Gelatin–chitosan hydrogel particles for efficient removal of Hg(II) from wastewater
Received
28th September 2018
, Accepted 2nd November 2018
First published on 5th November 2018
Abstract
Unregulated discharge of nonbiodegradable heavy metals (HMs) from mining operations, tanneries, and metal plating is posing an impending global water pollution concern. Early detection, accurate determination, and practical removal of HMs from industrial water are vital for a clean ecosystem and critical for the survival of imperiled aquatic life. Herein we report a robust, green, and cost-effective approach for the removal of heavy metal ions such as Pb(II), Hg(II), Cd(II), and Cr(III) from wastewater using a synthetic bioadsorbent made of gelatin–chitosan (GC) hydrogel particles, operated at neutral pH and laboratory temperature. The chemical and structural morphology of the synthesized hydrogel was confirmed by Fourier transform infrared spectroscopy (FTIR) and scanning electron microscopy (SEM), respectively. HM ion adsorption was first tracked by the coffee ring effect (CRE) and subsequently characterized by inductively coupled plasma mass spectrometry (ICP-MS). As compared to Pb(II), Cd(II), and Cr(III) metal ions, the hydrogel particles displayed a quite notable binding affinity for Hg(II). Also, the hydrogel particles were examined for selective adsorption of HMs. As such, this strategy is robust and cheap, and could efficiently remove Hg(II) metal ions from wastewater.
Water impact
The early detection, accurate determination, and practical removal of heavy metals from wastewater are vital for a clean ecosystem. We report a robust, green, and cost-effective approach for the removal of heavy metals ions (Pb(II), Hg(II), Cd(II), and Cr(III)) from aqueous solutions using gelatin–chitosan hydrogel particles. The removal was first effortlessly tracked by the coffee ring effect (CRE) and subsequently characterized by inductively coupled plasma mass spectrometry (ICP-MS).
|
Introduction
Inappropriate disposal of wastewater from increased industrialization and excessive human activities is having a detrimental impact on both public health and aquatic life by causing fatal diseases and disorders even at trace levels.1 Among the most common pollutants found in wastewater, heavy metals are at the top of the list. Thus, removing heavy metal ions from water during water treatment is crucial and requires urgent research attention.2 Previously, various techniques such as precipitation, adsorption, oxidation, and nanofiltration have been employed to remove hazardous heavy metal ions from aqueous solutions.3–6 In this list, the adsorption method is widely regarded as easy, versatile, and cost-effective.
Biodegradable adsorbents derived from natural precursors are being widely explored for feasible wastewater treatment, substituting conventional materials. For instance, green renewable adsorbents derived from polysaccharide-based biopolymers (i.e., cellulose, chitin, and chitosan) and their derivatives are profoundly significant in the area of HM ion absorption.7–9 Hydrogels extracted from such natural polymers are an intriguing class of three-dimensional (3D) biomaterials which undergo reversible volume changes in water.10 In addition to the high correlation between swelling and water permeability, the high water absorption capacity of such hydrogels allows more rapid ion penetration into the cross-linked mesh via chelation or ion exchange mechanisms.11–14
Gelatin and chitosan are among the promising biodegradable adsorbent materials applied for efficient HM removal from drinking water.15 Gelatin is a water-soluble biopolymer which is obtained as a degradation product of water-insoluble protein collagen and has the ability to remove HMs from drinking water.16,17 The distinctive advantages of nontoxicity, biodegradability, low cost and easy availability, and the presence of various functional groups (–OH, –NH2, and –COOH) that act as binding sites via polar or ionic interactions have made gelatin an effective bioadsorbent in wastewater treatment. Nonetheless, the non-covalent associations within the 3D gelatin network are easily broken in water over a mild temperature range (30–35 °C), consequently destroying the physical network. Such limitations could be overcome by establishing stable covalent amide bonds between carboxyl groups and amino groups of the gelatin backbone, via crosslinking. Similarly, chitosan is a deacylated product of chitin. Owing to its hydrophilicity, biocompatibility, biodegradability and non-toxicity, and the presence of reactive amino (–NH2) and hydroxyl (–OH) groups on its backbone, chitosan has been widely recommended for the recovery of toxic heavy metal ions from wastewater.18–20 However, due to its poor solubility, poor mechanical strength, low elasticity and pKa value (about 6.3–6.4), the application of chitosan is unsatisfactory. Interestingly, when crosslinked with ethylene glycol, diglycidyl ether, formaldehyde, glyoxal, epichlorohydrin, glutaraldehyde, isocyanates, and carbodiimides, both gelatin and chitosan polymers show an increased number of binding sites for metal ions.
