DOI:
10.1039/C9RA00004F
(Paper)
RSC Adv., 2019,
9, 11451-11458
Novel sulfonamidospirobifluorenes as fluorescent sensors for mercury(II) ion and glutathione†
Received
1st January 2019
, Accepted 5th April 2019
First published on 11th April 2019
Abstract
Novel spirobifluorene derivatives containing two and four sulfonamide groups are successfully synthesized from the commercially available bromo-9,9′-spirobifluorene by Sonogashira couplings. These compounds exhibit an excellent selective fluorescence quenching by Hg(II) in DMSO/HEPES buffer mixture with three-times-noise detection limits of 10.4 to 103.8 nM. A static aggregation induced quenching mechanism is proposed based on the data from 1H-NMR and UV-Vis spectroscopy, as well as the observation of the Tyndall effect. Quantifications of Hg(II) using these sensors are in good agreement with those obtained from ICP-OES. The reversibility of these sensors is demonstrated by a complete fluorescence restoration upon addition of EDTA or L-glutathione. The application as a turn-on sensor for L-glutathione is demonstrated in a quantitative analysis of three samples of L-glutathione supplement drinks.
1. Introduction
Mercury is a highly toxic metal element. The World Health Organization (WHO)1 has recommended reducing and eliminating the use of mercury in the environment because it causes several diseases such as Minamata disease2 and the risk of heart disease.3 However, mercury and its derivatives are used in medicinal,4 agricultural,5 and many other industrial applications.6 Hence, close monitoring of mercury and its ionic species that contaminate the environment is of great significance. Several techniques such as atomic absorption spectroscopy (AAS),7 atomic emission spectroscopy (AES),8 inductively coupled plasma-mass spectrometry (ICP-MS),9 and inductively coupled plasma-optical emission spectrometry (ICP-OES)10 are routinely used for the analysis of mercury. Even though these methods can provide high sensitivity, accuracy, and precision, they are not practical for real-time or on-site analysis. In addition, they require well-trained specialists and high instrument maintenance costs, which can be avoided using selective fluorescent recognition probes.
Recent examples of selective fluorescent probes for Hg(II) are the derivatives of triarylamine–triazine,11 rhodamine,12 bis-thiophene,13 and coumarin.14 During our research project on design and synthesis of selective fluorescent sensors, we became interested in spirobifluorene since it has high chemical and thermal stabilities with strong emission signal.15 Several derivatives of spirobifluorene are used as materials in optoelectronic devices such as field-effect transistors (FET),16 phototransistors,17 solar cells,18 and organic light-emitting diodes.19 There are a few examples of spirobifluorenes used as fluorescent chemosensors for analytes such as nitric oxide,20 H+,21 Ag(I),22 and Hg(II) ion.23 The sensing mechanism for Hg(II) is mostly involved specific hydrolysis reactions of thioacetals with Hg(II).24 Although these sensors exhibited excellent selectivity towards Hg(II), the irreversibility of hydrolysis reaction prevents further applications as a turn-on sensor for a secondary analyte.
In this work, we report our synthesis and application of two new derivatives of spirobifluorene named disulfonamidospirobifluorene (DSS) and tetrasulfonamidospirobifluorene (TSS) (Fig. 1). The sulfonamide groups are expected to function as specific binding sites for Hg(II).25 This reversible complex formation may allow an application of this system as a turn-on fluorescent sensor for a secondary analyte such as cysteine and L-glutathione that can form a more stable complex with Hg(II).26 L-Glutathione is a value-added ingredient in a variety of health supplements as it helps reduce oxidative stress,27 and symptoms of neurodegenerative diseases such as Alzheimer,28 and Parkinson's disease.29 The quantitative analysis of L-glutathione in health supplements by a competitive binding with Hg(II) in the DSS–Hg(II) complex will also be demonstrated.
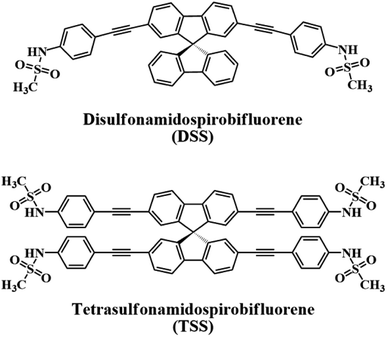 |
| Fig. 1 The structures of DSS and TSS. | |
2. Experimental
2.1 Chemicals and materials
All reagents and solvents were purchased from Merck (Germany), Sigma-Aldrich (USA), or Fluka (Switzerland). Unless stated otherwise, these chemicals were used without further purification. All column chromatography were operated using silica gel 60 (70–230 mesh) purchased from Merck. Water used in all spectroscopic experiments was deionized with a Milli-Q® reference water purification system (Merck) to a specific resistivity of 18.2 MΩ cm. The stock solutions of DSS and TSS were prepared in dimethyl sulfoxide (DMSO) at 1 mM and diluted further to obtain expected concentrations. The stock solutions of metal ions in deionized water were prepared at 10 mM using the following commercially available salts: LiNO3, NaNO3, KNO3, AgNO3, Ca(NO3)2, Mg(NO3)2, Ba(NO3)2, Co(NO3)2, Cd(NO3)2, Zn(NO3)2, Pb(NO3)2, Ni(NO3)2, Cu(NO3)2, Hg(OAc)2, Fe(OAc)2, Fe(NO3)3, Al(NO3)3, Bi(NO3)3, Cr(NO3)3, and AuCl3. The pH-controlled and buffer solutions in deionized water (10 mM) were NaCl/HCl buffer (pH 1–2), acetate buffer (pH 3–5), HEPES buffer (pH 6–8), and glycine/NaOH buffer (pH 9–10).
