DOI:
10.1039/C8RA09928F
(Paper)
RSC Adv., 2019,
9, 3303-3310
Mn4+-activated BaLaMgSbO6 double-perovskite phosphor: a novel high-efficiency far-red-emitting luminescent material for indoor plant growth lighting
Received
3rd December 2018
, Accepted 18th January 2019
First published on 24th January 2019
Abstract
In the present work, novel high-efficiency Mn4+-activated BaLaMgSbO6 (BLMS) far-red-emitting phosphors used for plant growth LEDs were successfully synthesized via a solid-state reaction method. X-ray diffraction (XRD), photoluminescence (PL), temperature-dependent PL, CIE color coordinates, and lifetimes as well as internal quantum efficiency (IQE) were used to characterize the phosphor samples. The excitation spectrum of the as-obtained BLMS:Mn4+ phosphors presented two wide bands covering 250–550 nm and the emission spectrum exhibited a far-red emission band in the range of 650–800 nm peaked at 700 nm. Concentration-dependent PL properties of BLMS:Mn4+ phosphors were studied. The optimal doping concentration of Mn4+ ions was 0.6 mol%, and the concentration quenching mechanism was determined to be the nonradiative energy transfer among the nearest-neighbor Mn4+ activators. Impressively, the BLMS:0.6%Mn4+ sample showed an outstanding IQE of 83%. In addition, luminescence thermal quenching characteristics were also analyzed. Furthermore, the PL spectrum of BLMS:0.6%Mn4+ sample was compared with the absorption spectrum of phytochrome PFR. Finally, after combining BLMS:0.6%Mn4+ phosphors with a 365 nm near-UV LED chip, a far-red light-emitting diode (LED) device was successfully achieved to demonstrate its possible applications in plant growth LEDs.
1. Introduction
Recently, red-emitting luminescent materials doped with rare-earth ions (such as Eu3+, Eu2+, Ce3+) and transitional metal ions (such as Mn4+ and Cr3+) have attracted much attention due to their promising applications in lighting and displays.1–14 Mn4+ ion with a 3d3 electron configuration belongs to the transitional metal ion, and in recent years Mn4+-activated red-emitting phosphors have been widely reported for warm-white light-emitting diodes (LEDs) towards general lighting applications.15–19 Generally, Mn4+ ions can substitute for W6+, Sn4+, Sb5+, Al3+, Ge4+, Ta5+, and Ti4+ ions in the octahedral or distorted octahedral systems.20–30 Just recently, Mn4+ doped oxide-based phosphors with excellent luminescent properties and chemical stability such as Li2MgZrO4:Mn4+, Ca3La2W2O12:Mn4+, and La(MgTi)1/2O3:Mn4+ have been researched for their potential applications in far-red LEDs for indoor plant growth.31–33 It is known that far-red light around 730 nm (700–740 nm) is most needed for photosynthesis in all plant growth, especially the growth of plant stems.34,35 The perovskite-type compounds are potential hosts for luminescent materials due to their excellent optical properties and diversity of their structure and composition.36–44 Therefore, double perovskite BaLaMgSbO6 (BLMS), which contains many [SbO6] octahedrons, would be a novel good host for doping Mn4+ ions.
In this work, highly efficient BLMS:Mn4+ far-red-emitting phosphors were synthesized by a high-temperature solid-state reaction method in air atmosphere. Upon 340 nm excitation, BLMS:Mn4+ phosphors gave a far-red emission band between 650 and 800 nm with a maximum peak at 700 nm, which matched well with the absorption band of phytochrome PFR. The optimal Mn4+ ions doping concentration was about 0.6 mol%, for which the BLMS:Mn4+ phosphors exhibited the highest luminescence intensity. The full width at half maximum (FWHM) and CIE chromaticity coordinates of BLMS:0.6%Mn4+ sample were ∼38 nm and (0.7231, 0.2768), respectively. More importantly, when excited at 340 nm, the BLMS:0.6%Mn4+ sample had internal quantum efficiency (IQE) as high as 83%. These results suggested that the BLMS:Mn4+ could be considered as promising far-red-emitting phosphors for application in far-red LEDs towards indoor plant growth lighting.
2. Experimental
A series of BaLaMgSb1−xO6:xMn4+ (BLMS:xMn4+; x = 0.2, 0.4, 0.6, 0.8, 1.0, and 1.2 mol%) phosphors were synthesized by using a high-temperature solid-state reaction method with BaCO3 (analytical reagent, AR), La2O3 (99.99%), MgO (AR), Sb2O5 (AR), and MnCO3 (AR) as starting raw materials. These materials were weighed based on the stoichiometric ratio and ground thoroughly in an agate mortar. The mixed mixtures were firstly heated at 600 °C for 3 h. Subsequently, they were reground and sintered again at 1500 °C for 6 h. Finally, the resulting products were cooled down to room temperature naturally and then ground once again to get the fine powders for further characterization.
