UVA-induced neurotoxicity in Japanese medaka (Oryzias latipes)
Received
20th April 2018
, Accepted 26th September 2018
First published on 27th September 2018
Abstract
Ultraviolet radiation-induced neurodegeneration has been studied in the early stages of development in fish, but not extensively in the adult stage. The present study aimed at investigating the effects of ultraviolet radiation-A (UVA) in adult Japanese medaka (Oryzias latipes). The brain, spinal cord, and retina were examined histopathologically as nervous system target organs. Japanese medaka fish were exposed to 15, 30, and 60 min day−1 UVA for 3 days, and samples were obtained 24 h and 14 days after UVA exposure. Neurohistopathological alterations in brain tissue included vacuoles, blood congestion, degeneration of neuropils, and pyknotic nuclei in neurons. Alterations in the spinal cord included neuronal cell degeneration, reduction in the spinal cord area, and degeneration of Mauthner cells. Retinal tissue showed vacuolation in the nerve fiber layer (NFL), pyknotic nuclei in the ganglion cell layer (GCL), and decreased cell populations particularly in the inner nuclear layer (INL) and GCL. The degree of degeneration was dependent on the duration of UVA exposure. The signs of degeneration decreased gradually and disappeared completely after the 14-day recovery period. In addition, p53-deficient medaka fish were more tolerant than were wild-type (Hd-rR) Japanese medaka. In conclusion, UV radiation induced neurodegeneration in the brain, spinal cord, and retina of adult Japanese medaka (Oryzias latipes) but their normal histological architecture reappeared in these tissues after 14 days.
1. Introduction
The damaging effects of ultraviolet radiation-A (UVA) have been reported in fishes and frogs, and include hemotoxic1–4 and embryotoxic effects,5–7 enzymatic disruption,8 and DNA damage.8,9 UVA radiation also has severe histopathological effects on the early life stages of the northern anchovy (Engraulis mordax(, Pacific mackerel (Scomber japonicus), and crayfish. These effects include lesions in the brain and retina,10,11 discontinuous pigmented epithelium, and degeneration of the lens, retinal layers, gray matter, and central canal of the spinal cord.5 Generally, the brain and spinal cord play an important role in fish physiology and the brain tissue is of interest in fish toxicology. It is thought that brain biomarkers may be useful for pollution biomonitoring and may provide warning signals about pollutant exposure.12 The retina is a complex organized structure at the back of the eye, and comprises three nuclear neuronal layers and two synaptic layers. Several factors affect the susceptibility of the fish retina to light damage, including eye pigmentation, the rod/cone ratio, and the age of the animal.13
Histopathological investigations in laboratory experiments provide a sensitive tool for detecting the direct effects of chemical compounds within the target organs of fish. Histopathological biomarkers appear as tissue lesions arising from previous or current exposure of the organism to one or more toxins.14 The tumor suppressor protein, p53, is a DNA-binding transcription factor encoded by Trp53. This protein induces apoptosis and cell cycle arrest in response to various genotoxic stresses, which blocks the transmission of induced DNA mutations to progeny cells.15 Several studies indicate that the loss of p53 function increases cell proliferation as part of the self-renewal of neural stem cells (NSCs) in the adult murine brain; this observation suggests that p53 acts to suppress neurogenesis among these stem cells.16–18
Medaka has been used as a research organism in toxicological studies. Such studies have used adults, embryos, and early larval stages to identify the indicators of aquatic toxicology.19,20 The purposes of our study were to first investigate the effects of UVA irradiation on the histology of the brain, spinal cord, and retina of medaka, and to study the sensitivity of wild-type (WT) (Hd-rR) and p53-deficient (−/−) medaka to UVA irradiation.
2. Materials and methods
2.1. Medaka fish
Adult medaka female fish with mature ovaries (O. latipes), WT (Hd-rR) and homogeneic p53-deficient medaka originally made by the TILLING mutation were kept before treatment at 26–28 °C with a 14 h light/10 h dark photoperiod cycle to reduce circadian variation. The fish were fed three times per day (5% body weight), and the feeding regime used a formulated diet (Tetra-min, Tetra Werke Co., Melle, Germany) and brine shrimp (Artemia franciscana).
