DOI:
10.1039/C8RA07447J
(Paper)
RSC Adv., 2019,
9, 2199-2204
Continuous flow synthesis of diaryl ketones by coupling of aryl Grignard reagents with acyl chlorides under mild conditions in the ecofriendly solvent 2-methyltetrahydrofuran†
Received
6th September 2018
, Accepted 10th January 2019
First published on 17th January 2019
Abstract
An efficient continuous flow sequential synthesis of diaryl ketones was achieved by coupling of aryl Grignard reagents with acyl chlorides in the bio-derived “green” solvent 2-methyltetrahydrofuran (2-MeTHF) under mild reaction conditions (ambient temperature, 1 hour), allowing a safe and on-demand generation of 2-(3-benzoylphenyl)propionitrile with a productivity of 3.16 g hour−1.
Introduction
Diaryl ketones are frequently used as core motifs for developing pharmaceutical agents.1–3 They exist in numerous bioactive molecules (Fig. 1),1,4 such as the nonsteroidal anti-inflammatory drug (NSAID) ketoprofen,5 the broad-spectrum human anthelmintic mebendazole,6 the classical lipid regulating agent fenofibrate7 and the therapeutically useful uricosuric agent benzbromarone (BBR)8 (Fig. 1). Therefore, development of synthetic methods for diarylketones continues to be a highly active area in synthetic chemistry (Scheme 1). The conventional method is Friedel–Crafts acylation of arenes, which has drawbacks such as the requirement for superstoichiometric amounts of Lewis acid and low regioselectivity.9,10 Alternative synthetic approaches have been developed, including oxidation of secondary alcohols,11,12 acylation of organometallic reagents,13–16 transition-metal-catalyzed cross-coupling reactions of carboxylic acid derivatives with organometallic reagents,17–24 palladium-catalyzed carbonylative cross-coupling reactions between aryl halides and related compounds with carbon nucleophiles,25–30 acylation of (hetero)aryl halides with aldehydes,31 decarboxylative cross-coupling reactions,32,33 decarboxylative addition reactions,33 and other uncommon reactions.34 Unfortunately, there remain a number of limitations with regards to expensive catalysts, safety concerns, and environmentally practical considerations.
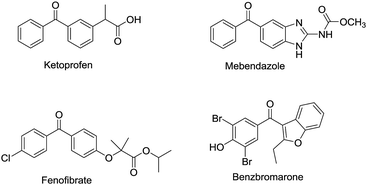 |
| Fig. 1 Pharmaceutical with diary ketone motif. | |
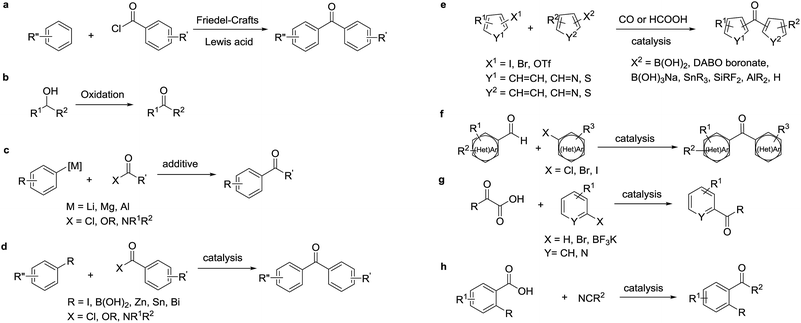 |
| Scheme 1 (a) Friedel–Crafts acylation, (b) oxidation of the corresponding secondary alcohols, (c) acylation of organometallic reagents, (d) transition-metal-catalyzed cross-coupling reactions of carboxylic acid derivatives with organometallic reagents, (e) palladium-catalyzed carbonylative cross-coupling reactions between aryl halides and related compounds with carbon nucleophiles, (f) acylation of (hetero)aryl halides with aldehydes, (g) decarboxylative cross-coupling reactions, (h) decarboxylative addition reactions. | |
Compared to traditional batch processing, flow chemistry represents a powerful technology with several advantages including safety, cost, efficiency, robustness and quality. It is considered an ideal partner with green chemistry,35 and is a powerful tool for the pharmaceutical industry.36–38 Recently, the Jamison group described an efficient continuous flow synthesis of ketones from carbon dioxide and organolithium or Grignard reagents at ambient temperature and pressure.39 The Kim group developed an efficient method for the synthesis of ketones using organolithium reagents and acid chlorides under continuous flow conditions at −40 °C.40 Unfortunately, most of the solvents used in the synthesis of diaryl ketones are not environmentally friendly. In recent years there has been a trend towards the use of bio-derived, green solvents as replacements for hazardous and/or environmentally-damaging solvents.35 In fact, 2-methyltetrahydrofuran (2-MeTHF) is one such solvent that has been developed as a greener alternative to classical solvents.41,42 With our interest in developing green organic reactions for the synthesis of diaryl ketones, herein we describe an efficient continuous flow parallel synthesis of diaryl ketones by the direct coupling of aryl Grignard reagents with acyl chlorides without additives at ambient temperature using the bio-derived “green” solvent 2-methyltetrahydrofuran (2-MeTHF). We demonstrated the scalability of our method by achieving a productivity of 3.16 g hour−1 in the synthesis of 2-(3-benzoylphenyl)propionitrile, an important intermediate in the synthesis of ketoprofen.