The X-ray diffraction (XRD) patterns of the samples were identified using a Bruker D8 X-ray diffractometer with Cu Kα radiation (λ = 1.5406 Å). The photoluminescence (PL) and PL excitation (PLE) spectra as well as decay curves of the as-obtained phosphors were recorded on an Edinburgh FS5 spectrometer equipped with a 150 W continued-wavelength Xenon lamp and a pulsed Xenon lamp, respectively. The IQE was measured by using the Edinburgh FS5 spectrometer equipped with an integrating sphere coated with BaSO4. The temperature-dependent PL spectra of the phosphors ranging from 303 to 463 K were recorded on the same spectrometer with a temperature controlling system.
3. Results and discussion
Fig. 1 shows the XRD patterns of BLMS:xMn4+ (x = 0.2%, 0.6%, 0.8%, and 1.2%) samples. All the observed diffraction peaks of the as-obtained samples were similar, suggesting that doping Mn4+ ions did not significantly influence the crystal structure. To further gain more structural information, the Rietveld refinement of BLMS:0.6%Mn4+ was conducted, as shown in Fig. 1(b). The cross shows the observed patterns, the red solid line indicates the calculated patterns, the green solid line refers to the background line, the blue line represents the difference between the experimental and calculated data, and short vertical pink line stands for the positions of Bragg reflection. Moreover, the refinement results demonstrated that the BLMS:0.6%Mn4+ belonged to a cubic system with space group Fm
m. The lattice parameters were calculated to be a = b = c = 8.04869 Å, α = β = γ = 90°, V = 525.406 Å3, and N = 4. The crystal structure of BLMS:0.6%Mn4+ was displayed in Fig. 1(c). Sb5+ and Mg2+ were surrounded by the six nearest-neighbor oxygen ligand ions, which formed [SbO6] and [MgO6] octahedrons. As well known, when Mn4+ ions entered into the octahedral sites, red emission might be observed. According to the difference of ionic radii (r (Mn4+) = 0.53 Å, r (Sb5+) = 0.60 Å, r (Mg2+) = 0.72 Å, r (Ba2+) = 1.35 Å, and r (La3+) = 1.32 Å), so Mn4+ ions preferred to occupy the Sb5+ sites in BLMS host owing to their similar ionic radii.
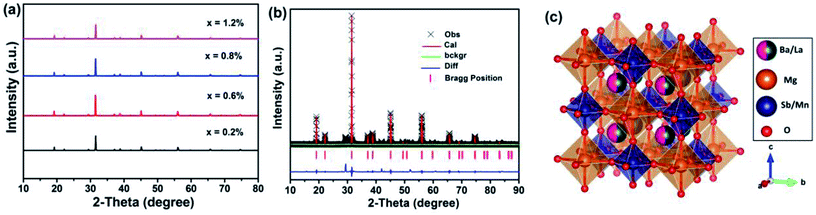 |
| Fig. 1 (a) XRD patterns of BLMS:xMn4+ (x = 0.2%, 0.6%, 0.8% and 1.2%) phosphors. (b) Rietveld refinement XRD patterns of BLMS:0.6%Mn4+ phosphors. (c) The crystal structure of BLMS:0.6%Mn4+. | |
Fig. 2(a) shows the PLE and PL spectra of BLMS:0.6%Mn4+ sample at room temperature. Monitored with a emission wavelength of 700 nm, the obtained PLE spectrum of BLMS:0.6%Mn4+ sample consisted of two wide bands covering 250–550 nm, which could be deconvoluted into three Gaussian component peaks at 336 nm, 383 nm, and 501 nm. The first strong band centered at 336 nm was assigned to the Mn–O charge transfer band (CTB) and the Mn4+ spin-allowed transitions of 4A2g → 4T1g.45 The second band centered at 383 nm was attributed to the spin-allowed transition of Mn4+: 4A2g → 2T2g.46 The third band centered at 501 nm was due to the spin-allowed transition of Mn4+: 4A2g → 4T2g.47 The broad excitation bands of BLMS phosphors located in the near-UV region, indicating that BLMN:Mn4+ were suitable to be used as far-red phosphors with efficient excitation of near-UV LED chips.48,49 Upon 340 nm excitation, the obtained emission spectrum had a narrow emission band in the wavelength range of 650–800 nm peaking at 700 nm, which was attributed to the spin-forbidden 2Eg → 4A2g transition of Mn4+.50–52 The full width at half maximum (FWHM) of the emission band was about 38 nm, which was narrower than that of Ca3La2W2O12:Mn4+ (FWHM: 39 nm), Li2MgTiO4:Mn4+ (FWHM: 46 nm), and Na2MgAl10O17:Mn4+ (FWHM: 105 nm).1,32,51
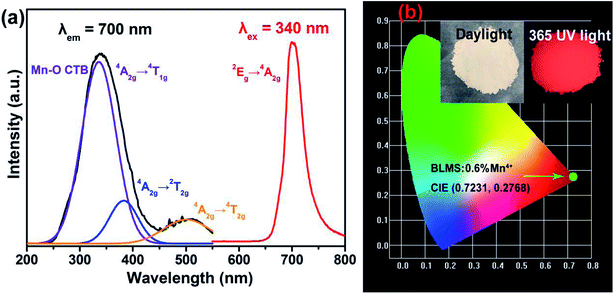 |
| Fig. 2 (a) PLE and PL spectra of BLMS:0.6%Mn4+ phosphors. (b) CIE chromaticity coordinates of BLMS:0.6%Mn4+ phosphors under 340 nm excitation. The inset shows the digital photographs of BLMS:0.6%Mn4+ phosphors in daylight and under a 365 nm UV lamp. | |
The CIE chromaticity coordinates of BLMS:0.6%Mn4+ sample were calculated and illustrated in Fig. 2(b). The CIE coordinates of BLMS:0.6%Mn4+ were found to be (0.7231, 0.2768), which located near the edge of the CIE diagram. Obviously, BLMS:Mn4+ phosphors had the good CIE chromaticity coordinates, which were located in the far-red region. As shown in the inset of Fig. 2(b), the phosphors emitted bright red light under 365 nm UV light, which indicated that the BLMS:Mn4+ phosphors were remarkably suitable for indoor plant growth.