In this context, a crosslinked gelatin–chitosan blend would be an ideal soft material to address both the limitations of low mechanical stability and actuation repeatability,21 and may also impart increased metal binding capacity. Improvisations have been made to introduce high mechanical strength in hydrogels by creating double-network-hydrogels (hydrogels based on two interpenetrating and cross-linked polymer networks).22–24 Hence, the idea of double-network-hydrogels may provide the opportunity to build a versatile category of adsorbents that are both mechanically strong and functionally active and repetitive.
In this study, we crosslinked natural polymers (gelatin and chitosan) to prepare a composite hydrogel which was finally transformed into irregular particles of various grade sizes for the adsorption of heavy metal ions. FTIR confirmed the crosslinking between gelatin and chitosan, whereas, SEM revealed the structural morphology of the hydrogel. Depending upon the drying procedure, the GC hydrogel attained offbeat features. One, the freeze-dried hydrogel behaved like a “sponge” with bigger air sacs, and the empty spaces were hydrated without driving any outward actuation. Second, the freeze-dried hydrogel, when placed in a vacuum overnight at 40 °C, was transformed into a compact “muscle”-like actuator with narrow open spaces/channels that display quick swelling/deswelling properties over multiple cycles in water at laboratory temperature. The adsorption of heavy metal ions on hydrogel particles was carried out over a 24 h time-scale. The degree of metal ion adsorption was initially tracked by the CRE25 and finally measured by ICP-MS. Adsorption of Hg(II) on GC hydrogel particles was found to be outstanding when measured separately as well as in a mixture of four heavy metal salts. This study presents a straightforward, robust, and eco-friendly method to detect and remove HM ions from the aqueous mixtures.
1. Materials and methods
1.1. Reagents
The heavy metal salts used in this experiment were lead(II) chloride (PbCl2), mercury(II) chloride (HgCl2), cadmium(II) chloride (CdCl2), and chromium(III) chloride (CrCl3). PbCl2, CdCl2, and CrCl3 were purchased from Samchun, Korea, whereas HgCl2 and glacial acetic acid were received from Duksan, South Korea. Chitosan was purchased from Daemyung Chemicals, South Korea (with 92% degree of deacetylation and an average molecular weight of ∼2000 kDa), whereas gelatin (porcine skin-type A) was received from Sigma Aldrich. The crosslinking agent glutaraldehyde (50% aqueous solution) was purchased from Daejung, South Korea. PDMS Sylgard 184 elastomer was purchased from Dow Corning (Midland, MI, USA).
1.2. Preparation of GC hydrogel particles
The composite GC hydrogel particles with a size range of ≤425 μm were used as adsorbents for metal ion binding. Chitosan (3 wt%) was dissolved in an aqueous mixture of 0.1 M acetic acid. The mixture was stirred overnight at laboratory temperature until chitosan was fully dissolved to yield a viscous, pale yellow solution. To this mixture, an aqueous solution of gelatin (10 wt%), dissolved in deionized (D.I.) water, was added at 40 °C. The reaction mixture was allowed to stir at 250 rpm for 2 h at laboratory temperature before the crosslinking agent, glutaraldehyde (1 wt%), was added dropwise. The reaction mixture underwent a quick (within 5–10 s) crosslinking process, which finally turned the reaction mixture from increasingly viscous to a hydrogel state. With increased viscosity, the movement of the magnetic stirrer first slowed down and then stopped completely with gel formation. At this point, the soft solid hydrogel material was taken out, crushed in a food grinder-mixer, and vortexed overnight to complete the crosslinking process, followed by washing the reaction mixture with D.I. water until a neutral pH value was achieved. The reaction mixture was washed to remove acetic acid and any excess amount of glutaraldehyde that may hinder the adsorption of HM ions. The reaction mixture was then freeze-dried for 72 h to obtain a porous GC hydrogel composite, characterized by FTIR. The freeze-dried hydrogel was ground again to make hydrogel particles of variable sizes. The particles were separated into different size grades (∼1000 μm, ∼710 μm, ∼425 μm, and ∼45 μm) on a vibrating sieve tray column. The scheme of the reaction is illustrated in Fig. 1a.
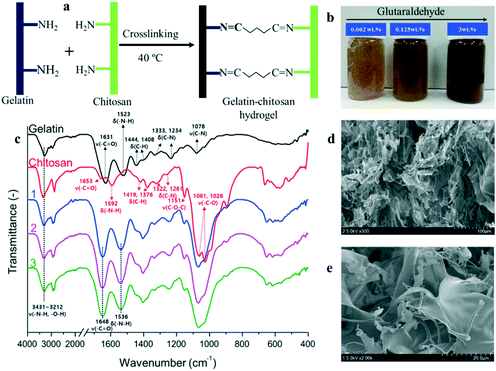 |
| Fig. 1 (a) Schematic illustration of the synthetic approach used to prepare the GC hydrogel, (b) hydrogels prepared at 0.062, 0.125, and 3 wt% of glutaraldehyde (50% aqueous solution), (c) FTIR spectra of gelatin, chitosan, and three different hydrogels, (d) SEM image of the dried hydrogel, and (e) high magnification image of the hydrogel depicted in panel (d). | |
1.3. Heavy metal adsorption test
The PDMS substrates employed to inspect the coffee rings of aqueous mixtures of heavy metal ions were fabricated by soft lithography. The PDMS prepolymer and cross-linking agent were thoroughly mixed at a weight ratio of 10
:
1 and then degassed in a vacuum chamber. Subsequently, the degassed PDMS mixture was poured onto a silicon wafer and cured at 65 °C for 4 h. After the curing process, PDMS stamps were cut and lifted off from the silicon wafer to examine the CRE of the aqueous mixtures.