2.2 Instruments
1H and 13C NMR spectra were collected on a 400 MHz NMR spectrometer which operated at 400 MHz for 1H and 100 MHz for 13C (Bruker Company). Mass spectra were recorded on a Microflex MALDI-TOF mass spectrometer (Bruker Daltonics). High-resolution mass spectra were obtained from a triple quadrupole GC/MS (Agilent Technologies). Elemental analysis was obtained from a CHNS/O analyzer (Flash 2000, Thermo Scientific). Absorption spectra were obtained from a UV-2250 UV-Vis Spectrophotometer (SHIMADZU, Japan) and emission spectra were obtained from a Carry Eclipse Fluorescence Spectrophotometer (Agilent Technologies). The absolute fluorescence quantum yields and lifetimes were determined using FLS980 Spectrometer (Edinburgh Instruments). The pH buffers were measured from an Ohaus pH meter. Amounts of Hg(II) ion in water samples were verified by an inductively coupled plasma-optical emission spectrometer (ICP-OES) (iCAP 6500, Thermo Scientific).
2.3 Synthesis and characterizations
2.3.1 Synthesis of 2,7-di(trimethylsilylethynyl)-9,9′-spirobifluorene (2a). A mixture of 2,7-dibromo-9,9′-spirobifluorene (1a, 0.50 g, 1.05 mmol), Pd(PPh3)2Cl2 (74.0 mg, 0.11 mmol), CuI (40.0 mg, 0.21 mmol), PPh3 (55.6 mg, 0.21 mmol), and (n-Bu)4NBr (34.0 mg, 0.11 mmol) was dissolved in toluene (5 mL) and diisopropylamine (5 mL) under nitrogen atmosphere. After 15 minutes of stirring at ambient temperature, trimethylsilylacetylene (0.62 g, 6.33 mmol) was added dropwise, and the reaction was heated at 100 °C for 24 h. The crude was extracted with CH2Cl2 and 30% NH4Cl solution. The organic phase was washed deionized water and brine, dried over anhydrous Na2SO4, filtered, and concentrated under reduced pressure. The crude residue was purified by silica gel column chromatography with hexane as the eluent. A light-yellow solid of 2a was obtained in 0.51 g (95%). 1H-NMR (400 MHz, CDCl3) δ ppm 7.93 (d, J = 7.4 Hz, 1H), 7.83 (d, J = 7.8 Hz, 1H), 7.58 (d, J = 7.8 Hz, 1H), 7.45 (t, J = 7.3 Hz, 1H), 7.19 (t, J = 7.3 Hz, 1H), 6.95 (s, 1H), 6.79 (d, J = 7.4 Hz, 1H), 0.24 (s, 9H), 13C-NMR (100 MHz, CDCl3) δ ppm 149.2, 147.7, 141.9, 141.4, 132.1, 128.2, 128.1, 127.8, 124.3, 122.9, 120.28, 120.27, 105.4, 95.0, 65.7, 0.1. MS (MALDI-TOF) calcd. for C35H32Si2 ([M]+) 508.204, found 507.473.
2.3.2 Synthesis of 2,2′,7,7′-tetra(trimethylsilylethynyl)-9,9′-spirobifluorene (2b). A mixture of 2,2′,7,7′-tetrabromo-9,9′-spirobifluorene (1b, 0.50 g, 0.79 mmol), Pd(PPh3)2Cl2 (55.5 mg, 0.08 mmol), CuI (30.1 mg, 0.16 mmol), and PPh3 (41.5 mg, 0.16 mmol) was dissolved in toluene (5 mL) and diisopropylamine (5 mL) under nitrogen atmosphere. After 15 minutes of stirring at ambient temperature, trimethylsilylacetylene (0.47 g, 4.77 mmol) was added dropwise, and the reaction was heated at 100 °C for 24 h. The crude was extracted with CH2Cl2 and 30% NH4Cl solution. The organic phase was washed deionized water and brine, dried over anhydrous Na2SO4, filtered, and concentrated under reduced pressure. The crude residue was purified by silica gel column chromatography with hexane as the eluent. A white solid of 2b was obtained in 0.52 g (94%). 1H-NMR (400 MHz, CDCl3) δ ppm 7.77 (d, J = 7.9 Hz, 1H), 7.52 (d, J = 7.9 Hz, 1H), 6.81 (s, 1H), 0.18 (s, 9H), 13C-NMR (100 MHz, CDCl3) δ ppm 148.1, 141.4, 132.4, 127.8, 123.1, 120.4, 105.1, 95.3, 65.2, 0.0. MS (MALDI-TOF) calc. mass for C45H48Si4 ([M]+) 700.283, found 699.728.
