DOI:
10.1039/C9RA01427F
(Paper)
RSC Adv., 2019,
9, 10320-10325
Facile preparation of low-cost HKUST-1 with lattice vacancies and high-efficiency adsorption for uranium
Received
25th February 2019
, Accepted 26th March 2019
First published on 2nd April 2019
Abstract
In this work, we prepared HKUST-1 and HKUST-1 with lattice vacancies (HLV) using benzoic acid (BA) as a low-cost modulator to replace part of the traditional trimesic acid ligand (H3BTC). The structure and morphology of the products were characterized by FTIR, XRD, SEM and XPS. The adsorption performance of the products for uranium from aqueous solutions was investigated. The results showed that the sorption of U(VI) on HKUST-1 and HLV agreed with the Langmuir isotherm model (RHKUST-12 = 0.9867 and RHLV2 = 0.9828) and the maximum adsorption capacity was 430.98 mg g−1 and 424.88 mg g−1, respectively. According to kinetics studies, the adsorption fitted better with a pseudo-second-order model (RHKUST-12 = 1.0000 and RHLV2 = 0.9978). The as-prepared adsorbents were used for the removal of uranium from real water samples as well. The results showed that HLV with lower cost is a promising adsorbent for uranium from aqueous solutions.
1. Introduction
Nowadays, there is a growing interest in the development and applications of various nanostructured materials in many fields.1 Various materials including organic complexes,2 inorganic nanocomposites,3,4 and polyzwitterions/anions5 have been applied for contamination removal. Recently, metal–organic frameworks (MOFs) have become a new promising nanostructured material. MOFs are crystalline porous materials comprised of metal ions linked by various organic bridges. MOFs with large specific surface areas, high porosity, tuneable pore sizes and adjustable internal surfaces have been widely applied in various fields, such as gas storage and separation,6,7 catalysis,8,9 supercapacitor fabrication10 and adsorption of undesirable materials.11–13 Among various wastewater treatment technologies adsorption is considered the best and most universal technique for the removal of a wide variety of organic and inorganic pollutants.14 Because of its excellent chemical and thermal stability,15,16 HKUST-1 is a typical material among MOFs and has been intensively investigated for the removal of various pollutants, such as H2S,17 Pb(II),18 dyes,19,20 Sr2+,21 Ni(II),22 As(V),23 Cr(VI),24 hydroquinone,25 fluoroquinolone,26 pesticides27,28 and uranium.29,30
In general, trimesic acid (H3BTC) has been used as the traditional ligand to prepare HKUST-1 in the previous reports. However, the cost of H3BTC (the cost of analytical grade product is currently 566 ¥/500 g) is much higher than that of benzoic acid (BA) with less –COOH groups (the cost of analytical grade product is currently 16.4 ¥/250 g). The preparation cost of HKUST-1 will greatly reduce by using BA as the ligand to replace part of H3BTC. To the best of our knowledge, no reports have been published about the preparation and adsorption capacity of HKUST-1 using low-cost BA as the modulator for uranium from aqueous solutions. Therefore, to reduce significantly the preparation cost of HKUST-1, we used BA to replace part of the traditional H3BTC ligand to obtain HKUST-1 with lattice vacancies (HLV). Moreover, the as-synthesized HKUST-1 and HLV in the present work have another two advantages: (1) the removal rate of uranium using HKUST-1 and HLV reached 99% even for the solutions bearing a high concentration of uranium (100 mg L−1), and (2) the maximum adsorption capacity of HKUST-1 and HLV were up to about 431 and 425 mg g−1, respectively, which was higher than most of the reported references (see Table 3). Herein, we prepared HKUST-1 and HLV by a simple hydrothermal synthesis method for the adsorption of uranium from aqueous solutions. The influence of solution pH, co-exiting ions, contact time, and initial U(VI) concentration on the adsorption capacity was studied. The adsorption isotherms and kinetics models were also investigated.
2. Materials and methods
2.1. Materials
Stock solutions of uranium (5–100 mg L−1) were prepared by dissolving UO2(NO3)2·6H2O (Xi'an Dingtian Chemical Reagent Co.) in deionized water (DW) and acidified with a small amount of concentrated HNO3. All the chemicals, i.e., Cu(NO3)2·3H2O, H3BTC, BA and absolute ethanol, were of analytical grade and used without further purification. DW was used throughout the experiments.