The adsorption test was carried out by immersing GC hydrogel microparticles (≤425 μm size range) in a flask containing 100 mL of an aqueous mixture of HgCl2 (concentration = 1 g L−1). The reaction mixture was mildly (30 to 100 rpm) stirred for 24 h at laboratory temperature. The mixture was filtered through a syringe cellulose filter with a pore size of 0.2 μm. Small drops of the mixture were cast on a flat PDMS surface using a plastic syringe fixed with a needle (32 G). HM ion detection results extracted from the CRE were compared with the results obtained from ICP-MS. All the experiments were conducted under neutral conditions.
2. Results and discussion
2.1. Swelling/deswelling properties
The swelling behavior of the GC hydrogel was measured by immersing a small piece of dry gel in D.I. water for 24 h at laboratory temperature and neutral pH. The hydrogel underwent a significant volume change which was attributed to its porous morphology and the presence of hydrophilic functional groups on the blend. These two properties allow water to pass through the hydrogel blend via capillary forces. As a result, the water rushes into the hydrogel blend and creates an outward pressure to yield quick swelling—a perfect condition for metal ion adsorption. The volume increase continues until an equilibrium state of swelling is reached within 24 h. The following equation was employed to calculate the degree of swelling (DS):
where DS = degree of swelling, Ww = weight of the wet hydrogel, and Wd = weight of the dried hydrogel.
Depending upon the nature of the drying process, an excellent correlation was found between the DS and volume increase. A significant decrease in DS was recorded in the hydrogel dried under vacuum at ∼40 °C against the freeze-dried hydrogel of the same composition. In the case of the vacuum dried hydrogel, the DS was found to be in the range of 500–700%, and the swelling/deswelling was noticed to be significantly high, as observed by live visualization using an optical microscope with a mounted video camera (Fig. 2). Furthermore, the vacuum dried hydrogel shows remarkable quick shape-memory properties as the hydrogel retains its original shape without fatigue even after 25 rounds of quick swelling/deswelling in D.I. water.
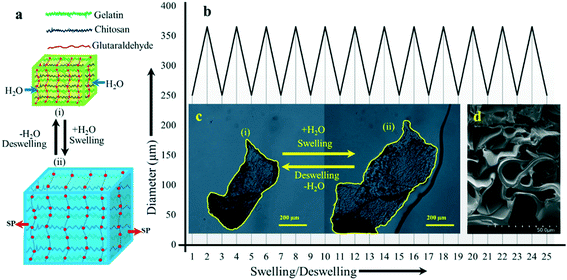 |
| Fig. 2 (a) Schematic illustration of swelling and deswelling of the GC hydrogel in dry and wet states at neutral pH and at laboratory temperature. (b) Multiple cycles of shape-memory between shrunk and swollen states in dry and hydrated states, respectively. (c) Corresponding optical image of the shrunk and swollen states in dry and hydrated states, respectively. (d) SEM cross-sectional image of the GC hydrogel dried overnight under vacuum at 40 °C. | |
In an alternative scenario, when the hydrogel was freeze-dried for 72 h, the DS was recorded to be between 7000–8000%, against the marginal outward volume increase (Fig. 3). The swelling/deswelling behavior was studied for 24 h, and the results were reproducible. We assume the difference in the reversible swelling/deswelling phenomena between two samples is due to the presence of large number of empty spaces in the freeze-dried blend, which were absent in the vacuum dried hydrogel, as demonstrated in Fig. 3. SEM (Fig. 3b and c) confirmed the presence of empty spaces in the freeze-dried hydrogel. The hydrogel under vacuum drying becomes highly coiled with fine micron-size open spaces or channels. When placed in contact with water, through hydrogen bonding, water rushes into the open spaces by capillary forces. These capillary forces generate an outward pressure called swelling pressure (SP) or stress to increase the hydrogel volume. However, during evaporation, the hydrogel collapses due to stress release and finally returns to its original shrunken state.