Fig. 3(a) shows the PL spectra of BLMS:Mn4+ phosphors with various Mn4+ doping concentrations. As depicted in Fig. 3(a), the shapes and positions of the emission spectra of all the samples were almost identical except for the emission intensities, which further proved that doping Mn4+ ions had little influence on crystal structure. As exhibited in Fig. 3(b), the emission intensity of this series of phosphors increased first and reached a maximum at x = 0.6%, then decreased with further increase in the Mn4+ concentration. This phenomenon was attributed to concentration quenching effect.53 Concentration quenching is usually caused by the energy transfer within the nearest Mn4+ ions with the terminal step ending at a defect or killer site.1,54 Since there was no overlap between PLE and PL spectra of BLMS:Mn4+, so the radiation reabsorption was not dominant energy transfer mechanism. In order to figure out which mechanism was responsible for the concentration quenching among Mn4+ ions in the BLMS host, the critical distance (Rc) between Mn4+ ions was roughly calculated using the following equation:55,56
|
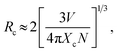 | (1) |
where
V is the volume of the host lattice,
Xc refers to the critical doping concentration of Mn
4+ ions, and
N is the number of available sites for the dopant in the unit cell. In this work, the
Xc = 0.6%;
V = 525.406 Å
3; and
N = 4, respectively. Therefore, the calculated
Rc value for Mn
4+ was determined to be 34.71 Å, which was higher than 5 Å. Consequently, we inferred that the concentration quenching mainly took place
via an electric multipolar interaction among Mn
4+ ions. To further determine the type of interaction mechanism between Mn
4+ ions, we could use the following equation:
57 |
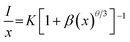 | (2) |
where
I is the emission intensity of BLMS:Mn
4+ phosphors;
x represents the Mn
4+ concentration;
K and
β are the constants for the same excitation condition; and
θ is an index of the electric multipolar character with
θ = 3, 6, 8 and 10 corresponding to the nonradiative energy transfer among the nearest-neighbor ions, dipole–dipole, dipole-quadrupole and quadrupole–quadrupole interactions, respectively.
58,59 The dependence of log(
I/
x) on log(
x), as clearly displayed in
Fig. 3(c), was well linearly fitted with a slope (−
θ/3) = −1.4952. Thus
θ = 4.4856, which was approximately calculated to be 3, implying that the major concentration quenching mechanism in BLMS:Mn
4+ was the nonradiative energy transfer among the nearest-neighbor Mn
4+ ions.
58–60
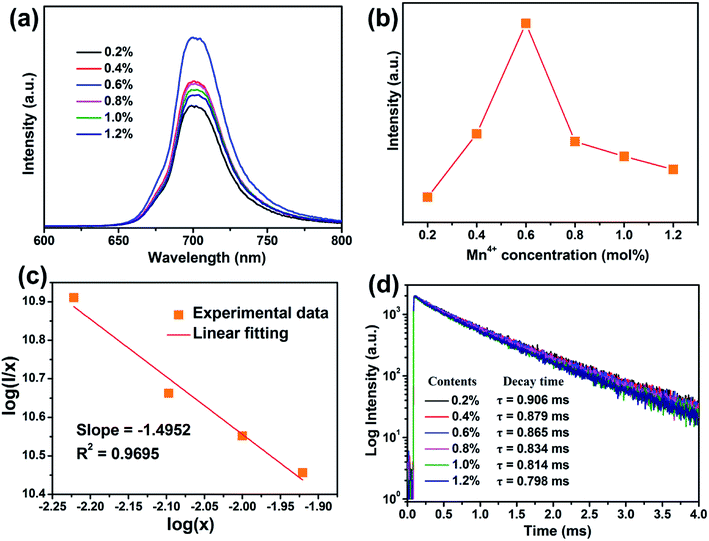 |
| Fig. 3 (a) PL spectra of BLMS:xMn4+ (x = 0.2%, 0.4%, 0.6%, 0.8%, 1.0%, and 1.2%) phosphors under 340 nm excitation. (b) PL intensity of BLMS:xMn4+ as a function of Mn4+ doping concentration. (c) Linear fitting of log(x) versus log(I/x) in BLMS:xMn4+ phosphors excited at 340 nm. (d) Decay curves and calculated lifetimes of BLMS:xMn4+ (x = 0.2%, 0.4%, 0.6%, 0.8%, 1.0%, and 1.2%) under 340 nm excitation and monitored at 700 nm. | |
Fig. 3(d) illustrates the room-temperature PL decay curves of the 700 nm emissions of BLMS:xMn4+ samples upon 340 nm excitation. All the decay curves could be well-fitted by using the following equation:61
|
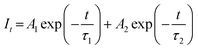 | (3) |
where
It is the luminescence intensities at time
t;
A1 and
A2 represent constants; and
τ1 and
τ2 correspond to the short and long lifetimes for the exponential components, respectively. Furthermore, the average lifetime
τs could be calculated by the following equation:
62 |
τs = (A1τ12 + A2τ22)/(A1τ1 + A2τ2)