2.3.3 Synthesis of 2,7-diethynyl-9,9′-spirobifluorene (3a). To a solution of 2a (0.50 g, 0.98 mmol) in CH2Cl2 (16 mL) was added a solution of NaOH (0.39 g, 9.83 mmol) in MeOH (4 mL) under nitrogen atmosphere. The reaction was stirred at room temperature for 15 h. The reaction mixture was extracted with CH2Cl2 and deionized water. The organic phase was washed brine, dried over anhydrous Na2SO4, filtered, and concentrated under reduced pressure. The crude mixture was purified by silica gel column chromatography with 5% CH2Cl2 in hexane as the eluent. A light-yellow solid of 3a was obtained in 0.36 g (100%). 1H-NMR (400 MHz, CDCl3) δ ppm 7.86 (d, J = 7.6 Hz, 1H), 7.80 (d, J = 7.9 Hz, 1H), 7.53 (d, J = 7.9 Hz, 1H), 7.40 (t, J = 7.5 Hz, 1H), 7.14 (t, J = 7.5 Hz, 1H), 6.89 (s, 1H), 6.73 (d, J = 7.6 Hz, 1H), 2.98 (s, 1H), 13C-NMR (100 MHz, CDCl3) δ ppm 149.4, 147.6, 141.9, 141.6, 132.2, 128.2, 128.1, 128.0, 124.2, 121.9, 120.4, 120.3, 83.9, 77.9, 65.7. MS (MALDI-TOF) calc. mass for C29H16 ([M]+) 364.125, found 363.221.
2.3.4 Synthesis of 2,2′,7,7′-tetraethynyl-9,9′-spirobifluorene (3b). To a solution of 2b (0.46 g, 0.65 mmol) in CH2Cl2 (16 mL) was added a solution of NaOH (0.26 g, 6.50 mmol) in MeOH (4 mL) under nitrogen atmosphere. The reaction was stirred at room temperature for 24 h. The reaction mixture was extracted with CH2Cl2 and deionized water. The organic phase was washed brine, dried over anhydrous Na2SO4, filtered, and concentrated under reduced pressure. The crude residue was purified by silica gel column chromatography with 5% CH2Cl2 in hexane as the eluent. A light-yellow solid of 3b was obtained in 0.27 g (100%). 1H-NMR (400 MHz, CDCl3) δ ppm 7.79 (d, J = 7.9 Hz, 1H), 7.54 (d, J = 7.9 Hz, 1H), 6.85 (s, 1H), 3.01 (s, 1H), 13C-NMR (100 MHz, CDCl3) δ ppm 148.0, 141.6, 132.6, 127.9, 122.2, 120.6, 83.7, 78.2, 65.2. MS (MALDI-TOF) calc. mass for C33H16 ([M]+) 412.125, found 411.361.
2.3.5 Synthesis of N-(4-iodophenyl)methanesulfonamide (CH3SO2NH-C6H4-I). 4-Iodoaniline (2.00 g, 9.13 mmol) was dissolved in CH2Cl2 (5 mL) and pyridine (0.81 mL, 10.04 mmol) at 0 °C, and methanesulfonyl chloride (0.88 mL, 11.41 mmol) was slowly added dropwise. The reaction was allowed to warm at room temperature and stirred for 15 h. The reaction mixture was extracted with CH2Cl2 and 3N NaOH solution three times. The combined aqueous phase was acidified by concentrated HCl, and a white solid of CH3SO2NH-C6H4-I was filtered, washed with distilled water, and dried under vacuum. The product was obtained in 2.59 g (95%). 1H-NMR (400 MHz, acetone-D6) δ ppm 8.68 (s, 1H), 7.69 (d, J = 8.6 Hz, 2H), 7.16 (d, J = 8.6 Hz, 2H), 3.02 (s, 3H), 13C-NMR (100 MHz, acetone-D6) δ ppm 139.3, 139.1, 122.9, 87.9, 39.5. HRMS (ESI) calc. mass for C7H7INO2S− ([M−H]−) 295.92423, found 295.94424.