 |
| Fig. 3 (a) Schematic illustration of GC hydrogel swelling in water at neutral pH and laboratory temperature. (b) Top view SEM image of the freeze-dried GC blend. (c) Cross-sectional image of the hydrogel displayed in panel b. The hydrogel used here was freeze-dried over 72 h. | |
2.2. Tracking of HM ion adsorption by the coffee-ring effect (CRE)
To the best of our knowledge, less efficient methods are available to home-monitor the presence of HM ions in tap water at a cheaper cost. The early detection of toxic metals in tap water is essential to prevent long-term exposure. However, the current detection technologies do not achieve such end-results. For the detection of HM ions, ICP-MS is the only qualified method, as suggested by the Environmental Protection Agency (EPA). However, being localized in national laboratories, ICP-MS is both expensive and time-consuming to be installed for public usage. Efforts have been made to develop sensors that are suitable for home-monitoring.26–30 Nonetheless, there is still a strong urgency to develop HM detection systems that can be quick, cheap, and easy to operate for the general public. Recently, Rebecca Lahr and co-workers31 highlighted an efficiently quick and remarkably low-cost water quality home-monitoring technique based on the CRE. The group revealed that the general public could establish the hardness or the softness of tap water at a faster speed with cell phone-based technology. The technique may not necessarily have the sensitivity of the standard analytical technologies; however, being quick and cheaper, it may provide early signs of the problem that needs further analysis as reported by the author. Inspired by this study, we investigated the presence of Hg(II) ions in aqueous mixtures and also tracked the rate of adsorption on GC hydrogel particles by the CRE. After the synthesis of crosslinked hydrogel particles, the adsorption of Hg(II) on hydrogel particles was carried out over a 24 h time scale at laboratory temperature. The CRE first tracked the rate of adsorption on hydrogel particles at different time intervals and then compared the results with the data provided by ICP-MS. The correlated measurements confirmed a fine agreement in the rate of HM ion adsorption. The adsorption of HM ions on GC hydrogel particles was tested by using HgCl2 metal salt. Before the adsorption process, a drop of an aqueous mixture containing 1 g L−1 HgCl2 displays a perfect coffee ring pattern on a flat PDMS substrate with a thick rim structure. The rim thickness regularly decreased in time, making it effortless to predict the rate of reaction like in thin layer chromatography (TLC). Hence, we present a simple, fast and low-cost wastewater monitoring approach that has the potential to quickly monitor various undesired suspended or dissolved ingredients in wastewater before using complex and expensive techniques.
As detailed in Fig. 4a, when cast on a flat PDMS surface a drop of an aqueous mixture consisting of the heavy metal salt HgCl2 (1 g L−1) under evaporation crystallizes the dissolved salts at the periphery of the drop into a thick rim-like structure under the CRE. Under this effect, the non-volatile suspended particles or dissolved salts in a drop of a volatile solvent tend to concentrate around the droplet edges as the solvent evaporates. The CRE has been a familiar phenomenon since the discovery of this effect by Deegan and co-workers in 1997; the subject has been meticulously investigated, owing to its immense significance in patterning technology, biology, medical science, and wastewater research.32,33 The experimental procedure was started by dissolving HgCl2 in D.I. water at a concentration of 1 g L−1. A syringe filter (cellulose with a pore size of 0.2 μm) was used to filter the reaction mixture to remove any impurities. From the aqueous filtered mixture, a small drop was cast on a flat PDMS substrate fixed horizontally on a glass slide. This drop that is in contact with the PDMS surface undergoes evaporation at laboratory temperature. After complete evaporation, a fine coffee ring pattern was left behind on the PDMS surface. The amount of crystallized salt was found to be higher on the outer ring rim than in the center part of the pattern (Fig. 4b and d). The coffee ring pattern observed from the reaction mixture before the HM adsorption test was compared to the one taken after the test. We noticed that the pattern observed after the adsorption test was completely different from the one taken before. As shown in the optical microscopy images (Fig. 4c and e), two major changes are displayed: (a) the disappearance of the thick outer rim in the dried pattern, and (b) the occurrence of a very thin crystal pattern left behind in the middle part of the pattern. SEM observed a more evident structural difference in the two dried patterns (Fig. 4f and g). The disappearance of the coffee ring rim and the thinness of the crystallization in the pattern are a strong validation of the adsorption of HgCl2 on hydrogel particles.