| (4) |
The calculated lifetimes were also shown in Fig. 3(d). It was found that the luminescence lifetime decreased gradually from 0.906 to 0.798 ms with increasing Mn4+ concentrations, which was due to the increasing possibility of nonradiative energy migration among the adjacent Mn4+ ions.27
The temperature-dependent PL spectra of BLMS:0.6%Mn4+ phosphors under excitation at 340 nm were measured and illustrated in Fig. 4(a). Note that the emission profiles of BLMS:0.6%Mn4+ sample at different temperatures almost did not change except for PL intensity. It was clearly observed that the emission intensity decreased continuously with increasing temperature because of the thermal quenching effect. Fig. 4(b) shows the normalized PL intensity of the BLMS:0.6%Mn4+ phosphors as a function of temperature. The emission intensity at 423 K (150 °C) remained 37% of that at 303 K (30 °C). Moreover, the activation energy (Ea) was calculated via the equation, which is expressed as follows:63,64
|
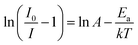 | (5) |
where
I0 and
I represent the emission intensity of the BLMS:0.6%Mn
4+ phosphor at room temperature and at different given temperatures
T, respectively;
k is the Boltzmann coefficient;
A is the constant; and
Ea is activation energy. According to the linear relationship between ln(
I0/
I − 1) and 1/
kT, which was plotted in
Fig. 4(c), the value of
Ea was obtained to be 0.315 eV. The relatively high
Ea demonstrated that the as-obtained phosphors had good thermal stability.
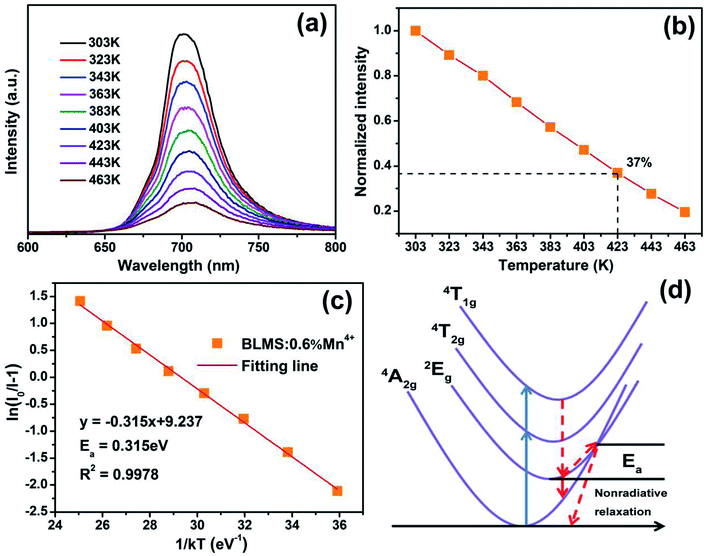 |
| Fig. 4 (a) Temperature-dependent PL spectra of BLMS:0.6%Mn4+ phosphors under 340 nm excitation. (b) The normalized PL intensities of BLMS:0.6%Mn4+ phosphors at different temperature from 303 to 463 K. (c) The plot of ln(I0/I − 1) versus 1/kT and the calculated activation energy (Ea) for the BLMS:0.6%Mn4+ phosphors. (d) The configuration coordinate diagram of Mn4+. | |
The thermal quenching mechanism of BLMS:Mn4+ could be explained by the configuration coordinate scheme, as illustrated in Fig. 4(d). When the electrons at ground state 4A2g absorbed energy, they would be pumped to the excited states of 4T1g and 4T2g, and then they could relax to the lowest excited state 2Eg through nonradiative transition (red dashed line). After that they returned to the ground states 4A2g by radiative transition (red solid line), which emitted far-red light. However, when the temperature was elevated, a partial electrons at 2Eg state were more likely to be thermally excited via the cross point between 4A2g and 2Eg states, and could finally transit to the ground state with nonradiative transition, leading to lower the luminescence efficiency, which was so-called thermal quenching.54
Fig. 5(a) shows the PL spectrum of BLMS:0.6%Mn4+ phosphors and the absorption spectrum of phytochrome PFR. An obvious spectral overlap existed between the emission band of BLMS:0.6%Mn4+ phosphors and the absorption of phytochrome PFR. Furthermore, the IQE of BLMS:0.6%Mn4+ sample could be calculated using the following equation:32
|
 | (6) |
where
η is IQE,
LS represents the emission spectrum of the sample,
ES and
ER correspond to the spectra of excitation light with sample and only with BaSO
4 reference, respectively. Thus, as shown in
Fig. 5(b), the IQE of BLMS:0.6%Mn
4+ sample was measured to be about 83%, which was much higher than that of many previously reported Mn
4+-activated perovskite-type red-emitting phosphors such as Li
2MgZrO
4:Mn
4+ (IQE: 32.3%), Ba
2YNbO
6:Mn
4+ (IQE: 29.2%), NaLaMgWO
6:Mn
4+ (IQE: 60%), and LiLaMgWO
6:Mn
4+ (IQE: 69.1%).