2.2. Preparation of HKUST-1 and HLV
The preparation method of HKUST-1 and HLV was modified according to the ref. 31. In a typical synthesis of HKUST-1, 1.0870 g of Cu(NO3)2·3H2O was dissolved in 15 ml DW, while 0.5250 g of H3BTC was dissolved in 15 ml absolute ethanol. Both solutions were mixed and stirred for 30 min. The resulting mixture was transferred into a Teflon autoclave and heated in an oven at 110 °C for 24 h. The resultant blue crystals were filtered under vacuum and washed with ethanol and DW (v
:
v = 1
:
1) and ethanol. Then, the products were immersed in ethanol for 36 h and ethanol was replaced every 12 h. Finally, the products were filtered under vacuum and dried at 100 °C under vacuum.
In a typical synthesis of HLV, 1.0870 g of Cu(NO3)2·3H2O was dissolved in 15 ml DW. The mixture of H3BTC and BA with molar ratio 3
:
1 was dissolved in 15 ml absolute ethanol. Then, both solutions were transferred into a Teflon autoclave and heated in an oven at 110 °C for 24 h. The resultant blue crystals were filtered under vacuum and washed with a solution of ethanol and DW (v
:
v = 1
:
1) and ethanol. Then, the products were immersed in ethanol for 36 h and ethanol was replaced every 12 h. Finally, the products were filtered under vacuum and dried at 100 °C under vacuum.
2.3. Characterization of the products
The Fourier transform infrared (FTIR) spectra of the as-prepared adsorbents were obtained using an FTIR spectrometer (Bruker VERTEX 70, Germany). The crystal phases of the samples were characterized by X-ray diffraction (XRD) pattern (Dandong Fangyuan DX-2700 model, China). The surface morphology of the products was determined using scanning electron microscopy (SEM) (FEI Helios 600i, USA). X-ray photoelectron spectroscopy (XPS) was used to analysis the elemental content of HKUST-1 and HLV using an ESCALAB 250 X-ray photoelectron spectrometer (Thermo Fisher, USA).
2.4. Adsorption tests
The influences of pH, contact time and initial uranium concentration on the removal efficiency of uranium were investigated by batch adsorption experiments. The solution pH was adjusted by NaOH and HCl. The as-prepared adsorbent was added to a 20 ml U(VI) solution and shaken in a shaker (Kangshi, China). After filtration, residual uranium concentrations were measured by a micro-quantity uranium analyser (MUA model, China). The removal rate R (%) and the adsorption capacity of U(VI) Q (mg g−1) were calculated according to eqn (1) and (2), respectively. |
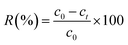 | (1) |
|
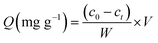 | (2) |
where c0 (mg L−1) is the initial U(VI) concentration, ct (mg L−1) is U(VI) concentration at time t, V (L) is the solution volume and W (g) is the weight of adsorbent.
3. Results and discussion
3.1. Characterization of the products
The functional group structures of HKUST-1 and HLV were determined by FTIR and are shown in Fig. 1. The characteristic peak at 1370 cm−1 was assigned to the C–O of H3BTC, and the bands at 1448 cm−1 and 1549 cm−1 were attributed to the C
O of H3BTC. The characteristic peak at 1647 cm−1 resulted from aromatic C
C of H3BTC. This result is in accordance with a previous study.31,32 However, in the HLV FTIR spectrum, the intensity of the H3BTC characteristic peaks at 1448 cm−1 and 1549 cm−1 attributed to C
O and the –OH group at >3000 cm−1 decreased significantly because part of the H3BTC was replaced by BA in the HLV, which has fewer –COOH groups than H3BTC. Moreover, by XPS elemental analysis the calculated atomic ratio of C
:
Cu
:
O in HKUST-1 and HLV is 8.37
:
1
:
4.73 and 7.22
:
1
:
4.25, respectively, which indicated that the part of H3BTC was replaced by BA with less –COOH groups in HLV.
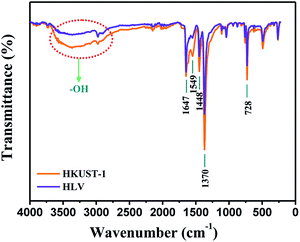 |
| Fig. 1 FTIR spectra of HKUST-1 and HLV. | |
The XRD patterns of the resulting HKUST-1 and HLV are shown in Fig. 2. All of the diffraction peaks of HKUST-1 were in accordance with those reported in the ref. 29, and the indexed values of all of the diffraction peaks were accordance with the reference.33–35 Fig. 2 shows that the crystalline structure of HKUST-1 was similar to that of HLV. No obvious impurity peaks can be detected in the XRD patterns of HKUST-1 and HLV. The results showed that HKUST-1 and HLV had good chemical stability and high crystallinity even if the part of H3BTC was replaced by BA.36
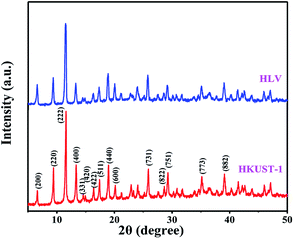 |
| Fig. 2 XRD patterns of HKUST-1 and HLV. | |
The morphology of HKUST-1 and HLV are shown in Fig. 3. In the SEM of HKUST-1, the crystal sizes of tens of microns (Fig. 3a) were observed. Some of the particles were octahedra with clear edges, and the other particles were flower-like (Fig. 3b). Due to the replacement by BA, the pores in HLV were deeper than those in HKUST-1.