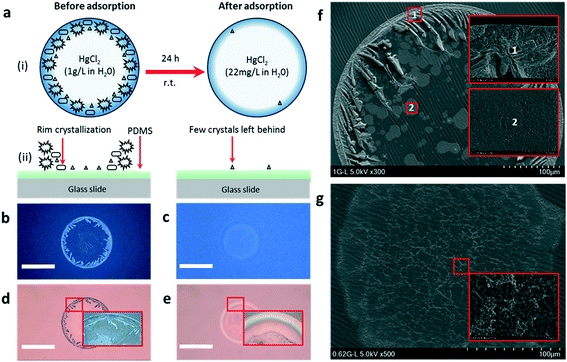 |
| Fig. 4 CRE based pattern as an indicator of HM ion adsorption on hydrogel particles in an evaporated aqueous mixture drop of D.I. water and HgCl2 salt (1 g L−1). (a) Schematic outline of the changes in the CRE in an evaporated drop of HgCl2 (1 g L−1) on a flat PDMS substrate before and after the HM ion adsorption: top view (i) and lateral view (ii). (b–e) Optical microscopy images of the pattern before (b and d: dark field images) and after (c and e: bright field images) the adsorption test. The insets in panels (d) and (e) represent the magnified images of the outer rim of the CRE pattern. The scale bar is 250 μm. (f) SEM image of the pattern before the adsorption test (insets 1 and 2 represent the outer rim and middle crystal formations, respectively). (g) SEM image of the pattern after the adsorption test (inset: magnified image of the leftover crystals). | |
The rate of HgCl2 adsorption on hydrogel particles was efficiently tracked by the coffee ring patterns on PDMS surfaces at different times such as 30 min, 5 h, 10 h, and 24 h, respectively. A perfect correlation between the CRE based patterns and the results obtained from ICP-MS was established. The detailed experimental results are shown in Fig. 5. To study the rate of reaction by the CRE, we first cast an aqueous drop of HgCl2 (745 mg L−1) on a flat PDMS substrate. The aqueous mixture was filtered with a syringe filter (cellulose with a pore size of 0.2 μm). Under evaporation at laboratory temperature, the drop left behind a perfect coffee ring structure with crystallized salt on the edges. Then we set up the adsorption test by mixing 1 g of GC hydrogel microparticles (with ≤425 μm size range) with 100 ml of an aqueous mixture of HgCl2 (745 mg L−1). The mixture was stirred at 30 to 100 rpm at laboratory temperature. After 30 minutes of reaction progress, stirring was stopped for a few minutes to allow the suspended hydrogel particles to settle down. This was followed by filtering a small amount of the reaction mixture by a syringe filter. The experimental procedure was repeated at 5 h, 10 h, and 24 h of the reaction progress. On casting the filtrate drops from the reaction mixture on a PDMS substrate, we observed a regular decrease in the rim width of the coffee ring structure from an evaporated drop. The rim thickness decreased abruptly in the first 30 minutes of the adsorption test. After obtaining the ICP-MS results, we found that 82% of HgCl2 ions were removed from the solution mixture in the first 30 min. Between 5 h and 24 h reaction time, slight changes were noticed in the dried pattern. When the adsorption test was carried out for 24 h, the coffee ring pattern of an evaporating drop almost disappeared. At 24 h, ICP-MS results revealed that 98% of HgCl2 metal ions were adsorbed on hydrogel particles. From these results, we extracted that the first 30 minutes of the adsorption test is significant, as 82% of the metal ions get adsorbed on the GC hydrogel particles in this period. As the adsorption test proceeds, a lower number of suspended metal ions are available in the reaction. When a drop from this reaction mixture is cast on a PDMS surface, the rim structure is not prominent. Having a variable rim structure at a different time interval of an adsorption test is a strong manifestation of the rate of a reaction. The rim diameter of the coffee ring regularly decreased with time, hence making it effortless to predict the rate of the reaction like in the thin-layer chromatography (TLC) technique. Additionally, to examine the HM salt concentration-based coffee ring patterns, we cast filtrate drops from the reaction mixture on flat PDMS substrates over a 24 h time scale at laboratory temperature; each time we reduced the concentration, we obtained a different coffee ring pattern (Fig. 5).
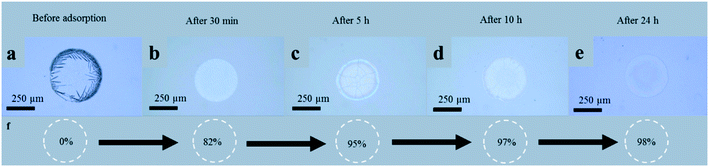 |
| Fig. 5 The rate of HM ion adsorption on hydrogel microparticles as a function of the CRE, over a 24 h time scale at laboratory temperature. The coffee ring pattern of an evaporated aqueous mixture drop of HgCl2 filtrate with an initial concentration of 745 mg L−1 cast on a PDMS surface before the adsorption test (a) and after 30 min (b), 5 h (c), 10 h (d), and 24 h (e) of the reaction progress, respectively. (f) Left to right: ICP-MS results presenting the percentage of HM ions adsorbed on the GC hydrogel at different time intervals (i.e., 82%, 95%, 97%, and 98% of HM ions were removed at 30 min, 5 h, 10 h, and 24 h, respectively). A syringe cellulose filter was used to filter all the mixtures used in casting the aqueous drops on PDMS surfaces with a pore size of 0.2 μm. The scale bar in panels a–e is 250 μm. | |
2.3. ICP-MS results
2.3.1. Individual heavy metal ion adsorption.
After synthesis, the GC hydrogel microparticles (with ∼425 μm size range) were immersed in a round-bottom flask containing an aqueous mixture of heavy metal ions (1 g L−1). The mixture was stirred at 30 to 100 rpm (at laboratory temperature) for 24 h. The reaction mixture was filtered through a syringe cellulose membrane with a pore size of 0.2 μm. The filtered water was collected and characterized by ICP-OES for metal ion detection. We studied the adsorption percentage of Pb(II), Hg(II), Cd(II), and Cr(III) metal ions under at pH and atmospheric temperature.