2.3.6 Synthesis of disulfonamidospirobifluorene (DSS). A mixture of CH3SO2NH-C6H4-I (0.73 g, 2.47 mmol), Pd(PPh3)4 (95.1 mg, 0.08 mmol), CuI (15.6 mg, 0.08 mmol), and PPh3 (10.8 mg, 0.04 mmol) were dissolved in toluene (4 mL) and diisopropylamine (5 mL) under nitrogen atmosphere. After 15 minutes of stirring at room temperature, 3a (0.15 g, 0.41 mmol) that was dissolved in DMSO (1 mL) was dropped slowly via a syringe over a period of 5 minutes, and the reaction was heated at 50 °C for 24 h. The crude was extracted with CH2Cl2 and 30% w/v NH4Cl. The organic phase was washed deionized water and brine, dried over anhydrous Na2SO4, filtered, and concentrated under reduced pressure. The crude residue was purified by silica gel column chromatography with 60% ethyl acetate in hexane as the eluent. A light-brown solid of DSS was obtained in 0.20 g (69%). 1H-NMR (400 MHz, CDCl3) δ ppm 7.88 (d, J = 7.6 Hz, 1H), 7.82 (d, J = 7.9 Hz, 1H), 7.55 (d, J = 8.0 Hz, 1H), 7.41 (t, J = 7.5 Hz, 1H), 7.38 (d, J = 8.3 Hz, 2H), 7.33 (s, 1H), 7.16 (d, J = 8.0 Hz, 2H), 7.15 (t, J = 7.4 Hz, 1H), 6.92 (s, 1H), 6.77 (d, J = 7.5 Hz, 1H), 3.00 (s, 3H), 13C-NMR (100 MHz, CDCl3) δ ppm 149.4, 147.8, 141.9, 141.3, 136.8, 133.0, 131.7, 128.23, 128.17, 127.4, 124.3, 122.8, 120.4, 120.3, 120.03, 119.96, 90.2, 89.4, 65.8, 39.7. MS (MALDI-TOF) calc. mass for C43H30N2O4S2 ([M]+) 702.165, found 701.918. Elemental analysis (%) calc. for C43H30N2O4S2: C, 73.48; H, 4.30; N, 3.99. Found: C, 73.26; H, 4.48; N, 3.75.
2.3.7 Synthesis of tetrasulfonamidospirobifluorene (TSS). A mixture of CH3SO2NH-C6H4-I (0.86 g, 2.91 mmol), Pd(PPh3)4 (56.0 mg, 0.05 mmol), CuI (9.2 mg, 0.05 mmol), and PPh3 (12.7 mg, 0.05 mmol) were dissolved in toluene (4 mL), and diisopropylamine (4 mL) under nitrogen atmosphere. After 15 minutes of stirring at room temperature, 3b (0.10 g, 0.24 mmol) that was dissolved in DMSO (2 mL) was dropped slowly via a syringe over a period of 5 minutes. The reaction was heated at 50 °C for 48 h, allowed to cool to room temperature, and extracted with CH2Cl2 and 30% w/v NH4Cl. The organic phase was washed with deionized water and brine, dried over anhydrous Na2SO4, filtered, and concentrated under reduced pressure. The crude mixture was purified by silica gel column chromatography with 80% ethyl acetate in hexane as the eluent. A light-brown solid of TSS was obtained in 0.04 g (15%). 1H-NMR (400 MHz, acetone-D6) δ ppm 8.77 (s, 1H), 8.09 (d, J = 7.9 Hz, 1H), 7.60 (d, J = 7.9 Hz, 1H), 7.42 (d, J = 8.7 Hz, 2H), 7.28 (d, J = 8.7 Hz, 2H), 6.92 (s, 1H), 3.00 (s, 3H), 13C-NMR (100 MHz, acetone-D6) δ ppm 149.3, 142.2, 139.8, 133.5, 132.7, 127.5, 124.0, 122.1, 120.3, 119.2, 90.8, 89.8, 66.1, 39.8. MS (MALDI-TOF) calc. mass for C61H44N4O8S4 ([M]+) 1088.204, found 1089.066. Elemental analysis (%) calc. for C61H44N4O8S4: C, 67.26; H, 4.07; N, 5.14. Found: C, 67.01; H, 4.30; N, 4.99.
2.4 Quantum yield determinations
The excitation wavelengths for DSS and TSS were set at 360 nm and the integrated area of the emission signals was set from 370 to 600 nm. The signal rate on the instrument was set to 1 × 106 counts per second. The emission signals were detected on the integrating sphere and analyzed by F980 software (Edinburgh instrument).
3. Results and discussion
3.1 Synthesis
The synthesis of DSS and TSS (Scheme 1) began with a Sonogashira coupling between the commercially available brominated spirobifluorenes (1a, b). Both substrates were effectively coupled with trimethylsilylacetylene in excellent yields. After a treatment with NaOH in MeOH and CH2Cl2, the corresponding terminal alkyne 3a and 3b were produced in quantitative yields. In the last step, 3a and 3b were reacted with N-(4-iodophenyl)methanesulfonamide (CH3SO2NH-C6H4-I), which was prepared from 4-iodoaniline, to afford DSS and TSS in 69 and 15% yields, respectively. It should be noted that the polymerization of 3b was responsible for a diminished yield of TSS as a dark brown spot was observed on the TLC baseline, while the formation of mono-, di-, and tri-substituted products were not observed.