2.2. Experimental design
Medaka fish were kept in separate tanks under laboratory conditions. Each strain was divided into four experimental groups, and the groups were physically and visually isolated from each other to avoid cross-contamination of UV irradiation. The UVA doses were chosen consistent with Sayed et al.19 as follows: group 1; control fish, kept for 3 days without UVA exposure; group 2, UVA-exposed fish exposed daily to UVA for 15 min day−1 for 3 days; group 3, UVA-exposed fish exposed daily to UVA for 30 min day−1 for 3 days; and group 4, UVA-exposed fish exposed daily to UVA for 60 min day−1 for 3 days.
Three replicates were used for each treatment group. The laboratory conditions of acclimation remained stable during UVA exposure, which was performed at the same time of the day for 3 days. Tissue samples were obtained 24 h and 14 days after UVA exposure.
2.3. Irradiation with UVA
Irradiation was performed using a UV lamp with a wavelength of 366 nm (UVL-56, Ultraviolet products, Inc. San Gabriel, CA, USA). The fish were placed in an experimental plastic container incorporated with a UV lamp placed 8 cm above the bottom of the container according to the procedure described in Sayed et al.19 The dose rate at this level was 2450 μW cm−2 as measured using a UV meter (UVPF-A [PD-365]), Zhi Cai Chemical Co., Ltd Kunshan, China. At the two sampling times after UVA exposure, three replicates for each group (two samples from each replicate) were prepared for examination.
2.4. Ethics statement
All experiments and protocols were approved (Permit Number: C-14-02) by the Committee on the Ethics of Animal Experiments of The University of Tokyo.
2.4. Histological examination
Six fish per treatment at the sampling time were sacrificed using ice-cold water.21 The entire body was fixed overnight in Davidson's solution, and the fixed tissues were removed, dehydrated, and embedded in paraffin. Serial sections of 7 μm thickness were cut using a microtome (Leica Microsystems, Modell RM2125, Germany) for the whole body and then stained with hematoxylin and eosin. The samples were examined under a digital microscope (BX50; Olympus, Japan) fitted with a digital color video camera (DP-70; Olympus, Japan).
3. Results
3.1. Brain (telencephalon) histopathology
The telencephalon of the control fish showed a normal structure. The telencephalon neurons comprise a cell body containing large central nuclei with a prominent nucleolus (Fig. 1a). After 15 min UVA exposure, the brain of WT showed vacuoles, blood congestion, and pyknotic nuclei (Fig. 1b), whereas the brain of p53-deficient fish showed only minor degeneration (Fig. 2b). After 30 min UVA exposure, the brain of WT fish showed degeneration of the neuropil, blood congestion, and pyknotic nuclei (Fig. 1d); by contrast, the brain of p53-deficient fish showed minor vacuolation and blood congestion (Fig. 2d). After 60 min UVA exposure, the brain of WT fish exhibited obvious degeneration of the neuropil, blood congestion, and pyknotic nuclei (Fig. 1f), but the brain of p53-deficient fish exhibited only some degeneration and blood congestion (Fig. 2f). After the 14-day recovery period after UVA exposure, the architecture of the brain appeared to be normal in all groups of both WT and p53-deficient fish.
 |
| Fig. 1 Brain from WT medaka fish (a) control, (b) 24 h after 15 min UVA exposure, (c) 14 days after 15 min UVA exposure, (d) 24 h after 30 min UVA exposure, (e) 14 days after 30 min UVA exposure, (f) 24 h after 60 min UVA exposure, and (g) 14 days after 60 min UVA exposure. Cong, blood congestion; V, vacuoles; Pyk, pyknotic nuclei; and Deg, degeneration of neuropil. H and E stain. | |
 |
| Fig. 2 Brain from p53-deficient fish (a) control, (b) 24 h after 15 min UVA exposure (c), 14 days after 15 min UVA exposure, (d) 24 h after 30 min UVA exposure, (e) 14 days after 30 min UVA exposure, (f) 24 h after 60 min UVA exposure, and (g) 14 days after 60 min UVA exposure. Cong, blood congestion; V, vacuoles; Pyk, pyknotic nuclei; and Deg, degeneration of neuropil. H and E stain. | |
3.2. Spinal cord histopathology
The spinal cord of the control fish comprises the outer sheath surrounding the white matter and gray matter. The gray matter of the spinal cord is divided into a dorsal horn and two ventral horns (which give the gray substance the shape of an inverted Y), which are connected and surround the central canal lined by ependymal cells. The gray matter is mainly composed of somata of neurons, dendrites, and glial cells. The white matter lies between these horns and is best exemplified by the two presumptive Mauthner fibers found ventral to the central canal.