When tested individually, the experimental results revealed that 98% of the Hg(II) metal ions were removed from the water mixture against 34%, 20%, and 16% for Pb(II), Cd(II), and Cr(III), respectively. The experimental details are given in Table 1. Kd stands for the distribution coefficient which is the ratio of the concentration of the solute adsorbed on the soil or other sorbent divided by its concentration in solution. A 24 h Kd is the analogous ratio evaluated after 24 h of contact of the solute and sorbent. Kd can be calculated as follows:
where
A is the initial concentration of the solute defined as the mean concentration of the blanks (μg mL
−1),
B is the final concentration of the solute after 24 h in contact with the sorbent (μg mL
−1),
V is the volume of the solution used (mL),
Ms is the mass of the sorbent expressed on an oven-dried basis (g), and
Kd is the distribution coefficient (L g
−1).
Table 1 Individual metal ion adsorption and respective Kd values
Sample number |
Heavy metal salts |
Hydrogel adsorbent (g) |
Heavy metal solution (ppm) |
Adsorption of metal ions (ppm) |
K
d (L g−1) |
1 |
PbCl2 |
1 |
802 |
272 |
0.0513 |
2 |
HgCl2 |
1 |
824 |
811 |
6.24 |
3 |
CdCl2 |
1 |
997 |
101 |
0.0113 |
4 |
CrCl3 |
1 |
184 |
30 |
0.0195 |
2.3.2. Selective heavy metal ion adsorption.
Usually, wastewater discharge from industries contains multiple metal ions. Hence it has become vital to study the sorption phenomenon in a mixture containing various metallic ingredients. The selective adsorption of four metal ions (Pb(II), Cd(II), Hg(II), and Cr(III)) onto the hydrogel particles was investigated, and it was found that 97% of the Hg(II) metal ions were removed from the mixture against 24%, 12% and 2% for Cr(III), Pb(II), and Cd(II), respectively. The reason for the higher and selective adsorption of Hg(II) on the hydrogel network can be attributed to the higher affinity of Hg(II) towards active functional groups, especially functionalities with nitrogen and sulfur atoms.34 This chelation behaviour of Hg(II) can be supported by ‘hard soft acid base (HSAB)’ theory that classifies Hg(II) as a soft ion with strong binding affinity with –NH2, –CN, and –SH groups. The lone pair availability in these groups makes the metal-complex formation with Hg(II) significantly feasible. The reported composite GC hydrogel has sufficient reactive sites available in the network which account for the higher binding capability to Hg(II) for its extraction from the mixture (Table 2).35
Table 2 Selective metal ion adsorption and respective Kd values
Heavy metal salts |
Hydrogel adsorbent (g) |
Heavy metal solution (ppm) |
Adsorption of metal ions (ppm) |
K
d (L g−1) |
Mixture |
1 |
Pb(II) 327 |
Pb(II) 39 |
0.0135 |
Hg(II) 349 |
Hg(II) 338 |
3.07 |
Cd(II) 254 |
Cd(II) 5 |
0.00201 |
Cr(III) 88 |
Cr(III) 21 |
0.0313 |
3. Conclusion
In summary, we synthesized a composite GC hydrogel blend that exhibited prominent swelling/deswelling characteristics in water and dry state respectively. Two different drying processes tuned the volume changes of the hydrogel in water. The vacuum dried hydrogel displayed quick and repeatable volume actuation in D.I. water akin to shape memory, which we assume was due to the highly coiled structure of the blend. However, the behavior was significantly reduced in the freeze-dried hydrogel. Also, the hydrogel particles were examined for the adsorption of metal ions (PbCl2, HgCl2 CdCl2, and CrCl3) at neutral pH and laboratory temperature. The chemical and the structural morphology of the synthesized hydrogel was confirmed by FTIR and SEM, respectively. CRE and then ICP-MS characterized the HM ion adsorption test. It is worth pointing out that a perfect correlation was found between the results obtained from CRE and ICP-MS. As compared to Pb(II), Cd(II), and Cr(III) metal ions, hydrogel particles displayed notable binding affinity towards Hg(II). Furthermore, the hydrogel particles were elucidated for the selective adsorption of HMs. The GC hydrogel blend reported in this work was easily synthesized, the hydrogel microparticles were prepared via mass fabricating approach, and the unique way of monitoring the Hg(II) adsorption on hydrogel particles by CRE makes this study an important update in heavy metal ion removal from aqueous mixtures by cost-effective green adsorbents.