Results and discussion
To optimize the parameters (concentration, solvent, flow rate, residence time and temperature) (Table 1), our initial studies focused on the coupling between phenylmagnesium bromide and benzoyl chloride using a simple continuous system comprised of two standard-pressure HPLC pumps, a T-shaped mixer (Peek 1/32′′ inner diameter) and a standard PTFE reactor (poly tetrafluoroethylene, 1/16′′ inner diameter, 120.0 mL internal volume). Upon exiting the reactor, the reaction stream was immediately quenched with 1 N HCl. The results indicated that concentration obviously impacted the yield, as high concentrations clogged the reactor (entry 1) and low concentrations decreased the yield (entry 4). The optimal concentrations of 2a and 3a were 0.6 M and 0.4 M respectively. Some common solvents such as ether, dioxane, and 1,2-dimethoxyethane, were investigated, and the results demonstrated that none were suitable due to clogging the reactor (entries 5–7). The substitution of 2-MeTHF (entry 8) for THF (entry 3) proved to be benign to the reaction, and room temperature led to the highest yield of 85% (entry 8). These results clearly show that this procedure fits the 5th and 6th principles of Green Chemistry very well, which demand biomass-derived solvents that meet the 3R requirements (reduce, recycle, and reuse) and energy efficiency by employing synthetic methods conducted at mild temperatures and atmospheric pressure.35 After prolonging the residence time from 30 min to 120 min by lowering the flow rate (entries 8, 11, and 12), we determined that a 60 min residence time afforded the best yield (85%). Decreasing the stoichiometric ratio of 1a to 2a from 3
:
2 to 1
:
1 had a detrimental effect on the formation of 3a (entry 13). For comparative purposes the reaction was run as a standard batch process, applying the same concentration of reagents, temperature, and reaction time as optimized in the flow protocol (entry 8). We found that the yield of the batch process to produce compound 3a was 34%, which is similar to the reported yield at −78 °C,43 and, significantly lower than the yield of the optimized flow protocol. Based on the GC-MS of 3a from batch process, the major byproduct 7 was identified (ESI†). We proposed the possible mechanism that Grignard reagent 1a discomposed to radical 5 when the internal temperature increased by addition of a solution of benzoyl chloride 2b, followed by oxidation to phenol (Scheme 2).44–47
Table 1 Optimization of the coupling between phenylmagnesium bromide and benzoyl chloride in continuous flow
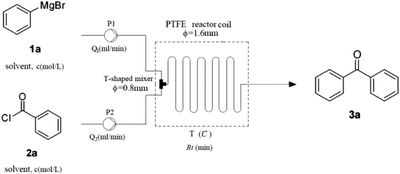
|
Entry |
Solvent |
c (mol L−1) |
Q (mL min−1) |
Rt (min) |
T (°C) |
Yielda (%) |
1a |
2a |
1 |
2 |
Isolated yield from 4 mmol of 2a by flash column chromatography. System clogged. Yield in ref. 44. Batch reaction. |
1 |
THF |
1.2 |
0.8 |
1 |
1 |
— |
rt |
—b |
2 |
THF |
0.9 |
0.6 |
1 |
1 |
60 |
rt |
71 |
3 |
THF |
0.6 |
0.4 |
1 |
1 |
60 |
rt |
72 |
4 |
THF |
0.3 |
0.2 |
1 |
1 |
60 |
rt |
56 |
5 |
Ether |
0.6 |
0.4 |
1 |
1 |
— |
rt |
—b |
6 |
Dioxane |
0.6 |
0.4 |
1 |
1 |
— |
rt |
—b |
7 |
DME |
0.6 |
0.4 |
1 |
1 |
— |
rt |
—b |
8 |
2-MeTHF |
0.6 |
0.4 |
1 |
1 |
60 |
rt |
85 |
9 |
2-MeTHF |
0.6 |
0.4 |
1 |
1 |
60 |
0 |
64 |
10 |
2-MeTHF |
0.6 |
0.4 |
1 |
1 |
60 |
50 |
70 |
11 |
2-MeTHF |
0.6 |
0.4 |
2 |
2 |
30 |
rt |
61 |
12 |
2-MeTHF |
0.6 |
0.4 |
0.5 |
0.5 |
120 |
rt |
54 |
13 |
2-MeTHF |
0.4 |
0.4 |
1 |
1 |
60 |
rt |
61 |
14 |
THF |
0.4 |
0.4 |
— |
— |
120 |
−78 |
33c |
15 |
2-MeTHF |
0.6 |
0.4 |
— |
— |
60 |
rt |
34d |
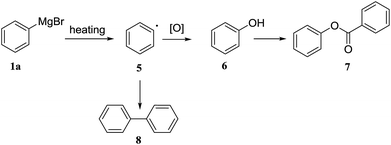 |