After 15 min UVA exposure, the spinal cord in both WT and p53-deficient fish showed a reduction in the spinal cord area and degeneration of Mauthner cells (Fig. 3b and 4b). After 30 min UVA exposure, the spinal cord of WT fish showed less degeneration of Mauthner cells (Fig. 3d) compared with the spinal cord of p53-deficient fish, which showed greater degeneration of Mauthner cells (Fig. 4d). After 60 min UVA exposure, the spinal cord of WT fish showed a reduced spinal cord area and neuronal cell degeneration (Fig. 4f), and the spinal cord brain of p53-deficient fish showed Mauthner cell degeneration (Fig. 5f). After the 14-day recovery period, the architecture of the spinal cord had recovered in all groups for both WT and p53-deficient fish.
 |
| Fig. 3 Spinal cord from WT medaka fish (a) control, (b) 24 h after 15 min UVA exposure (c), 14 days after 15 UVA exposure, (d) 24 h after 30 min UVA exposure, (e) 14 days after 30 min UVA exposure, (f) 24 h after 60 min UVA exposure, and (g) 14 days after 60 min UVA exposure. GM, gray matter; WM, white matter; C, central canal; M, Mauthner cells ↔, reduction in the spinal cord area; and M deg, degeneration of Mauthner cells. H and E stain. | |
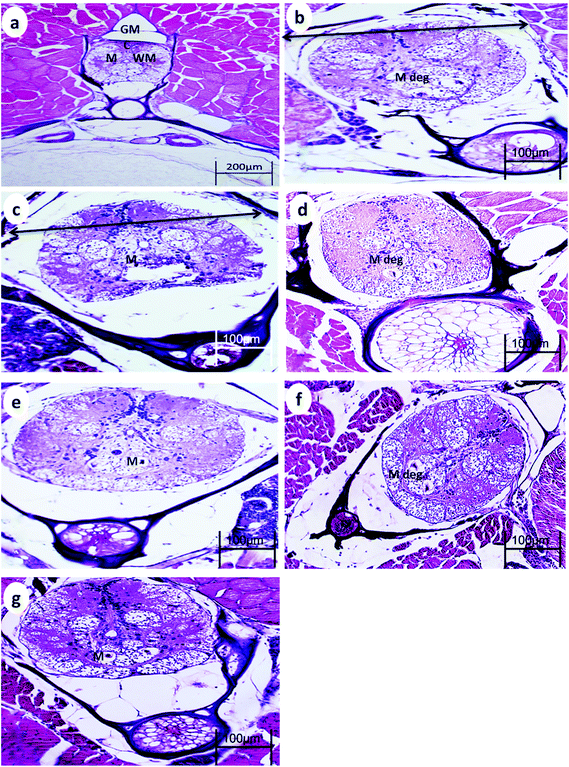 |
| Fig. 4 Spinal cord from p53-deficient fish (a) control, (b) 24 h after 15 min UVA exposure, (c) 14 days after 15 min UVA exposure, (d) 24 h after 30 min UVA exposure, (e) 14 days after 30 min UVA exposure, (f) 24 h after 60 min UVA exposure, and (g) 14 days after 60 min UVA exposure. GM, gray matter; WM, white matter; C, central canal; M, Mauthner cells ↔, reduction in the spinal cord area; and M deg, degeneration of Mauthner cells. H and E stain. | |
 |
| Fig. 5 Retina from WT medaka fish (a) control, (b) 24 h after 15 min UVA exposure, (c) 14 days after 15 min UVA exposure, (d) 24 h after 30 min UVA exposure, (e) 14 days after 30 min UVA exposure, (f) 24 h after 60 min UVA exposure, and (g) 14 days after 60 min UVA exposure. NFL, nerve fiber layer; GCL, ganglion cell layer; IPL, inner plexiform layer; INL, inner nuclear layer; OPL, outer plexiform layer; ONL, outer nuclear layer; R&C, layer of rods and cones; PE, pigment epithelium, →, decreased cellular populations particularly in the GCL★, decreased cellular populations particularly in the INL; V, vacuoles; and Pyk, pyknotic nuclei of GCL. H and E stain. | |
3.3. Eye retinal histopathology
Control retinas displayed normal retinal layering, and the three nuclear layers were intact: ganglion cell layer (GCL), inner nuclear layer (INL), and outer nuclear layer (ONL), as were the synaptic layers (inner- and outer plexiform layers (IPL and OPL, respectively)). There were no obvious signs of toxic insults to the tissue or cells.
