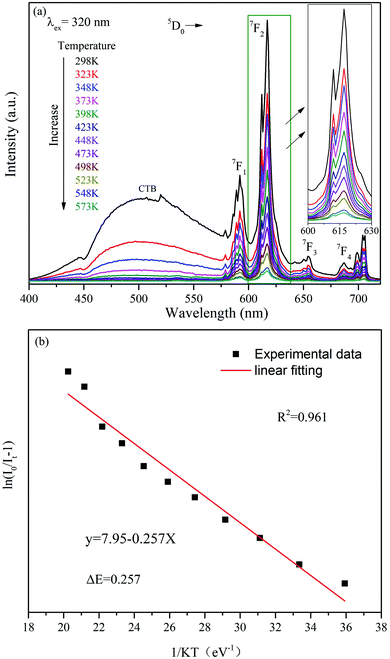 |
| Fig. 7 (a) The temperature-dependent PL spectra of the Ba3La0.99 (VO4)3:0.01Eu3+ phosphor excited at 320 nm. (b) Dependence of Eu3+ emission intensities on temperature and their linear fit. | |
Interestingly, it is observed from the inset of Fig. 8(a) that the integrated emission intensity of the VO43− group is dramatically dropped with the increase of temperature, while the Eu3+ emission intensity exhibits a relatively weaker decrease in the temperature. Therefore, ratiometric optical thermometry between VO43− (400–580 nm) emission and Eu3+ (7F0 → 5D2) emission (FIR = IVO43−/IEu3+) could be employed as a sensitive thermometric probe. As shown in Fig. 8(a), the FIR value reveals a remarkable decrease as the temperature increases. The FIR changing with various temperatures can be fitted as an exponential curve in the 298–573 K range, which could be described as follows:
|  | (3) |
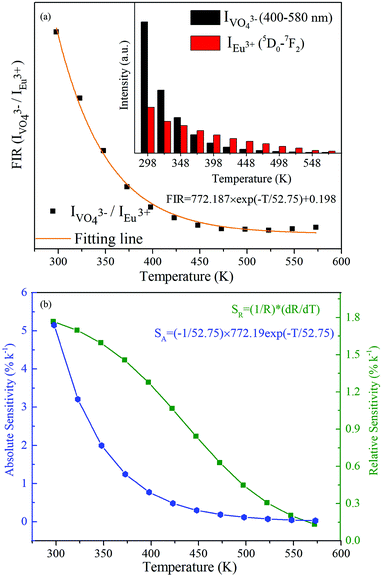 |
| Fig. 8 (a) Temperature-dependent intensity ratio (IVO43−/IEu3+) and exponential fitting. The inset shows the integrated emission intensity of the Eu3+:5D0 → 7F2 and VO43− group as a function of temperature. (b) Absolute sensitivity SA and relatively sensitivity SR values at different temperatures. | |
The sensitivity of optical thermometry is the rate of change of FIR in response to the variation of temperature T.36 There are two key parameters, i.e., the relative sensitivity (SR) and absolute sensitivity (SA), to quantify the temperature sensing properties of the material for practical applications. SR and SA are defined as the following expressions, respectively:39,40
|  | (4) |
| 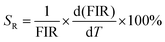 | (5) |
The temperature dependence of SA and SR values was calculated and is displayed in Fig. 8(b). As presented in Fig. 8(b), the maxima of the absolute sensitivity and relative sensitivity are 0.0515 K−1 and 1.77% K−1 at 298 K, both being comparable to those of other reported Eu3+-doped optical temperature sensing materials, whose thermometric properties were characterized based on the TCL of Eu3+ (5D1 and 5D0).41–47 Comparison results are summarized in Table 1. Furthermore, the Ba3La0.99(VO4)3:0.01Eu3+ phosphor exhibits a good repeatability after several cycling processes. The above results demonstrate its potential applications in temperature sensing with comparable sensing performance.