| Scheme 2 Possible mechanism for low yield of 3a from the batch process. | |
Over the past several years, high-throughput chemical synthesis has become increasingly important due to its potential to positively impact the drug discovery process.48–51 With the proof of concept, we explored the sequential flow synthesis of a library of diaryl ketones using the optimized reaction conditions. Firstly, we evaluated the substrate scope for various aryl acid chlorides with phenylmagnesium bromide using a continuous flow parallel system adjusted by a five-way valve. The data in Table 2 clearly demonstrate that the formation of diaryl ketones from acyl chlorides was quite general and occurred smoothly in the continuous flow system, with moderate to good yields. Among the acid chlorides with meta substituents, yields of fluoride 3f (65%), chloride 3h (82%), and trifluoromethylated compound 3i (77%) were higher than those of methylated compound 3c (57%), methoxylated compound 3k (60%), and 1-cyanoethylated compound 3o (56%). These results indicate that presence of electron-withdrawing groups on the acyl chlorides has a positive influence on the reaction with phenylmagnesium bromide. Interestingly, among the compounds 3b–3d and 3e–3g, the yields of ortho-substituted ketones (3b and 3e) were clearly better than those of the corresponding meta-substituted (3c and 3f) or para-substituted ketones (3d and 3g).
Table 2 Reaction of phenylmagnesium bromides 1a with acyl chloride 2b–o under continuous flow parallel system
In addition, the coupling reaction with 4-cyanobenzoyl chloride and 2-naphthoyl chloride afforded moderate yields.
Secondly, we further explored the substrate scope for various arylmagnesium bromides with 2-fluorobenzoyl chloride using the simple flow synthesis system. The data in Table 3 demonstrate that arylmagnesium bromides with electron-withdrawing, electron-neutral, or electron-donating groups were well-tolerated in the reaction with 2-fluorobenzoyl chloride, affording ketones in moderate to excellent yields (Table 3, entries 1–7). Interestingly, the reaction of o-tolylmagnesium bromide with 2-fluorobenzoyl chloride afforded almost quantitative yield (entry 2).
Table 3 Reaction of aryl Grignard reagents 1b–h with 2-fluorobenzoyl chloride 2e under continuous flow parallel system
Finally, to demonstrate the scalability of our method, 4.74 g of 2-(3-benzoylphenyl)propionitrile (3o), an important intermediate of ketoprofen, was straightforwardly produced after 1.5 h (output 3.16 g per hour). Yield and purity were similar to that of the small scale reaction, showing that the reaction conditions identified for the production of a few milligrams could be transferred to a larger scale without any further optimization.
Conclusions
In summary, we developed a novel continuous flow method for parallel synthesis of diaryl ketones using commercially available reagents. The continuous flow nature of our synthesis enabled the facile scale-up of these compounds, which would be highly advantageous for drug discovery. The alignment of our work with green chemistry principles should result in its adoption by the chemistry community.
Conflicts of interest
The authors declare no conflict of interest.
Acknowledgements
This work was supported by National Natural Science Foundation of China (21772068), the Department of Science and Technology of Jiangsu Province (BY2016022-37), and National Science & Technology Major Project “Key New Drug Creation and Manufacturing Program”, China (Number: 2018ZX09711002).
Notes and references
- M. H. Keylor, B. S. Matsuura and C. R. J. Stephenson, Chem. Rev., 2015, 115, 8976–9027 CrossRef CAS PubMed.