Conflicts of interest
There are no conflicts to declare.
Acknowledgements
The authors are very grateful to the Ministry of Trade, Industry & Energy of the Republic of Korea for providing financial support (Grant No. 10070241).
References
- X. W. Peng, L. X. Zhong, J. L. Ren and R. C. Sun, Highly effective adsorption of heavy metal ions from aqueous solutions by macroporous xylan-rich hemicelluloses-based hydrogel, J. Agric. Food Chem., 2012, 60, 3909–3916 CrossRef CAS PubMed.
- L. Fan, C. Luo, X. Li, F. Lu, H. Qiu and M. Sun, Fabrication of novel magnetic chitosan grafted with graphene oxide to enhance adsorption properties for methyl blue, J. Hazard. Mater., 2012, 215–216, 272–279 CrossRef CAS PubMed.
- Q. Y. Chen, Z. Luo, C. Hills, G. Xue and M. Tyrer, Precipitation of heavy metals from wastewater using simulated flue gas: sequent additions of fly ash, lime and carbon dioxide, Water Res., 2009, 43, 2605–2614 CrossRef CAS PubMed.
- G. X. Yang and H. Jiang, Amino modification of biochar for enhanced adsorption of copper ions from synthetic wastewater, Water Res., 2014, 48, 396–405 CrossRef CAS PubMed.
- L. T. T. Cao, H. Kodera, K. Abe, H. Imachi, Y. Aoi, T. Kindaichi, T. Ozaki and A. Ohashi, Biological oxidation of Mn(II) coupled with nitrification for removal and recovery of minor metals by downflow hanging sponge reactor, Water Res., 2015, 68, 545–553 CrossRef CAS PubMed.
- Z. W. Thong, G. Han, Y. Cui, J. Gao, T. S. Chung, S. Y. Chan and S. Wei, Novel nanofiltration membranes consisting of a sulfonated pentablock copolymer rejection layer for heavy metal removal, Environ. Sci. Technol., 2014, 48, 13880–13887 CrossRef CAS PubMed.
-
A. Isogai, Material Science of Cellulose, University of Tokyo Press, Tokyo, 2001 Search PubMed.
- M. N. V. R. Kumar, A review of chitin and chitosan applications, React. Funct. Polym., 2000, 46, 1–27 CrossRef CAS.
- G. P. Martin, Applications and Environmental Aspects of Chitin and Chitosan, J. Macromol. Sci., Part A: Pure Appl. Chem., 1995, 32, 629–640 Search PubMed.
- P. Calvert, Hydrogels for Soft Machines, Adv. Mater., 2009, 21, 743–756 CrossRef CAS.
- D. Seliktar, Designing cell-compatible hydrogels for biomedical applications, Science, 2012, 336, 1124–1128 CrossRef CAS PubMed.
- G. Zhou, C. Liu, L. Chu, Y. Tang and S. Luo, Rapid and efficient treatment of wastewater with high-concentration heavy metals using a new type of hydrogel-based adsorption process, Bioresour. Technol., 2016, 219, 451–457 CrossRef CAS PubMed.
- G. Zhou, J. Luo, C. Liu, L. Chu and J. Crittenden, Efficient heavy metal removal from industrial melting effluent using fixed-bed process based on porous hydrogel adsorbents, Water Res., 2018, 131, 246–254 CrossRef CAS PubMed.
- G. Zhou, J. Luo, C. Liu, L. Chu, J. Ma, Y. Tang, Z. Zeng and S. Luo, A highly efficient polyampholyte hydrogel sorbent based fixed-bed process for heavy metal removal in actual industrial effluent, Water Res., 2016, 89, 151–160 CrossRef CAS PubMed.
- W. Jiang, W. Wang, B. Pan, Q. Zhang, W. Zhang and L. Lv, Facile fabrication of magnetic chitosan beads of fast kinetics and high capacity for copper removal, ACS Appl. Mater. Interfaces, 2014, 6, 3421–3426 CrossRef CAS PubMed.
- X. Bi, R. J. Lau and K.-L. Yang, Preparation of ion-imprinted silica gels functionalized with glycine, diglycine, and triglycine and their adsorption properties for copper ions, Langmuir, 2007, 23, 8079–8086 CrossRef CAS PubMed.
- G. Chen, C. Qiao, Y. Wang and J. Yao, Synthesis of magnetic gelatin and its adsorption property for Cr(VI), Ind. Eng. Chem. Res., 2014, 53, 15576–15581 CrossRef CAS.