After 15 min UVA exposure, the retina in WT medaka exhibited vacuolation in the nerve fiber layer (NFL), pyknotic nuclei in the GCL, and decreased cell populations particularly in the INL and GCL (Fig. 5b). The retina of p53-deficient fish showed decreased cell populations in the GCL (Fig. 6b).
 |
| Fig. 6 Retina from p53-deficient fish (a) control, (b) 24 h after 15 min UVA exposure, (c) 14 days after 15 min UVA exposure, (d) 24 h after 30 min UVA exposure, (e) 14 days after 30 min UVA exposure, (f) 24 h after 60 min UVA exposure, and (g) 14 days after 60 min UVA exposure. NFL, nerve fiber layer; GCL, ganglion cell layer; IPL, inner plexiform layer; INL, inner nuclear layer; OPL, outer plexiform layer; ONL, outer nuclear layer; R&C, layer of rods and cones; PE, pigment epithelium; →, decreased cellular populations particularly in the GCL★; decreased cellular populations particularly in the INL; V, vacuoles; and Pyk, pyknotic nuclei of GCL. H and E stain. | |
After 30 min UVA exposure, the retina in WT medaka showed decreased populations particularly in the GCL in both WT and p53-deficient fish (Fig. 5d and 6d). After 60 min UVA exposure, the retina in WT fish showed vacuolation in the NFL and decreased cell populations, particularly in the INL, ONL, and GCL (Fig. 5f), and the retina in p53-deficient fish showed decreased cell populations particularly in the GCL (Fig. 6f). Partial remediation of the architecture of the retina was observed in all groups in both WT and p53-deficient fish.
4. Discussion
There are few reports in the literature of the changes associated with the histology of nervous tissue and sense organs caused by exposure to UV light. In the present study, histopathological analysis showed vacuoles, blood congestion, degeneration of the neuropils, and pyknotic nuclei in the neurons in the brain, and neuronal cell degeneration, reduction in the spinal cord area, and degeneration of the Mauthner cells in the spinal cord. The retina exhibited vacuolation in the NFL, pyknotic nuclei in the GCL, and decreased cell populations particularly in the INL and GCL. The degree of degeneration was dependent on the duration of UVA exposure. Degeneration decreased gradually and disappeared completely after a 14-day recovery period.
The histopathological changes observed after exposure to UV in our study are similar to those reported by Hunter et al.,11 who reported UV-induced lesions in the brain and retina. Mahmoud et al.5 observed discontinuous pigmented epithelium, degenerated lens and retinal layers and degeneration of gray matter and the central canal of the spinal cord. Brain hemorrhaging has also been reported in UVB-exposed larval medaka.22 Exposure of rat retinal segments to UVB selectively damages the photoreceptor cells (causing pyknotic nuclei) and caused the disappearance of the outer segments of photoreceptor cells. This damage was more pronounced over several hours after cessation of UVB exposure.23 Constant intense light exposure of albino zebrafish causes widespread photoreceptor cell death,24–29 rod outer segment degradation (solar pruning), and photoreceptor loss in the albino rainbow trout30–32 and albino Oscar (Astronotus ocellatus).31 Bejarano-Escobar et al.13 reported that the retina exhibits abundant photoreceptor cell apoptosis during exposure to intense light in trench larvae, but not in juveniles. Damage to the retinal layers has been reported in photoreceptor cells, pigmented layer, and undifferentiated portions at the lateral sides of the retina in tadpoles (Egyptian toad; Bufo regularis).7.Vacuolation in the surrounding neuropil might be attributed to the shrinkage of cells and the withdrawal of their processes secondary to cytoskeletal disruption, which may leave pericellular spaces,33 or to the presence of vacuoles remaining from the phagosomes of microglia clustering in apoptotic neuronal cells.34 p53-deficient embryos were more tolerant than were WT (Hd-rR) Japanese medaka. This is consistent with the earlier findings of Sayed et al.19 and Yasuda et al.35
There is accumulating evidence that p53 might suppress the self-renewal of NSCs in the adult murine brain;16,17 if so, this suggests that p53 negatively regulates tissue self-renewal in adult tissues. The present results seem to be consistent with these reports, although p53 might also play a role in promoting tissue self-renewal through its complex functions in cell cycle regulation, as reported by Isoe et al.,36 who noted that p53 positively regulates neurogenesis in the adult medaka brain. By contrast, Yasuda et al.35 reported that self-renewal of brain tissue was completed faster at the time of hatching in p53-deficent fish compared to that in WT fish because p53 reduces the acute severe neural apoptosis induced by irradiation. This finding suggests that p53 is not essential for tissue self-renewal in the developing brain. This is explained by the fact that fish are capable of regenerating a variety of complex organs through epimorphosis, such as damaged retina, severed spinal cord, injured brain and heart, and amputated fins.37
In conclusion, the present work shows that UVA radiation induces histological alterations in the brain, spinal cord, and eye of both p53-deficient and WT medaka. The neurohistopathological alterations included vacuoles, blood congestion, degeneration of the neuropil, and pyknotic nuclei of neurons in brain tissue and neuronal cell degeneration, reduction in spinal cord area, and degeneration of Mauthner cells in the spinal cord. The retina exhibited vacuolation in the NFL, pyknotic nuclei in the GCL, and decreased cell populations particularly in the INL and GCL. The degree of degeneration was dependent on the duration of UVA exposure. p53-Deficient fish displayed fewer alterations than did WT medaka, which suggests that UVA exposure stimulates p53-dependent histological alterations.