Table 1 Optical thermometric properties of Eu3+ ion-based doped luminescent materials
Dopant |
Host |
Temperature range (K) |
S
R (K−1) @temperature |
Ref. |
Eu3+ |
Ba3La(VO4)3 |
298–573 |
1.77%@323 K |
This work |
Eu3+ |
CaEu2(WO4)4 |
300–500 |
1.4%@300 K |
41
|
Eu3+ |
BiF3 |
303–443 |
0.0034%@443 K |
42
|
Eu3+ |
Gd2Ti2O7 |
303–423 |
0.95%@423 K |
43
|
Eu3+ |
NaEuF4 |
298–523 |
0.43% |
44
|
Eu3+ |
Ca2.94Eu0.04Sc2SiO12 |
123–273 |
1.35%@273 K |
45
|
Eu3+ |
EuFDC |
12–320 |
0.33%@300 K |
46
|
Eu3+ |
YBO3 |
333–773 |
1.8%@333 K |
47
|
3.5 Red-shift phenomenon in the excitation spectrum
Significantly, a continuous redshift of the charge transfer band in PLE was observed in the 298–573 K range. The maximum of the V5+ → O2− CT band in Fig. 9(a) band shifts from 320 nm at RT to 336 nm at 573 K, with a redshift of 16 nm and unchanged shape of the PLE spectra. Simultaneously, the decrease of PLE intensity with increasing temperature is in accordance with the observation of PL due to the thermal quenching effect.48,49 It is worth mentioning that such a temperature-induced red-shift phenomenon of the CT excitation band in Fig. 9(a) is beneficial for UV absorption with longer wavelengths. And this redshift phenomenon can be explained by the alteration in the relative location of the transition from the ground state to the CT excited states,50,51 as depicted in Fig. 9(b). Under 320 nm excitation, the VO43− groups are promoted from their ground state 1A1 to the excited states 1T1 and 1T2, and this process is called the optical absorption transition. At lower temperature, the transition to the excited states 1T1 and 1T2 starts from the lowest vibronic level ν1 of the ground state 1A1. However, this transition mainly occurs from the higher thermally populated vibronic level ν2 of the ground state at higher temperature. The thermal population of vibronic levels of the ground state leads to an excitation redshift, i.e. a shift of the excitation peak towards lower energy as the temperature increases.
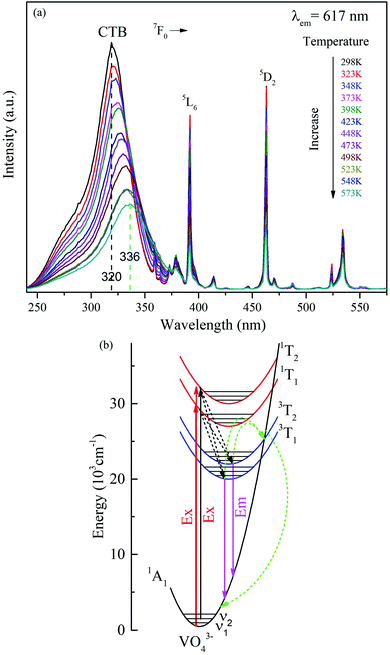 |
| Fig. 9 (a) The temperature-dependent PLE spectra of the Ba3La0.9(VO4)3:0.1Eu3+ phosphor monitored at 617 nm. (b) Schematic configurational coordinate diagram for Ba3La(VO4)3:Eu3+, and an illustration of the excitation, emission, and nonradiative transition processes. | |
The relationship between the maximum peak position of the V5+ → O2− CT band and temperature is given in Fig. 10(a), manifesting an almost linear relationship. Further analysis of the data indicates that the maximum peak position (λ) shifting can be well fitted with a linear curve λ = 0.0614T + 301.01, as shown in Fig. 10(a). The slope of the curve represents the peak changes with the temperature, revealing the absolute temperature sensitivity SA (Δλ/ΔT). The relative sensitivity SR can be further calculated according to
,52 as depicted in Fig. 10(b). Thus, the values of SA and SR are determined to be 0.0614 nm K−1 and 0.01923% K−1@298 K, respectively. Compared with previously reported materials based on the spectral shift, the value of SA (0.0614 nm K−1) is much larger than 0.033 nm K−1 in CaWO4:Ho3+ and 0.007 nm K−1 in LaF3:Nd3+.53,54 The luminescence results on the Ba3La0.99(VO4)3:0.01Eu3+ phosphor demonstrated a potential temperature sensor based on the fluorescence intensity ratio, as well as the spectral shift, giving a pathway for new luminescence materials designing.