- X. Qi, L.-B. Jiang, H.-P. Li and X.-F. Wu, Chem.–Eur. J., 2015, 21, 17650–17656 CrossRef CAS PubMed.
- M. L. N. Rao and B. S. Ramakrishna, Eur. J. Org. Chem., 2017, 5080–5093 CrossRef CAS.
- I. Jabeen, K. Pleban, U. Rinner, P. Chiba and G. F. Ecker, J. Med. Chem., 2012, 55, 3261–3273 CrossRef CAS PubMed.
- E. A. Smith, J. G. Marshall, S. S. Selph, D. R. Barker and C. M. Sedgley, J. Endod., 2017, 43, 7–15 CrossRef PubMed.
- S. C. Zimmermann, T. Tichy, J. Vavra, R. P. Dash, C. E. Slusher, A. J. Gadiano, Y. Wu, A. Jančařík, L. Tenora and L. Monincova, Drug Metab. Pharmacokinet., 2018, 61, 3918–3929 CrossRef CAS PubMed.
- V. Agarwal, M. Bajpai and A. Sharma, Recent Pat. Drug Delivery Formulation, 2018, 12, 40–52 CrossRef CAS PubMed.
- J. Zheng, Drug Metab. Pharmacokinet., 2018, 33, S70 CrossRef.
- H. Firouzabadi, N. Iranpoor and F. Nowrouzi, Tetrahedron, 2004, 60, 10843–10850 CrossRef CAS.
- P. H. Tran, H. Q. Phung, P. E. Hansen, H. N. Tran and T. N. Le, Synth. Commun., 2016, 46, 893–901 CrossRef CAS.
- K.-J. Liu, S. Jiang, L.-H. Lu, L.-L. Tang, S.-S. Tang, H.-S. Tang, Z. Tang, W.-M. He and X. Xu, Green Chem., 2018, 20, 3038–3043 RSC.
- X. Ye, M. D. Johnson, T. Diao, M. H. Yates and S. S. Stahl, Green Chem., 2010, 12, 1180–1186 RSC.
- F. Wang, A. Lu, D. Huang, Y. Su and X. Xia, Chin. J. Org. Chem., 2015, 35, 1046 CrossRef CAS.
- J. Nakatani and T. Nozoe, Org. Process Res. Dev., 2016, 20, 1633–1636 CrossRef CAS.
- X.-j. Wang, L. Zhang, X. Sun, Y. Xu, D. Krishnamurthy and C. H. Senanayake, Org. Lett., 2005, 7, 5593–5595 CrossRef CAS PubMed.
- M. Arisawa, Y. Torisawa, M. Kawahara, M. Yamanaka, A. Nishida and M. Nakagawa, J. Org. Chem., 1997, 62, 4327–4329 CrossRef CAS PubMed.
- S. Shi and M. Szostak, Org. Lett., 2016, 18, 5872–5875 CrossRef CAS PubMed.
- M. Blangetti, H. Rosso, C. Prandi, A. Deagostino and P. Venturello, Molecules, 2013, 18, 1188–1213 CrossRef CAS PubMed.
- S. Shi and M. Szostak, Chem.–Eur. J., 2016, 22, 10420–10424 CrossRef CAS PubMed.
- F. Rafiee and A. R. Hajipour, Appl. Organomet. Chem., 2015, 29, 181–184 CrossRef CAS.
- D. Lee, T. Ryu, Y. Park and P. H. Lee, Org. Lett., 2014, 16, 1144–1147 CrossRef CAS PubMed.
- D. Wang and Z. Zhang, Org. Lett., 2003, 5, 4645–4648 CrossRef CAS PubMed.
- J. W. Labadie and J. K. Stille, J. Am. Chem. Soc., 1983, 105, 6129–6137 CrossRef CAS.
- J. W. Labadie and J. K. Stille, J. Am. Chem. Soc., 1983, 105, 669–670 CrossRef CAS.
- X. Qi, L.-B. Jiang, H.-P. Li and X.-F. Wu, Chem.–Eur. J., 2015, 21, 17650–17656 CrossRef CAS PubMed.
- K. M. Bjerglund, T. Skrydstrup and G. A. Molander, Org. Lett., 2014, 16, 1888–1891 CrossRef CAS PubMed.
- X.-F. Wu, H. Neumann and M. Beller, Chem. Soc. Rev., 2011, 40, 4986–5009 RSC.
- M. Blangetti, H. Rosso, C. Prandi, A. Deagostino and P. Venturello, Molecules, 2013, 18, 1188–1213 CrossRef CAS PubMed.