Conflicts of interest
We declare no conflicts of interest.
Acknowledgements
This research was supported in part by the Japan Society for the Promotion of Science (FY2015 JSPS postdoctoral fellowship for overseas researchers) to Alaa El-Din H. Sayed (ID No. P15382).
References
- A. H. Sayed, A. T. Ibrahim, I. A. A. Mekkawy and U. M. Mahmoud, J. Photochem. Photobiol., B, 2007, 89, 170–174 CrossRef CAS PubMed.
- A. H. Sayed, H. S. Abdel-Tawab, S. S. Abdel Hakeem and I. A. Mekkawy, J. Photochem. Photobiol., B, 2013, 119, 9–14 CrossRef CAS PubMed.
- A. G. M. Osman, M. Koutb and A. H. Sayed, J. Photochem. Photobiol., B, 2010, 99, 1–8 CrossRef CAS PubMed.
- A. H. Sayed, Photochem. Photobiol., 2018, 94, 158–164 CrossRef CAS PubMed.
- U. M. Mahmoud, I. A. A. Mekkawy and A. H. Sayed, J. Photochem. Photobiol., B, 2009, 95, 117–128 CrossRef CAS PubMed.
- A. H. Sayed and H. Mitani, J. Photochem. Photobiol., B, 2016, 164, 132–140 CrossRef CAS PubMed.
- A. H. Sayed, E. T. Wassif and A. I. Elballouz, J. Radiat. Res. Appl. Sci., 2016, 9, 409–423 CrossRef.
- I. A. A. Mekkawy, U. M. Mahmoud, A. G. Osman and A. H. Sayed, Fish Physiol. Biochem., 2010, 36, 605–626 CrossRef CAS PubMed.
- A. H. Sayed and H. Mitani, J. Photochem. Photobiol., B, 2017, 171, 90–95 CrossRef CAS PubMed.
- Z. A. El-Bakary and A. H. Sayed, Ecotoxicol. Environ. Saf., 2011, 74, 960–966 CrossRef CAS PubMed.
- J. R. Hunter, J. H. Taylor and H. G. Moser, Photochem. Photobiol., 1979, 29, 325–338 CrossRef CAS PubMed.
- M. D. Ferrando and E. Andreu-Moliner, Bull. Environ. Contam. Toxicol., 1991, 47, 459–464 CrossRef CAS PubMed.
- R. Bejarano-Escobar, M. Blasco, G. Martin-Partido and J. Francisco-Morcillo, J. Exp. Biol., 2012, 215, 3799–3812 CrossRef PubMed.
- M. T. J. Bose, M. Ilavazhahan, R. Tamilselvi and M. Viswanathan, Biomed. Pharmacol. J., 2013, 6, 99–105 CrossRef CAS.
- C. J. Sherr and J. M. Roberts, Genes Dev., 1995, 9, 1149–1163 CrossRef CAS PubMed.
- K. Meletis, V. Wirta, S. M. Hede, M. Nister, J. Lundeberg and J. Frisen, Development, 2006, 133, 363–369 CrossRef CAS PubMed.