2,31,65,66
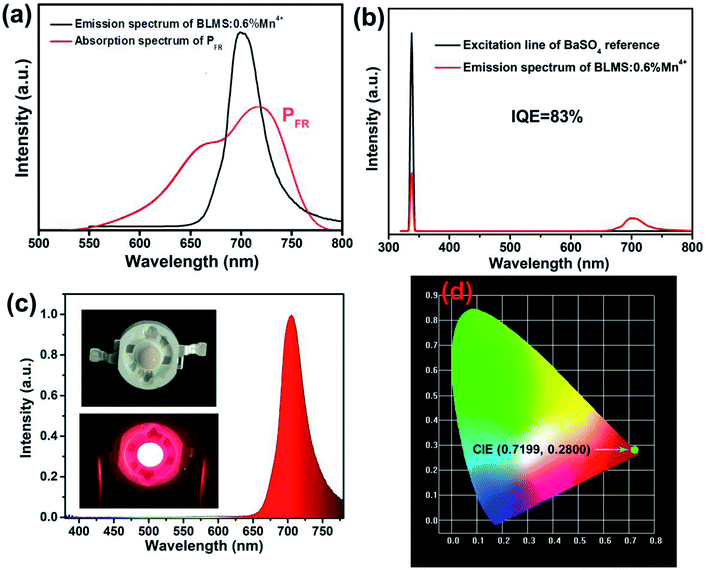 |
| Fig. 5 (a) PL spectrum of BLMS:0.6%Mn4+ phosphors and the absorption spectrum of phytochrome PFR. (b) Excitation line of BaSO4 and the PL spectrum of BLMS:0.6%Mn4+ phosphor collected using an integrating sphere. (c) EL spectrum of the fabricated far-red LED device by using BLMS:0.6%Mn4+ phosphors and a 365 nm near-UV LED chip under a driven current of 120 mA. Inset shows the fabricated LED device and corresponding luminescent image. (d) CIE chromaticity coordinates of the far-red LED device. | |
Moreover, we also compared our as-prepared BLMS:0.6%Mn4+ with some recent reported Mn4+-doped red phosphors for plant growth lighting. Table 1 summarizes their host materials, optimal Mn4+ doping concentrations, PL and PLE peaks and IQEs. Clearly, compared to other phosphors, our BLMS:0.6%Mn4+ phosphors had higher IQE and good PL properties. These results further demonstrated the excellent optical properties of the as-prepared BLMS:0.6%Mn4+ phosphors towards application in plant growth LEDs.
Table 1 Comparison of several reported Mn4+-activated red-emitting phosphors for indoor plant growth LEDs
Hosts |
Concentrations |
PLE peak (nm) |
PL peak (nm) |
IQE |
Ref. |
Li2MgZrO4 |
0.4% |
335 |
670 |
32.3% |
31 |
Sr9Y2W4O24 |
0.5% |
366 |
680 |
49.8% |
67 |
Ca2LaSbO6 |
0.5% |
354 |
687 |
52.2% |
68 |
CaYMgSbO6 |
0.2% |
294 |
688 |
51.5% |
69 |
Sr3NaSbO6 |
0.5% |
320 |
695 |
56.2% |
70 |
Ca2LaTaO6 |
0.4% |
325 |
696 |
34.6% |
71 |
Gd2ZnTiO6 |
0.2% |
365 |
705 |
39.7% |
26 |
La(MgTi)1/2O3 |
0.8% |
345 |
708 |
27.2% |
33 |
SrGdAlO4 |
0.1% |
353 |
709 |
23% |
72 |
Ca3La2W2O12 |
0.8% |
360 |
711 |
47.9% |
32 |
Ca14Al10Zn6O35 |
0.5% |
467 |
713 |
19.4% |
73 |
LiLaMgWO6 |
0.7% |
344 |
713 |
69.1% |
65 |
CaGdAlO4 |
0.2% |
349 |
715 |
45% |
29 |
BaLaMgSbO6 |
0.6% |
340 |
700 |
83% |
This work |
Finally, we fabricated a far-red LED device by coating the BLMS:0.6%Mn4+ phosphors onto the surface of a 365 nm near-UV LED chip. The electroluminescence (EL) spectrum of the corresponding LED device under 120 mA driven current was demonstrated in Fig. 5(c). As can be seen, an intense narrow far-red emission band peaking at 700 nm was presented. The CIE coordinates of the device emission were determined to be (0.7199, 0.2800), as shown in Fig. 5(d). These results showed that the as-prepared BLMS:Mn4+ phosphors could be promising far-red-emitting phosphors for far-red LEDs applications towards indoor plant growth lighting.