- R. Garrison Kinney, J. Tjutrins, G. M. Torres, N. J. Liu, O. Kulkarni and B. A. Arndtsen, Nat. Chem., 2017, 10, 193–199 CrossRef PubMed.
- J. Tjutrins and B. A. Arndtsen, J. Am. Chem. Soc., 2015, 137, 12050–12054 CrossRef CAS PubMed.
- T. Wakaki, T. Togo, D. Yoshidome, Y. Kuninobu and M. Kanai, ACS Catal., 2018, 8, 3123–3128 CrossRef CAS.
- R. Shang and L. Liu, Sci. China: Chem., 2011, 54, 1670–1687 CrossRef CAS.
- N. Rodríguez and L. J. Goossen, Chem. Soc. Rev., 2011, 40, 5030–5048 RSC.
- Z. Lian, D. U. Nielsen, A. T. Lindhardt, K. Daasbjerg and T. Skrydstrup, Nat. Commun., 2016, 7, 13782 CrossRef PubMed.
- H. C. Erythropel, J. B. Zimmerman, T. M. de Winter, L. Petitjean, F. Melnikov, C. H. Lam, A. W. Lounsbury, K. E. Mellor, N. Z. Janković, Q. Tu, L. N. Pincus, M. M. Falinski, W. Shi, P. Coish, D. L. Plata and P. T. Anastas, Green Chem., 2018, 20, 1929–1961 RSC.
- S. G. Koenig and H. F. Sneddon, Green Chem., 2017, 19, 1418–1419 RSC.
- G. Bernhard, C. David and K. C. Oliver, Angew. Chem., Int. Ed., 2015, 54, 6688–6728 CrossRef PubMed.
- D. Perera, J. W. Tucker, S. Brahmbhatt, C. J. Helal, A. Chong, W. Farrell, P. Richardson and N. W. Sach, Science, 2018, 359, 429–434 CrossRef CAS PubMed.
- J. Wu, X. Yang, Z. He, X. Mao, T. A. Hatton and T. F. Jamison, Angew. Chem., Int. Ed., 2014, 53, 8416–8420 CrossRef CAS PubMed.
- S.-Y. Moon, S.-H. Jung, U. Bin Kim and W.-S. Kim, RSC Adv., 2015, 5, 79385–79390 RSC.
- S. Monticelli, L. Castoldi, I. Murgia, R. Senatore, E. Mazzeo, J. Wackerlig, E. Urban, T. Langer and V. Pace, Monatsh. Chem., 2017, 148, 37–48 CrossRef CAS PubMed.
- R. K. Henderson, C. Jiménez-González, D. J. C. Constable, S. R. Alston, G. G. A. Inglis, G. Fisher, J. Sherwood, S. P. Binks and A. D. Curzons, Green Chem., 2011, 13, 854–862 RSC.
- F. Sato, M. Inoue, K. Oguro and M. Sato, Tetrahedron Lett., 1979, 20, 4303–4306 CrossRef.
- M. S. Kharasch and W. B. Reynolds, J. Am. Chem. Soc., 1943, 65(4), 501–504 CrossRef CAS.
- M. S. Kharasch and W. H. Urry, J. Org. Chem., 1948, 13(1), 101–109 CrossRef CAS PubMed.
- Z. He and T. F. Jamison, Angew. Chem., Int. Ed., 2014, 53(13), 3353–3357 CrossRef CAS PubMed.
- S. Butini, E. Gabellieri, P. B. Huleatt, G. Campiani, S. Franceschini, M. Brindisi, S. Ros, S. S. Coccone, I. Fiorini, E. Novellino, G. Giorgi and S. Gemma, J. Org. Chem., 2008, 73(21), 8458–8468 CrossRef CAS PubMed.
- G. Schneider, Nat. Rev. Drug Discovery, 2017, 17, 97–113 CrossRef PubMed.
- R. Martín-Rapún, S. Sayalero and M. A. Pericàs, Green Chem., 2013, 15, 3295–3301 RSC.
- L. Maestre, E. Ozkal, C. Ayats, A. Beltrán, M. M. Díaz-Requejo, P. J. Pérez and M. A. Pericàs, Chem. Sci., 2015, 6, 1510–1515 RSC.
- L. Osorio-Planes, C. Rodríguez-Escrich and M. A. Pericàs, Catal. Sci. Technol., 2016, 6, 4686–4689 RSC.
Footnote |
† Electronic supplementary information (ESI) available. See DOI: 10.1039/c8ra07447j |
|
This journal is © The Royal Society of Chemistry 2019 |
Click here to see how this site uses Cookies. View our privacy policy here.