- W. B. Jacobs, G. Govoni, D. Ho, J. K. Atwal, F. Barnabe-Heider, W. M. Keyes, A. A. Mills, F. D. Miller and D. R. Kaplan, Neuron, 2005, 48, 743–756 CrossRef CAS PubMed.
- S. Gil-Perotin, J. D. Haines, J. Kaur, M. Marin-Husstege, M. J. Spinetta, K.-H. Kim, M. Duran-Moreno, T. Schallert, F. Zindy, M. F. Roussel, J. M. Garcia-Verdugo and P. Casaccia, Eur. J. Neurosci., 2011, 34, 1040–1052 CrossRef PubMed.
- A. H. Sayed, T. Watanabe-Asaka, S. Oda and H. Mitani, J. Photochem. Photobiol., B, 2016, 161, 1–8 CrossRef CAS PubMed.
- A. H. Sayed, C. Kataoka, S. Oda, S. Kashiwada and H. Mitani, Environ. Toxicol. Pharmacol., 2018, 58, 98–104 CrossRef CAS PubMed.
- N. Kumar, P. Antony Jesu Prabhu, A. K. Pal, S. Remya, M. Aklakur, R. S. Rana, S. Gupta, R. P. Raman and S. B. Jadhao, Pestic. Biochem. Physiol., 2011, 99, 45–52 CrossRef CAS.
- T. N. Armstrong, R. Reimschuessel and B. P. Bradley, Aquat. Toxicol., 2002, 58, 1–14 CrossRef CAS PubMed.
- K. Tokuda, T. Tsukamoto, S. Fujisawa and M. Matsubara, Acta Ophthalmol. Scand., 2004, 82, 189–194 CrossRef PubMed.
- T. S. Vihtelic and D. R. Hyde, J. Neurobiol., 2000, 44, 289–307 CrossRef CAS PubMed.
- J. L. Thomas, C. M. Nelson, X. Luo, D. R. Hyde and R. Thummel, Exp. Eye Res., 2012, 97, 105–116 CrossRef CAS PubMed.
- R. Thummel, S. C. Kassen, J. M. Enright, C. M. Nelson, J. E. Montgomery and D. R. Hyde, Exp. Eye Res., 2008, 87, 433–444 CrossRef CAS PubMed.
- R. Thummel, S. C. Kassen, J. E. Montgomery, J. M. Enright and D. R. Hyde, Dev. Neurobiol., 2008, 68, 392–408 CrossRef PubMed.
- T. S. Vihtelic, J. E. Soverly, S. C. Kassen and D. R. Hyde, Exp. Eye Res., 2006, 82, 558–575 CrossRef CAS PubMed.
- T. J. Bailey, S. L. Fossum, S. M. Fimbel, J. E. Montgomery and D. R. Hyde, Exp. Eye Res., 2010, 91, 601–612 CrossRef CAS PubMed.
- D. M. Allen and T. E. Hallows, Vis. Neurosci., 1997, 14, 589–600 CrossRef CAS PubMed.
-
D. M. Allen, C. Pipes, K. Deramus and T. E. Hallows, in Retinal Degenerative Diseases and Experimental Therapy, ed. J. G. Hollyfield, R. E. Anderson and M. M. LaVail, Springer US, Boston, MA, 1999, pp. 337–350 Search PubMed.
- W. T. Allison, T. E. Hallows, T. Johnson, C. W. Hawryshyn and D. M. Allen, Vis. Neurosci., 2006, 23, 25–34 CrossRef PubMed.
-
R. N. Auer and G. R. Sutherland, in Hypoxia and related conditions, Edward and Arnold, London, 7th edn., 2002, ch. 2, p. 233 Search PubMed.
- F. Peri and C. Nusslein-Volhard, Cell, 2008, 133, 916–927 CrossRef CAS PubMed.
- T. Yasuda, Y. Kimori, K. Nagata, K. Igarashi, T. Watanabe-Asaka, S. Oda and H. Mitani, J. Radiat. Res., 2016, 57, 9–15 CrossRef CAS PubMed.
- Y. Isoe, T. Okuyama, Y. Taniguchi, T. Kubo and H. Takeuchi, Biochem. Biophys. Res. Commun., 2012, 423, 627–631 CrossRef CAS PubMed.
- S. Ghosh and S. P. Hui, Neural Plast., 2016, 5815439, 13 Search PubMed.
|
This journal is © The Royal Society of Chemistry and Owner Societies 2019 |
Click here to see how this site uses Cookies. View our privacy policy here.