4. Conclusions
In summary, a series of novel Mn4+-activated BLMS far-red-emitting phosphors, which could be excited by UV or blue light, have been successfully prepared by a conventional high-temperature solid-state method. Under UV light excitation at 340 nm, the BLMS:Mn4+ phosphors gave rise to an intense far-red emission band peaking at 700 nm due to the 2Eg → 4A2g transition of Mn4+ ions, which could match well with the absorption spectra of phytochrome PFR. The optimal doping concentration of Mn4+ ions was determined to be 0.6 mol%, and the concentration quenching mechanism was the nonradiative energy transfer among the nearest-neighbor Mn4+ activators in the BLMS host. The CIE chromaticity coordinates of BLMS:0.6%Mn4+ sample were measured to be (0.7231, 0.2768). Furthermore, thermal stability was investigated by temperature-dependent emission spectra and the configuration coordinate diagram of Mn4+ ions. Finally, we fabricated a bright far-red LED device by coating the BLMS:0.6%Mn4+ phosphors onto the surface of a 365 nm near-UV LED chip. These results indicated the BLMS:Mn4+ red-emitting phosphors could be applied to obtain far-red LEDs for plant growth lighting.
Conflicts of interest
There are no conflicts to declare.
Acknowledgements
This work was supported by the National Natural Science Foundation of China (No. 51502190), the Program for the Outstanding Innovative Teams of Higher Learning Institutions of Shanxi, and the Open Fund of the State Key Laboratory of Luminescent Materials and Devices (South China University of Technology, No. 2017-skllmd-01).
References
- Y. Jin, Y. Hu, H. Wu, H. Duan, L. Chen, Y. Fu, G. Ju, Z. Mu and M. He, Chem. Eng. J., 2016, 288, 596–607 CrossRef CAS.
- A. Fu, Q. Pang, H. Yang and L. Zhou, Opt. Mater., 2017, 70, 144–152 CrossRef CAS.
- G. Jiang, B. Yang, G. Zhao, Y. Liu, J. Zou, H. Sun, H. Ou, Y. Fang and J. Hou, Opt. Mater., 2018, 83, 93–98 CrossRef CAS.
- L. Huang, Y. Liu, J. Yu, Y. Zhu, F. Pan, T. Xuan, M. G. Brik, C. Wang and J. Wang, ACS Appl. Mater. Interfaces, 2018, 10, 18082–18092 CrossRef CAS PubMed.
- J. Zhong, D. Chen, S. Yuan, M. Liu, Y. Yuan, Y. Zhu, X. Li and Z. Ji, Inorg. Chem., 2018, 57, 8978–8987 CrossRef CAS PubMed.
- X. Huang, Nat. Photonics, 2014, 8, 748–749 CrossRef CAS.
- Y. Q. Li, N. Hirosaki, R. J. Xie, T. Takeda and M. Mitomo, Chem. Mater., 2008, 20, 6704–6714 CrossRef CAS.
- D. Cui, Q. Xiang, Z. Song, Z. Xia and Q. Liu, J. Mater. Chem. C, 2016, 4, 7332–7338 RSC.
- X. Huang, S. Wang, B. Li, Q. Sun and H. Guo, Opt. Lett., 2018, 43, 1307–1310 CrossRef CAS PubMed.
- Q. Bai, Z. Wang, P. Li, T. Li, S. Xu and Z. Yang, Luminescence, 2016, 31, 1277–1282 CrossRef CAS.
- C. Xia, C. Yu, M. Cao, J. Xia, D. Jiang, G. Zhou, D. Zhang and H. Li, Ceram. Int., 2018, 44, 21040–21046 CrossRef CAS.
- X. Pu, D. Zhang, H. Li, S. I. Kim, P. Cai, C. Chen and H. J. Seo, Phys. B, 2015, 472, 41–44 CrossRef CAS.
- L. Li, D. Zhang, X. Pu, J. Zhao, D. Li, C. Wang, S. Wu and T. Ge, Adv. Powder Technol., 2011, 22, 553–556 CrossRef CAS.
- J. Zhong, Y. Peng, D. Chen, M. Liu, X. Li, Y. Zhu and Z. Ji, J. Mater. Chem. C, 2018, 6, 13305–13315 RSC.
- Y. Zhu, L. Cao, M. G. Brik, X. Zhang, L. Huang, T. Xuan and J. Wang, J. Mater. Chem. C, 2017, 5, 6420–6426 RSC.
- S. Adachi, J. Lumin., 2018, 197, 119–130 CrossRef CAS.
- S. Adachi, J. Lumin., 2018, 202, 263–281 CrossRef CAS.
- H.-D. Nguyen, C. C. Lin, M.-H. Fanga and R.-S. Liu, J. Mater. Chem. C, 2014, 2, 10268–10272 RSC.
- M. Kim, W. B. Park, J.-W. Lee, J. Lee, C. H. Kim, S. P. Singh and K.-S. Sohn, Chem. Mater., 2018, 30, 6936–6944 CrossRef CAS.