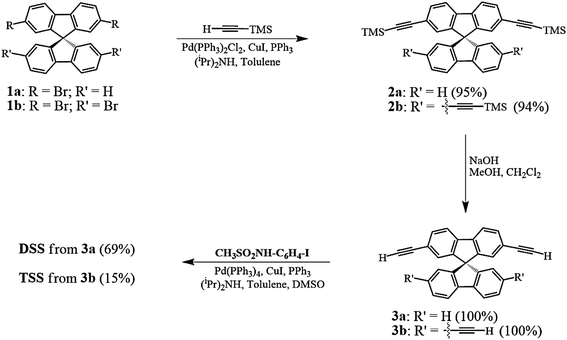 |
| Scheme 1 Synthesis of the DSS and TSS. | |
3.2 Photophysical properties
The UV-Vis absorption and emission spectra of DSS and TSS in DMSO are shown in Fig. 2, and the photophysical properties are summarized in Table 1. The maximum wavelengths of absorption and emission for both compounds were quite similar due to a relatively identical π-conjugated system between phenylacetylene and spirobifluorene units. However, the molar extinction coefficient for TSS is significantly higher than that of DSS because of a higher number of phenylacetylene units. The fluorescence quantum yield of DSS is significantly higher than that of TSS while their fluorescent lifetimes are relatively similar. These data suggest that the excited state of TSS has a higher tendency to lose energy via non-radiative proton transfer with DMSO.
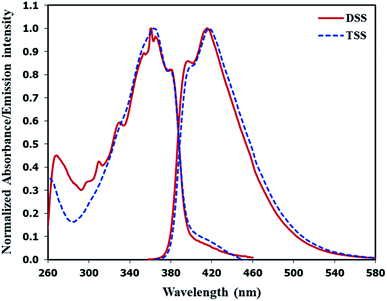 |
| Fig. 2 Normalized absorption and emission spectra of DSS and TSS in DMSO. | |
Table 1 Photophysical properties of DSS and TSS in DMSO
Cmpd. |
λabs (nm) |
ε (M−1cm−1) |
λema (nm) |
Φfb |
τc (ns) |
Excited at λabs. Absolute fluorescence quantum yield. Fluorescence lifetime. |
DSS |
361 |
26 900 |
415 |
0.85 |
0.74 |
TSS |
363 |
45 400 |
417 |
0.55 |
0.80 |
3.3 Solvent effect
The fluorescence spectra of DSS and TSS in various solvents are shown in Fig. S1.† The data suggested that DSS and TSS have good solubility in most solvents. In non-polar solvent like hexane and highly polar solvent like DMSO and water, a bathochromic shift and lower emission signals were detected. This could be rationale by their poor solubility in hexane and water or stabilization of excited states by DMSO. Even though the fluorescent signals are lower in DMSO compared to ethanol, methanol or acetonitrile, its high boiling point and low evaporating rate make it a suitable solvent for stock solution. Since the Hg(II) sensing applications are usually conducted in aqueous media, the optimal ratio of water and DMSO was investigated, and the results are shown in Fig. S2.† The fluorescence intensities of both compounds decreased upon the increment of water content, probably due to some aggregation or precipitation. Based on their emission intensity and UV-Vis absorbance in Fig. S3,† 50% and 30% seem like thresholds of water contents for further sensing studies of DSS and TSS, respectively.
3.4 Metal ion sensing studies
The selectivity screening was performed using stock solutions of DSS and TSS in DMSO diluted with HEPES buffer pH 7.0 and DMSO to achieve 10 μM solution of sensors. These solutions were treated with 10 mole equivalents of 20 metal ions including Li(I), Na(I), K(I), Ag(I), Ca(II), Mg(II), Ba(II), Co(II), Cd(II), Zn(II), Pb(II), Ni(II), Cu(II), Hg(II), Fe(II), Fe(III), Al(III), Bi(III), Cr(III), and Au(III). The results in Fig. 3 show that DSS and TSS have excellent selectivity towards Hg(II) ion with a 45- and 107-folds fluorescence quenching, respectively. The quantitative determination of Hg(II) was studied by fluorescence titration experiments. Linear plots were obtained in the Hg(II) concentrations between 0 to 0.1 μM for DSS and 0 to 1 μM for TSS (Fig. 4). The detection limits for Hg(II) at three-time noise were 10.4 nM for DSS and 103.8 nM for TSS. In addition, the interfering effect of foreign ions at 1 mM (10 times concentration of Hg(II)) was studied, and the results indicated that only Fe(II) can interfere the detection of Hg(II) (Fig. S4†). A competitive absorption mechanism was proposed since the UV-Vis spectra of Fe(II) completely overlapped with those of DSS and TSS (Fig. S5†).
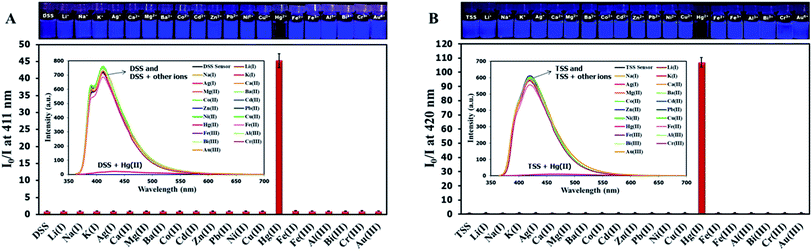 |
| Fig. 3 (A) Fluorescence responses of DSS (10 μM) and (B) TSS (10 μM) towards various metal ions (100 μM). | |
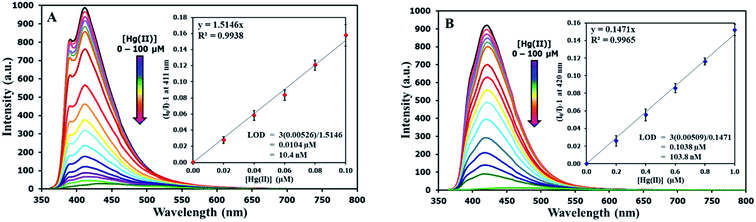 |
| Fig. 4 (A) Fluorescence responses of DSS (10 μM) and (B) TSS (10 μM) towards various concentrations of Hg(II). | |
3.5 Effect of pH
Because DSS and TSS contain acidic –NH groups, it is important to study the effect of pH on their fluorescence signals and sensitivity towards Hg(II). Data in Fig. S6† indicated that the original fluorescence signals of DSS and TSS were steady under acidic to neutral pH ranges and gradually decreased in basic media. Upon addition of Hg(II), the signals were decreased at all pHs, however, the quenching effect was most pronounced between 6.5 to 7.5. Therefore, the optimal working pH was selected at 7.0. The pH effect also provides a hint on the sensing mechanism which will be explained later.