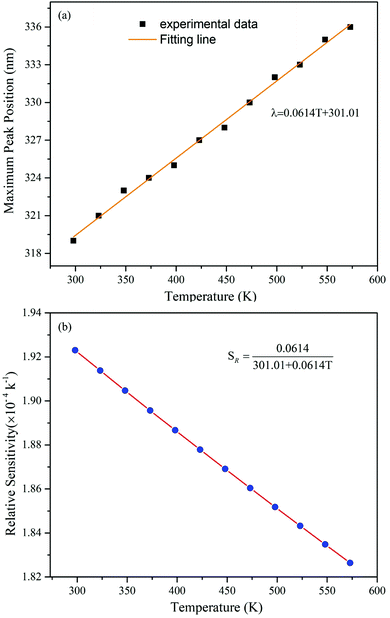 |
| Fig. 10 (a) The relationship between the maximum peak position of the V5+ → O2− CT band and temperature. (b) Relative sensitivity SR values at different temperatures. | |
4. Conclusions
In summary, Eu3+-activated Ba3La(VO4)3 phosphors were successfully synthesized by a high temperature solid state reaction method. The PL spectrum consists of a broad emission band from VO43− groups and several sharp emission peaks owing to the 5D0 → 7FJ (J = 0, 1, 2, 3, 4) transitions of Eu3+. By changing the Eu3+-doping concentration, it is possible to tune the emission color varying from green (0.2442, 0.3946) to red (0.6077, 0.3499), accordingly. The temperature-dependent luminescence properties of Ba3La0.99(VO4)3:0.01Eu3+ phosphors illustrate that the emission intensity ratio between VO43− (400–580 nm) and Eu3+ (7F0 → 5D2) can be applied as a thermometric probe with comparable temperature sensing performance. The maximum absolute sensitivity and relative sensitivity are found to be 0.0515 K−1 and 1.77% K−1@298 K, respectively. In addition, the V5+ → O2− CT band shifts toward the longer wavelength gradually with elevating temperature, showing that the studied material can be explored for temperature sensing based on the spectral shift. These results suggested that the Eu3+-activated Ba3La(VO4)3 phosphors may provide promising applications in tunable emission materials and non-contact optical thermometry.
Conflicts of interest
There are no conflicts to declare.
Acknowledgements
This project was funded by the National Natural Science Foundation of China (11674044, 11604037, and 11704054), the Chongqing Research Program of Basic Research and Frontier Technology (No. CSTC2016JCYJA0113, CSTC2016JCYJA0207, and CSTC2017jcyjAX0046), the Scientific and Technological Research Program of Chongqing Municipal Education Commission (No. KJQN201800619, KJ1600406, and KJZD-K201800602), the Wenfeng High-end Talents Project of Chongqing University of Posts and Telecommunications (W2017-06 and W2016-30) and the Scientific Research Foundation For Doctor of Chongqing University of Posts and Telecommunications (A2008-59).
References
- J. P. Xue, X. F. Wang, J. H. Jeong and X. H. Yan, Phys. Chem. Chem. Phys., 2018, 20, 11516–11541 RSC.
- J. Zhou, Q. L. Liu and Z. G. Xia, J. Mater. Chem. C, 2018, 6, 4371–4383 RSC.
- B. Li and X. Y. Huang, Ceram. Int., 2018, 44, 4915–4923 CrossRef CAS.
- R. Cao, H. Xiao, F. Zhang, Z. Luo, T. Chen, W. Li, P. Liu and G. Zheng, J. Lumin., 2019, 208, 350–355 CrossRef CAS.
- K. Li, M. Shang, H. Z. Lian and J. Lin, J. Mater. Chem. C, 2016, 4, 5507–5530 RSC.
- I. Carrasco, F. Piccinelli and M. Bettinelli, J. Phys. Chem. C, 2017, 121, 16943–16950 CrossRef CAS.
- K. Li and R. V. Deun, Dalton Trans., 2018, 47, 6995–7004 RSC.
- F. W. Kang and M. Y. Peng, Dalton Trans., 2014, 43, 277–284 RSC.
- C. Zhang, H. B. Liang, S. Zhang, C. Liu, D. Hou, L. Zhou, G. Zhang and J. Shi, J. Phys. Chem. C, 2012, 116, 15932–15937 CrossRef CAS.