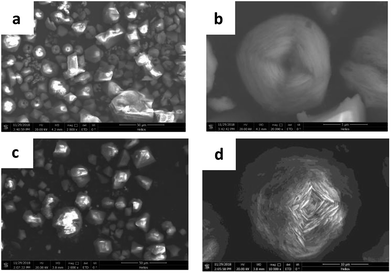 |
| Fig. 3 SEM of HKUST-1 ((a) 2000× and (b) 20 000×) and HLV ((c) 2000× and (d) 10 000×). | |
3.2. Effect of pH and co-existing ions on adsorption
The adsorption of U(VI) by HKUST-1 and HLV as a function of pH was carried out over the pH range of 3.0–8.0 for 30 min, as shown in Fig. 4, and the effect of co-existing ions on U(VI) adsorption was shown in Fig. 4 (inset). The results showed a significant impact of pH on uranium adsorption. The highest removal rate of U(VI) was observed at pH 4.0 and was found to be nearly 100%. The observed lower removal efficiency of U(VI) at pH < 3 may be attributed to formation of repulsive force between the protonated adsorbent and the positively charged uranyl ions which hindered the mass transfer and their adsorption onto the adsorbent. With the increase of pH deprotonation of HKUST-1 and HLV cause enhance in the complex formation and improvement in mass transfer to the adsorbent surface.37,38 However, at pH > 5 the interaction of U(VI) with HKUST-1 and HLV decreased due to the formation of uranyl species with low adsorption affinities, such as [UO2OH]+, [(UO2)3(OH)4]2+, [(UO2)3(OH)5]+, [(UO2)2(OH)2]2+, [(UO2)2OH]3+, [(UO2)3(OH)]5+, and [(UO2)4(OH)]7+.39 Therefore, the optimum pH of HKUST-1 and HLV for U(VI) adsorption was 4.0. Moreover, the effect of co-existing ions (K+, Mg2+, Ca2+, Al3+ and Sr2+) on U(VI) sorption is examined. As shown in Fig. 4 (inset), some co-existing ions (such as Mg2+ and Ca2+) had significantly effect on the adsorption efficiency for U(VI) by HLV at pH 4 while some ions (such as Al3+ and Sr2+) had no effect on the adsorption efficiency for U(VI).
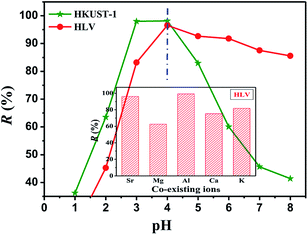 |
| Fig. 4 Effect of pH on U(VI) adsorption by HKUST-1 and HLV; the inset is the effect of co-existing ions on the removal of U(VI) by HLV. C(U)initial = 10 mg L−1, m/V = 0.25 g L−1, and contact time = 30 min. | |
3.3. Influence of contact time and adsorption kinetics study
Fig. 5a shows the effect of contact time on uranium adsorption by HKUST-1 and HLV. The adsorption efficiency of HKUST-1 and HLV improved significantly with time and reached adsorption equilibrium within 30 min. The removal rates of uranium of HKUST-1 and HLV were up to ∼99%. The linear fit of pseudo-first-order and pseudo-second-order kinetic models for U(VI) adsorption by HKUST-1 and HLV is shown in Fig. 5b and c, and the calculated fit parameters according to eqn (3) and (4) 40 are given in Table 1. The correlation coefficient of the pseudo-second-order model (R2 = 1.0000 for HKUST-1 and R2 = 0.9978 for HLV) was superior to that of the pseudo-first-order model, which indicated that the adsorption of U(VI) onto HKUST-1 and HLV fit the pseudo-second-order model well. The fit results demonstrated that the chemical interactions played a significant role in the rate-controlling steps. The equations are as follows: |
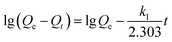 | (3) |
|
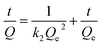 | (4) |
where k1 (min−1) is the Lagergren rate constant of adsorption and k2 (g (mg−1 min−1)) is the rate constant of pseudo-second-order adsorption.