- Y. Suneetha, B. N. Kumar, Y. Harinath, D. H. K. Reddy and K. Seshaiah, Functionalization of Cross Linked Chitosan with 2-Aminopyridine-3-carboxylic Acid for Solid Phase Extraction of Cadmium and Zinc Ions and Their Determination by Atomic Absorption Spectrometry, Microchim. Acta, 2012, 176, 169–176 CrossRef CAS.
- M. R. Gandhi and S. Meenakshi, Preparation and Characterization of Silica Gel/Chitosan Composite for the Removal of Cu(II) and Pb(II), Int. J. Biol. Macromol., 2012, 50, 650 CrossRef PubMed.
- L. L. Fan, C. N. Luo, Z. Lv, F. G. Lu and H. M. Qiu, Removal of Ag+ from Water Environment Using a Novel Magnetic Thiourea-Chitosan Imprinted Ag, J. Hazard. Mater., 2011, 194, 193–201 CrossRef CAS PubMed.
- J. T. Sun, X. H. Zhao, W. R. K. Illeperuma, O. Chaudhuri, K. H. Oh, D. J. Mooney, J. J. Vlassak and Z. G. Suo, Highly stretchable and tough hydrogels, Nature, 2012, 489, 133–136 CrossRef CAS PubMed.
- Y. Y. Zhang, Y. M. Li and W. G. Liu, Dipole-Dipole and H-Bonding Interactions Significantly Enhance the Multifaceted Mechanical Properties of Thermoresponsive Shape Memory Hydrogels, Adv. Funct. Mater., 2015, 25, 471–480 CrossRef CAS.
- Q. Chen, L. Zhu, L. N. Huang, H. Chen, K. Xu, Y. Tan, P. X. Wang and J. Zheng, Fracture of the Physically Cross-Linked First Network in Hybrid Double Network Hydrogels, Macromolecules, 2014, 47, 2140–2148 CrossRef CAS.
- J. Ma, G. Zhou, L. Chu and Y. Liu, Efficient Removal of Heavy Metal Ions with an EDTA Functionalized Chitosan/Polyacrylamide Double Network Hydrogel, ACS Sustainable Chem. Eng., 2017, 5, 843–851 CrossRef CAS.
- R. D. Deegan, O. Bakajin, T. F. Dupont, G. Huber, S. R. Nagel and T. A. Witten, Capillary flow as the cause of ring stains from dried liquid drops, Nature, 1997, 389, 827–829 CrossRef CAS.
- S. Laschi, I. Palchetti and M. Mascini, Gold-based screen-printed sensor for detection of trace lead, Sens. Actuators, B, 2006, 114, 460–465 CrossRef CAS.
- J. Wang and B. Tian, Mercury-free Disposable Lead Sensors Based on Potentiometric Stripping Analysis of Gold-coated Screen-printed Electrodes, Anal. Chem., 1993, 65, 1529–1532 CrossRef CAS PubMed.
- M. Guziński, G. Lisak, J. Kupis, A. Jasiński and M. Bocheńska, Lead(II)-selective ionophores for ion-selective electrodes: a review, Anal. Chim. Acta, 2013, 791, 1–12 CrossRef PubMed.
- M.-R. Huang, Y.-B. Ding and G. Li, Combinatorial Screening of Potentiometric Pb(II) Sensors from Polysulfoaminoanthraquinone Solid Ionophore, ACS Comb. Sci., 2014, 16, 128–138 CrossRef CAS PubMed.
- Y.-L. Hung, T.-M. Hsiung, Y.-Y. Chen and C.-C. Huang, A label-free colorimetric detection of lead ions by controlling the ligand shells of gold nanoparticles, Talanta, 2010, 82, 516–522 CrossRef CAS PubMed.
-
L. Rebecca, 254th National Meeting & Exposition of the American Chemical Society (ACS), Published on Aug 22, 2017 Search PubMed.
- R. D. Deegan, Pattern formation in drying drops, Phys. Rev. E: Stat. Phys., Plasmas, Fluids, Relat. Interdiscip. Top., 2000, 61, 475–485 CrossRef CAS.
- R. D. Deegan, O. Bakajin, T. F. Dupont, G. Huber, S. R. Nagel and T. A. Witten, Contact line deposits in an evaporating drop, Phys. Rev. E: Stat. Phys., Plasmas, Fluids, Relat. Interdiscip. Top., 2000, 62, 756–765 CrossRef CAS.
- C. Jeon and K. H. Park, Adsorption and desorption characteristics of mercury(II) ions using aminated chitosan bead, Water Res., 2005, 39, 3938–3944 CrossRef CAS PubMed.
- X. Wang, W. Deng, Y. Xie and C. Wang, Selective removal of mercury ions using a chitosan–poly(vinyl alcohol) hydrogel adsorbent with three-dimensional network structure, Chem. Eng. J., 2013, 228, 232–242 CrossRef CAS.
|
This journal is © The Royal Society of Chemistry 2019 |
Click here to see how this site uses Cookies. View our privacy policy here.