- Y. Cao, J. Ding, X. Ding, X. Wang and Y. H. Wang, J. Mater. Chem. C, 2017, 5, 1184–1194 RSC.
- P. P. Dai, X. T. Zhang, L. L. Bian, S. Lu, Y. C. Liu and X. J. Wang, J. Mater. Chem. C, 2013, 1, 4570–4576 RSC.
- F. W. Kang, M. Y. Peng, D. Y. Lei and Q. Y. Zhang, Chem. Mater., 2016, 28, 7807–7815 CrossRef CAS.
- Z. G. Xia, C. G. Ma, M. S. Molokeev, Q. L. Liu, K. Rickert and K. R. Poeppelmeier, J. Am. Chem. Soc., 2015, 137, 12494–12497 CrossRef CAS PubMed.
- R. Cao, T. Chen, Y. Ren, C. Liao, Z. Luo, Y. Ye and Y. Guo, Mater. Res. Bull., 2019, 111, 87–92 CrossRef CAS.
- K. Li and R. V. Deun, Dyes Pigm., 2018, 155, 258–264 CrossRef CAS.
- R. Cao, X. Wang, Y. Jiao, X. Ouyang, S. Guo, P. Liu, H. Ao and C. Cao, J. Lumin., 2019, 212, 23–28 CrossRef CAS.
- P. Du and J. S. Yu, Luminescence, 2017, 32, 1504–1510 CrossRef CAS PubMed.
- P. Du, L. Zhou, L. Luo and J. S. Yu, New J. Chem., 2019, 43, 6688–6695 RSC.
- R. Yu, J. H. Jeong and Y. F. Wang, J. Am. Ceram. Soc., 2017, 100, 5649–5658 CrossRef CAS.
- P. Du, Y. Hua and J. S. Yu, Chem. Eng. J., 2018, 352, 352–359 CrossRef CAS.
- H. Wang, D. Zhao, Y. Cui, Y. Yang and G. D. Qian, J. Solid State Chem., 2017, 246, 341–345 CrossRef CAS.
- Y. Gao, F. Huang, H. Lin, J. Zhou, J. Xu and Y. S. Wang, Adv. Funct. Mater., 2016, 26, 3139–3145 CrossRef CAS.
- D. Q. Chen, S. Liu, Y. Zhou, Z.
Y. Wan, P. Huang and Z. G. Ji, J. Mater. Chem. C, 2016, 4, 9044–9051 RSC.
- X. J. Zhou, L. Chen, S. Jiang, G. T. Xiang, L. Li, X. Tang, X. B. Luo and Y. Pang, Dyes Pigm., 2018, 151, 219–226 CrossRef CAS.
- F. Kang, L. Li, J. Han, D. Y. Lei and M. Y. Peng, J. Mater. Chem. C, 2017, 5, 390–398 RSC.
- L. Li, X. H. Tang, Y. F. Zheng, Z. J. Wu, W. X. Chang, S. Jiang, G. T. Xiang and X. J. Zhou, J. Am. Ceram. Soc., 2018, 101, 4095–4107 CrossRef CAS.
- J. Zhou, F. Huang, J. Xu, H. Chen and Y. S. Wang, J. Mater. Chem. C, 2015, 3, 3023–3028 RSC.
- X. Y. Huang and H. Guo, Dyes Pigm., 2018, 154, 82–86 CrossRef CAS.
- T. Hasegawa, Y. Abe, A. Koizumi, T. Ueda, K. Toda and M. Sato, Inorg. Chem., 2018, 57, 857–866 CrossRef CAS PubMed.
- J. P. Xue, H. P. Li, H. M. Noh, B. C. Choi, S. H. Park, J. H. Jeong and J. H. Kim, J. Lumin., 2018, 202, 97–106 CrossRef CAS.
- A. Vlasic, D. Sevic, M. S. Rabasovic, J. Krizan, S. Savic-Sevic, M. D. Rabasovic, M. Mitric, B. P. Marinkovic and M. G. Nikolic, J. Lumin., 2018, 199, 285–292 CrossRef CAS.
- L. Li, Y. Pan, X. Zhou, C. Zhao, Y. Wang, S. Jiang, A. Suchocki and M. G. Brik, J. Alloys Compd., 2016, 685, 917–926 CrossRef CAS.