- R. Cao, X. Liu, K. Bai, T. Chen, S. Guo, Z. Hu, F. Xiao and Z. Luo, J. Lumin., 2018, 197, 169–174 CrossRef CAS.
- J. Zhong, S. Zhou, D. Chen, J. Li, Y. Zhu, X. Li, L. Chen and Z. Ji, Dalton Trans., 2018, 47, 8248–8256 RSC.
- F. Xue, Y. Hu, L. Chen, H. Wu, G. Ju, T. Wang and L. Yang, Ceram. Int., 2017, 43, 15141–15145 CrossRef CAS.
- S. Liang, M. Shang, H. Lian, K. Li, Y. Zhang and J. Lin, J. Mater. Chem. C, 2016, 4, 6409–6416 RSC.
- X. Ding, Q. Wang and Y. Wang, Phys. Chem. Chem. Phys., 2016, 18, 8088–8097 RSC.
- D. Kim, S. Park, B. C. Choi, S. H. Park, J. H. Jeong and J. H. Kim, Mater. Res. Bull., 2018, 97, 115–120 CrossRef CAS.
- H. Chen, H. Lin, Q. Huang, F. Huang, J. Xu, B. Wang, Z. Lin, J. Zhou and Y. Wang, J. Mater. Chem. C, 2016, 4, 2374–2381 RSC.
- S. Wang, Q. Sun, B. Devakumar, L. Sun, J. Liang and X. Huang, RSC Adv., 2018, 8, 30191–30200 RSC.
- K. Sankarasubramanian, B. Devakumar, G. Annadurai, L. Sun, Y.-J. Zeng and X. Huang, RSC Adv., 2018, 8, 30223–30229 RSC.
- Q. Sun, S. Wang, B. Li, H. Guo and X. Huang, J. Lumin., 2018, 203, 371–375 CrossRef CAS.
- J. Liang, L. Sun, B. Devakumar, S. Wang, Q. Sun, H. Guo, B. Li and X. Huang, RSC Adv., 2018, 8, 31666–31672 RSC.
- R. Cao, Z. Shi, G. Quan, T. Chen, S. Guo, Z. Hu and P. Liu, J. Lumin., 2017, 188, 577–581 CrossRef CAS.
- X. Huang and H. Guo, Dyes Pigm., 2018, 152, 36–42 CrossRef CAS.
- Z. Zhou, J. Zheng, R. Shi, N. Zhang, J. Chen, R. Zhang, H. Suo, E. M. Goldys and C. Guo, ACS Appl. Mater. Interfaces, 2017, 9, 6177–6185 CrossRef CAS PubMed.
- Y. Zheng, H. Zhang, H. Zhang, Z. Xia, Y. Liu, M. S. Molokeev and B. Lei, J. Mater. Chem. C, 2018, 6, 4217–4224 RSC.
- J. Xiang, J. Chen, N. Zhang, H. Yao and C. Guo, Dyes Pigm., 2018, 154, 257–262 CrossRef CAS.
- Z. Lu, T. Huang, R. Deng, H. Wang, L. Wen, M. Huang, L. Zhou and C. Yao, Superlattices Microstruct., 2018, 117, 476–487 CrossRef CAS.
- A. Fu, A. Guan, D. Yu, S. Xia, F. Gao, X. Zhang, L. Zhou, Y. Li and R. Li, Mater. Res. Bull., 2017, 88, 258–265 CrossRef CAS.
- A. Fu, C. Zhou, Q. Chen, Z. Lu, T. Huang, H. Wang and L. Zhou, Ceram. Int., 2017, 43, 6353–6362 CrossRef CAS.
- K. Li, H. Lian and R. V. Deun, J. Lumin., 2018, 198, 155–162 CrossRef CAS.
- L. Wang, Q. Liu, K. Shen, Q. Zhang, L. Zhang, B. Song and C. Wong, J. Alloys Compd., 2017, 696, 443–449 CrossRef CAS.
- L. Li, W. Chang, W. Chen, Z. Feng, C. Zhao, P. Jiang, Y. Wang, X. Zhou and A. Suchocki, Ceram. Int., 2017, 43, 2720–2729 CrossRef CAS.
- Y. Takeda, H. Kato, M. Kobayashi, S. Nozawa, H. Kobayashi and M. Kakihana, J. Phys. Chem. C, 2017, 121, 18837–18844 CrossRef CAS.
- A. M. Srivastava, H. A. Comanzo, D. J. Smith, J. W. Choi, M. G. Brik, W. W. Beers and S. A. Payne, J. Lumin., 2019, 206, 398–402 CrossRef CAS.
- G. Zhou, X. Jiang, J. Zhao, M. Molokeev, Z. Lin, Q. Liu and Z. Xia, ACS Appl. Mater. Interfaces, 2018, 10, 24648–24655 CrossRef CAS PubMed.
- C. Yang, Z. Zhang, G. Hu, R. Cao, X. Liang and W. Xiang, J. Alloys Compd., 2017, 694, 1201–1208 CrossRef CAS.