3.6 Mechanism of sensing
Since the emission signals of both DSS and TSS were lowered under basic pHs, it is likely that the sensing mechanism may involve the deprotonation. For the investigation of sensing mechanism, we first examined the 1H-NMR spectra of DSS in D6-DMSO before and after the addition of 1 equivalent of Hg(II), and found that the –NH signal disappeared and the signal for the nearby aromatic –CH shifted slightly downfield when Hg(II) was added (Fig. 5). We postulated that the coordination between the sulfonamide and Hg(II) could promote a deprotonation of the –NH group at neutral pH. The addition of EDTA to DSS–Hg(II) and TSS–Hg(II) mixtures could restore the emission signals, which were completely recovered when the Hg(II)-EDTA stoichiometry reached 1
:
1 (Fig. S7†). In addition, lower absorbance and shifted baseline were observed in the UV-Vis spectra of DSS–Hg(II), along with a Tyndall effect (Fig. S8†). These results confirmed that the sensing mechanism involved a coordination between the sulfonamide group and Hg(II) that lead to an aggregation of such complex presumably via the face-to-face stacking of the spirobifluorene cores.30 This static binding quenching mechanism was ultimately proven by Stern–Volmer plots at different temperatures (Fig. S9†). The decreases in quenching efficiencies (lower KSV) at the higher temperature suggested that the formation of Hg(II) complex is responsible for signals quenching.
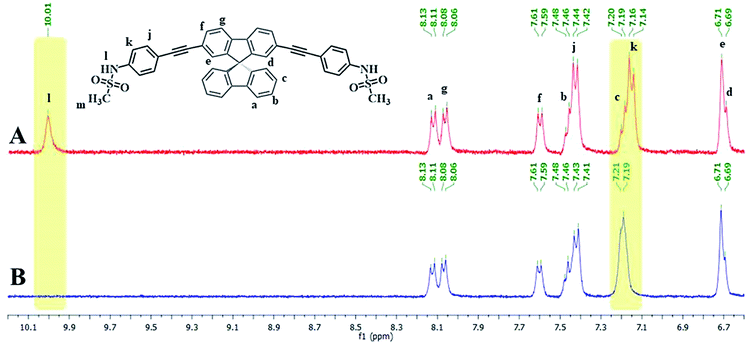 |
| Fig. 5 (A) 1H-NMR spectra of DSS and (B) DSS + Hg(OAc)2 (1.0 eq.) in D6-DMSO. | |
3.7 Quantitative analysis of Hg(II) in water samples
To demonstrate the use of DSS and TSS as sensors for Hg(II) in water, we selected 3 sources of water samples, spiked them with Hg(II), and analyzed the amount of Hg(II) using traditional ICP-OES technique and our sensors. The analysis results in Table 2 showed a good recovery of Hg(II) in each sample, while the statistical results in Table S1† indicated an insignificant difference between data from ICP-OES and our method (p-value > 0.05).
Table 2 Analytical results of Hg(II) in water samples (n = 3)
Samples |
Hg(II) added (ppm) |
ICP-OES |
DSS |
TSS |
Hg(II) found (ppm) |
Recovery (%) |
Hg(II) found (ppm) |
Recovery (%) |
Hg(II) found (ppm) |
Recovery (%) |
Rain water |
0.5 |
0.504 ± 0.032 |
101 |
0.482 ± 0.052 |
96 |
0.474 ± 0.040 |
95 |
1.0 |
1.118 ± 0.022 |
112 |
1.041 ± 0.048 |
104 |
1.101 ± 0.085 |
110 |
Tap water |
0.5 |
0.513 ± 0.030 |
103 |
0.506 ± 0.045 |
101 |
0.512 ± 0.067 |
102 |
1.0 |
1.185 ± 0.036 |
118 |
1.055 ± 0.058 |
105 |
1.116 ± 0.099 |
112 |
Bottled water |
0.5 |
0.571 ± 0.011 |
114 |
0.547 ± 0.037 |
109 |
0.565 ± 0.032 |
113 |
1.0 |
1.082 ± 0.019 |
108 |
1.022 ± 0.028 |
102 |
1.024 ± 0.071 |
102 |
3.8 Detection of L-glutathione
There have been several reports on specific binding between Hg(II) and L-glutathione, which is a biologically important antioxidant and a major component in food supplements and cosmetics. In our study, we tested the ability of L-glutathione and other food ingredients to restore the fluorescence signal of DSS, and found that the DSS–Hg(II) complex can exhibit a selective fluorescence turn-on by L-glutathione (Fig. 6, S10†). Ascorbic acid and its sodium salt, which are known to coordinate with Hg(II), can also restore the fluorescence signal, albeit in lower levels. However, the amount of ascorbic acid in most samples is usually 10-folds lower than L-glutathione. Quantitative analysis revealed a detection limit of about 0.6 μM or approximately 200 ppb of L-glutathione (Fig. 7). The applications of DSS for analyses of L-glutathione on three supplement beverages showed good agreements between the labeled and experimental contents (Table 3).