- B. Li, X. Huang, H. Guo and Y. Zeng, Dyes Pigm., 2018, 150, 67–72 CrossRef CAS.
- Z. Tao, T. Tsuboi, Y. Huang, W. Huang, P. Cai and H. J. Seo, Inorg. Chem., 2014, 53, 4161–4168 CrossRef CAS.
- H. Xie, T. Tsuboi, W. Huang, Y. Huang, L. Qin and H. J. Seo, J. Am. Ceram. Soc., 2014, 97, 1434–1441 CrossRef CAS.
- X. Wang, Q. Liu, Y. Bu, C. S. Liu, T. Liu and X. Yan, RSC Adv., 2015, 5, 86219–86236 RSC.
- K. Li, H. Lian, M. Shang and J. Lin, Dalton Trans., 2015, 44, 20542–20550 RSC.
- C. Zhao, Z. G. Xia and S. Yu, J. Mater. Chem. C, 2014, 2, 6032–6039 RSC.
- Y. Gao, F. Huang, H. Lin, J. Xu and Y. S. Wang, Sens. Actuators, B, 2017, 243, 137–143 CrossRef CAS.
- M. D. Dramićanin, Methods Appl. Fluoresc., 2016, 4, 042001 CrossRef PubMed.
- K. W. Meert, V. A. Morozov, A. M. Abakumov, J. Hadermann, D. Poelman and P. F. Smet, Opt. Express, 2014, 22, 961–972 CrossRef PubMed.
- P. Du, Y. Wu and J. S. Yu, RSC Adv., 2018, 8, 6419–6424 RSC.
- V. Lojpur, S. C. Ulrbrk and M. D. Dramicanin, J. Lumin., 2016, 169, 534–538 CrossRef CAS.
- Y. Tian, B. Tian, C. Cui, P. Huang, L. Wang and B. J. Chen, Opt. Lett., 2014, 39, 4164–4167 CrossRef CAS PubMed.
- L. Zhao, J. Cai, F. Hu, X. Li, Z. Cao, X. Wei, Y. Chen, M. Yin and C. K. Duan, RSC Adv., 2017, 7, 7198–7202 RSC.
- L. Li, Y. Zhu, X. Zhou, C. D. S. Brites, D. Ananias, Z. Lin, F. A. Almeida, P. J. Rocha, W. Huang and L. D. Carlos, Adv. Funct. Mater., 2016, 26, 8677–8684 CrossRef CAS.
- L. Zhao, Z. Cao, X. Wei, M. Yin and Y. Chen, J. Rare Earths, 2017, 35, 356–360 CrossRef CAS.
- S. S. Zhou, C. K. Duan and M. Wang, Opt. Lett., 2017, 42, 4703–4706 CrossRef CAS.
- J. Chen, Y. Zhao, Z. Y. Mao, D. J. Wang and L. J. Bie, J. Lumin., 2017, 186, 72–76 CrossRef CAS.
- L. Shi, H. J. Zhang, C. Y. Li and Q. Su, RSC Adv., 2011, 1, 298–304 RSC.
- J. W. Qiao, L. X. Ning, M. S. Molokeev, Y. C. Chuang, Q. L. Liu and Z. G. Xia, J. Am. Chem. Soc., 2018, 140, 9730–9736 CrossRef CAS PubMed.
- L. Li, S. K. Fu, Y. F. Zheng, C. Li, P. Chen, G. T. Xiang, S. Jiang and X. J. Zhou, J. Alloys Compd., 2018, 738, 473–483 CrossRef CAS.
- X. J. Zhou, R. X. Wang and G. T. Xiang, Opt. Mater., 2017, 66, 12–16 CrossRef CAS.
- U. Rocha, C. J. da Silva, W. F. Silva, I. Guedes, A. Benayas, L. M. Maestro, M. A. Elias, E. Bovero, F. C. J. M. van Veggel, J. A. García Solé and D. Jaque, ACS Nano, 2013, 7, 1188–1199 CrossRef CAS PubMed.
Footnote |
† Electronic supplementary information (ESI) available. See DOI: 10.1039/c9dt01917k |
|
This journal is © The Royal Society of Chemistry 2019 |
Click here to see how this site uses Cookies. View our privacy policy here.