- G. Hu, X. Hu, W. Chen, Y. Cheng, Z. Liu, Y. Zhang, X. Liang and W. Xiang, Mater. Res. Bull., 2017, 95, 277–284 CrossRef CAS.
- Y. Chen, K. Wu, J. He, Z. Tang, J. Shi, Y. Xu and Z. Liu, J. Mater. Chem. C, 2017, 5, 8828–8835 RSC.
- W. Lu, W. Lv, Q. Zhao, M. Jiao, B. Shao and H. You, Inorg. Chem., 2014, 53, 11985–11990 CrossRef CAS PubMed.
- R. Cao, W. Luo, H. Xu, Z. Luo, Q. Hu, T. Fu and D. Peng, Opt. Mater., 2016, 53, 169–173 CrossRef CAS.
- U. B. Humayoun, S. N. Tiruneh and D.-H. Yoon, Dyes Pigm., 2018, 152, 127–130 CrossRef.
- Q. Peng, R. Cao, Y. Ye, S. Guo, Z. Hu, T. Chen and G. Zheng, J. Alloys Compd., 2017, 725, 139–144 CrossRef CAS.
- L. Qin, S. Bi, P. Cai, C. Chen, J. Wang, S. I. Kim, Y. Huang and H. J. Seo, J. Alloys Compd., 2018, 755, 61–66 CrossRef CAS.
- H. Guo and X. Huang, J. Alloys Compd., 2018, 764, 809–814 CrossRef CAS.
- W. Chen, Y. Cheng, L. Shen, C. Shen, X. Liang and W. Xiang, J. Alloys Compd., 2018, 762, 688–696 CrossRef CAS.
- S. Zhang, Y. Hu, H. Duan, Y. Fu and M. He, J. Alloys Compd., 2017, 693, 315–325 CrossRef CAS.
- S. Zhang, Y. Hu, H. Duan, L. Chen, Y. Fu, G. Ju, T. Wang and M. He, RSC Adv., 2015, 5, 90499–90507 RSC.
- J. Zhong, X. Chen, D. Chen, M. Liu, Y. Zhu, X. Li and Z. Ji, J. Alloys Compd., 2019, 773, 413–422 CrossRef CAS.
- Q. Shao, H. Ding, L. Yao, J. Xu, C. Liang and J. Jiang, RSC Adv., 2018, 8, 12035–12042 RSC.
- H. Deng, Z. Gao, N. Xue, J. H. Jeong and R. Yu, J. Lumin., 2017, 192, 684–689 CrossRef CAS.
- X. Huang and H. Guo, RSC Adv., 2018, 8, 17132–17138 RSC.
- B. Li, S. Wang, Q. Sun, C. Lu, H. Guo and X. Huang, Dyes Pigm., 2018, 154, 252–256 CrossRef CAS.
- Z. Lu, A. Fu, F. Gao, X. Zhang and L. Zhou, J. Lumin., 2018, 203, 420–426 CrossRef CAS.
- G. Annadurai, B. Devakumar, H. Guo, R. Vijayakumar, B. Li, L. Sun, X. Huang, K. Wang and X. W. Sun, RSC Adv., 2018, 8, 23323–23331 RSC.
- J. Liang, P. Du, H. Guo, L. Sun, B. Li and X. Huang, Dyes Pigm., 2018, 157, 40–46 CrossRef CAS.
- J. Liang, L. Sun, B. Devakumar, S. Wang, Q. Sun, H. Guo, B. Li and X. Huang, RSC Adv., 2018, 8, 27144–27151 RSC.
- X. Huang, J. Liang, B. Li, L. Sun and J. Lin, Opt. Lett., 2018, 43, 3305–3308 CrossRef CAS PubMed.
- L. Shi, Y.-j. Han, H.-x. Wang, D.-c. Shi, X.-y. Geng and Z.-w. Zhang, J. Lumin., 2019, 208, 307–312 CrossRef CAS.
- L. Shi, Y.-j. Han, Z.-g. Zhang, Z.-x. Ji, D.-c. Shi, X.-y. Geng, H. Zhang, M. Li and Z.-w. Zhang, Ceram. Int., 2018 DOI:10.1016/j.ceramint.2018.11.166.
- L. Shi, Y.-j. Han, Z.-x. Ji and Z.-w. Zhang, J. Mater. Sci.: Mater. Electron., 2019 DOI:10.1007/s10854-018-00590-5.
- L. Shi, J.-x. Li, Y.-j. Han, W.-l. Li and Z.-w. Zhang, J. Lumin., 2019, 208, 201–207 CrossRef CAS.
- R. Cao, T. Chen, Y. Ren, T. Chen, H. Ao, W. Li and G. Zheng, J. Alloys Compd., 2019, 780, 749–755 CrossRef CAS.
- Q. Sun, S. Wang, B. Devakumar, B. Li, L. Sun, L. Jia, D. Chen and X. Huang, RSC Adv., 2018, 8, 39307–39313 RSC.
- L. Li, Y. Pan, Z. Chen, S. Huang and M. Wu, RSC Adv., 2017, 7, 14868–14875 RSC.
|
This journal is © The Royal Society of Chemistry 2019 |
Click here to see how this site uses Cookies. View our privacy policy here.