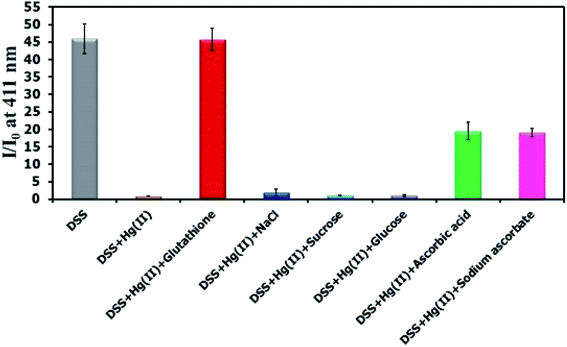 |
| Fig. 6 Fluorescence response of DSS (10 μM) with Hg(II) (100 μM) and other beverage ingredients (300 μM). | |
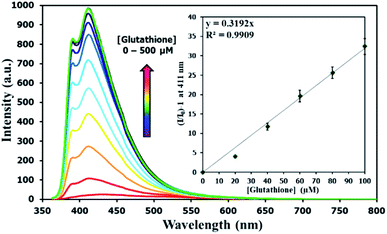 |
| Fig. 7 Fluorescence responses of DSS (10 μM) with Hg(II) (100 μM) upon addition of L-glutathione. | |
Table 3 Analytical results of L-glutathione in three commercial beverages (n = 3)
Samples |
Labeled content (%) |
Analyzed by DSS–Hg(II) |
Found (%) |
Recovery (%) |
1 |
0.05 |
0.0512 ± 0.0042 |
102 |
2 |
0.10 |
0.1102 ± 0.0090 |
110 |
3 |
0.30 |
0.2946 ± 0.0128 |
98 |
4. Conclusion
In summary, we successfully synthesized two derivatives of spirobifluorene containing methanesulfonamide groups. Both compounds showed a selective fluorescence quenching by Hg(II) via complexation and aggregation-caused quenching. The detection limits for Hg(II) were 10.4 nM and 103.8 nM for the derivatives bearing two and four sulfonamide groups, respectively. One of these mercury complexes was used as a selective turn-on sensor for L-glutathione with a detection limit of 0.6 μM. A satisfactory quantitative analysis of L-glutathione in three supplement drinks was also demonstrated.
Conflicts of interest
There are no conflicts to declare.
Acknowledgements
KS thanks the Graduate School, Chulalongkorn University for the 72nd Anniversary of His Majesty King Bhumibol Adulyadej and the 90th Anniversary of Chulalongkorn University Funds (Ratchadaphiseksomphot Endowment Fund). This work is supported by the Nanotechnology Center (NANOTEC), NSTDA, Ministry of Science and Technology, Thailand, through its program on Research Network NANOTEC (RNN), and the Grant for International Research Integration: Chula Research Scholar. We also thank Prof. Kanet Wongravee for his help on statistical analysis, Prof. Vinich Promarak and Mr Phatananawee Nalaoh of VISTEC for their helps on fluorescence quantum yield and lifetime measurements, and the Sci. Spec. Company Limited for the ICP-OES experiments.
References
- WHO, Exposure to Mercury: A Major Public Health Concern, Preventing Disease through Healthy Environments, World Health Organization, Geneva, 2007, http://www.who.int/phe/news/Mercury-flyer.pdf, (accessed July, 2018) Search PubMed.
- N. Hachiya, J. Jpn. Med. Assoc., 2006, 49, 112–118 Search PubMed.
- T. J. Larsen, M. E. Jørgensen, C. V. L. Larsen, I. K. Dahl-Petersen, P. F. Rønn, P. Bjerregaard and S. Byberg, Environ. Res., 2018, 164, 310–315 CrossRef CAS PubMed.
- J. Aaseth, B. Hilt and G. Bjørklund, Environ. Res., 2018, 164, 65–69 CrossRef CAS PubMed.
- Y. Zhou, M. Aamir, K. Liu, F. Yang and W. Liu, Environ. Pollut., 2018, 240, 116–124 CrossRef CAS PubMed.
-
(a) Y. Wang, T. Tian, W. Zang and X. Jian, Procedia Environ. Sci., 2016, 31, 432–439 CrossRef;
(b) S. Kim, Y. Eom and T. G. Lee, J. Ind. Eng. Chem., 2018, 61, 288–294 CrossRef CAS.
- T. Lech, Forensic Sci. Int., 2014, 237, e1–e5 CrossRef CAS PubMed.
- R. Shekhar, Talanta, 2012, 93, 32–36 CrossRef CAS PubMed.
- S. Zhu, B. Chen, M. He, T. Huang and B. Hu, Talanta, 2017, 171, 213–219 CrossRef CAS PubMed.
- A. E. Fathabad, N. Shariatifar, M. Moazzen, S. Nazmara, Y. Fakhri, M. Alimohammadi, A. Azari and A. M. Khaneghah, Food Chem. Toxicol., 2018, 115, 436–446 CrossRef CAS PubMed.
- H. Zhang, Y. Qu, Y. Gao, J. Hua, J. Li and B. Li, Tetrahedron Lett., 2013, 54, 909–912 CrossRef CAS.
-
(a) Y. Yan, G. Ding and H. Xu, J. Ind. Eng. Chem., 2015, 25, 73–77 CrossRef CAS;
(b) Y. Jiao, L. Zhang and P. Zhou, Talanta, 2016, 150, 14–19 CrossRef CAS PubMed.
- Q. Niu, X. Wu, T. Li, Y. Cui, S. Zhang and X. Li, Spectrochim. Acta, Part A, 2016, 163, 45–48 CrossRef CAS PubMed.
- J. Chen, W. Liu, Y. Wang, H. Zhang, J. Wu, H. Xu, W. Ju and P. Wang, Tetrahedron Lett., 2013, 54, 6447–6449 CrossRef CAS.
- T. P. I. Saragi, T. Spehr, A. Siebert, T. Fuhrmann-Lieker and J. Salbeck, Chem. Rev., 2007, 107, 1011–1065 CrossRef CAS PubMed.
- C. Isenberg, T. Reichert and T. P. I. Saragi, Synth. Met., 2015, 208, 29–34 CrossRef CAS.
- T. P. I. Saragi, K. Onken, I. Suske, T. Fuhrmann-Lieker and J. Salbeck, Opt. Mater., 2007, 29, 1332–1337 CrossRef CAS.
- M.-D. Zhang, D.-X. Zhao, L. Chen, N. Pan, C.-Y. Huang, H. Cao and M.-D. Chen, Sol. Energy Mater. Sol. Cells, 2018, 176, 318–323 CrossRef CAS.
- P. Wu, J. Zhu, Z. Zhang, D. Dou, H. Wang, B. Wei and Z. Wang, Dyes Pigm., 2018, 156, 185–191 CrossRef CAS.
- L.-Y. Lin, X.-Y. Lin, F. Lin and K.-T. Wong, Org. Lett., 2011, 13, 2216–2219 CrossRef CAS PubMed.
- Z. Han, J. Tan, T. Wei, Y. Zhang, H. Xiao, L. Xu and B. He, Sens. Actuators, B, 2018, 255, 2290–2297 CrossRef CAS.
- H. Xiao, Y. Zhang, W. Zhang, S. Li and R. Xu, Sens. Actuators, B, 2016, 233, 469–475 CrossRef CAS.
- B. Yang and W. Wu, Anal. Methods, 2013, 5, 4716–4722 RSC.
-
(a) H. Xiao, Y. Zhang, S. Li, W. Zhang, Z. Han, J. Tan, S. Zhang and J. Du, Sens. Actuators, B, 2016, 236, 233–240 CrossRef CAS;
(b) H. Xiao, Y. Zhang, W. Zhang, S. Li, J. Tan and Z. Han, Mater. Chem. Phys., 2017, 192, 268–273 CrossRef CAS.
- H.-W. Li, B. Wang, Y.-Q. Dang, L. Li and Y. Wu, Sens. Actuators, B, 2010, 148, 49–53 CrossRef CAS.
-
(a) F. Jalilehvand, B. O. Leung, M. Izadifard and E. Damian, Inorg. Chem., 2006, 45, 66–73 CrossRef CAS PubMed;
(b) V. Mah and F. Jalilehvand, Chem. Res. Toxicol., 2010, 23, 1815–1823 Search PubMed.
- N. Dickerhof, J. F. Pearson, T. S. Hoskin, L. J. Berry, R. Turner, P. D. Sly and A. J. Kettle, Free Radicals Biol. Med., 2017, 113, 236–243 CrossRef CAS PubMed.
- C. B. Pocernich and D. A. Butterfield, Biochim. Biophys. Acta, 2012, 1822, 625–630 CrossRef CAS PubMed.
- M. Smeyne and R. J. Smeyne, Free Radicals Biol. Med., 2013, 62, 13–25 CrossRef CAS PubMed.
- S. Thiery, B. Heinrich, B. Donnio, C. Poriel and F. Camerel, J. Phys. Chem. C, 2015, 119, 10564–10575 CrossRef CAS.
Footnote |
† Electronic supplementary information (ESI) available. See DOI: 10.1039/c9ra00004f |
|
This journal is © The Royal Society of Chemistry 2019 |
Click here to see how this site uses Cookies. View our privacy policy here.