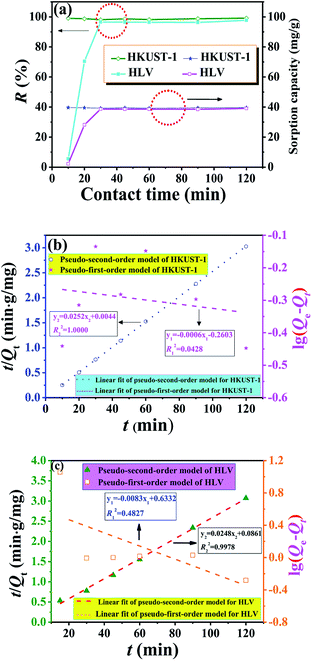 |
| Fig. 5 (a) Influence of contact time on U(VI) adsorption, (b) kinetics model fits of HKUST-1 and (c) kinetics model fits of HLV. pH = 4.0, C(U)initial = 10 mg L−1, and m/V = 0.25 g L−1. | |
Table 1 Parameters of pseudo-first-order and pseudo-second-order kinetic models for U(VI) adsorption by HKUST-1 and HLV. pH = 4.0, C(U)initial = 10 mg L−1, m/V = 0.25 g L−1
Sorbents |
Pseudo-first-order model |
Pseudo-second-order model |
Qe (mg g−1) |
k1 (min−1) |
R2 |
Qe (mg g−1) |
k2 (g (mg−1 min−1)) |
R2 |
HKUST-1 |
0.5492 |
0.0014 |
0.0428 |
39.6825 |
0.1443 |
1.0000 |
HLV |
4.2973 |
0.0191 |
0.4827 |
40.3226 |
0.0071 |
0.9978 |
3.4. Adsorption isotherm
The Langmuir and Freundlich isotherm models are expressed in eqn (5) and (6),40 respectively, and the calculated Langmuir and Freundlich isotherm fit data of HKUST-1 and HLV are shown in Table 2. It is clear from Fig. 6 that the equilibrium adsorption for HKUST-1 and HLV fit well to the Langmuir model, and the correlation coefficients of HKUST-1 and HLV were 0.9867 and 0.9828, respectively. The maximum adsorption capacity (Qm) of HKUST-1 and HLV for U(VI) reached 430.98 mg g−1 and 424.88 mg g−1, respectively. Though HLV has more lattice vacancies than that of HKUST-1 because of the replacement by BA, the Qm of HKUST-1 was higher than that of HLV, which showed that the adsorption mechanism was mainly the surface complexion and not the increase of active sites. |
 | (5) |
where Qe (mg g−1) is the equilibrium adsorption capacity, Ce (mg L−1) is the uranium concentration at equilibrium, Qm (mg g−1) is the maximum adsorption capacity, KL (L mg−1) and KF (mg1−n Ln g−1) are the Langmuir constant and Freundlich constant, respectively, and n is the Freundlich adsorption exponent.
Table 2 Langmuir and Freundlich model parameters for uranium adsorption on HKUST-1 and HLV. pH = 4.0, C(U)initial = 10 mg L−1, m/V = 0.25 g L−1 and contact time = 24 h
Sorbents |
Langmuir model |
Freundlich model |
Qm (mg g−1) |
kL (L mg−1) |
R2 |
n |
kF (mg1−n Ln g−1) |
R2 |
HKUST-1 |
430.98 |
3.9203 |
0.9867 |
2.88 |
292.13 |
0.8408 |
HLV |
424.88 |
2.9348 |
0.9828 |
2.84 |
265.30 |
0.8972 |
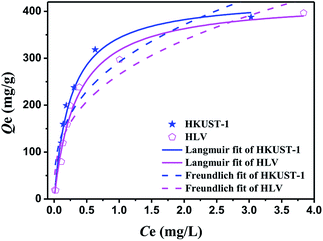 |
| Fig. 6 Linear fit of Langmuir and Freundlich isotherm models of HKUST-1 and HLV. pH = 4.0, C(U)initial = 5–100 mg L−1, m/V = 0.25 g L−1, and contact time = 24 h. | |
A comparison of Qm of HKUST-1 and HLV in this work and the reported other adsorbents is presented in Table 3. Table 3 shows that as-prepared low-cost HLV had higher Qm than most of the reported adsorbents and proved to be a promising adsorbent for the treatment of uranium-bearing wastewater.
Table 3 Maximum adsorption capacity of various adsorbents for U(VI) ions
Sorbents |
pH |
m/V (g L−1) |
Qm (mg g−1) |
References |
Modified aluminosilica |
3.5 |
1.0 |
83.30 |
39 |
Modified red muds |
3.0 |
3.5 |
124.56 |
41 |
Br-PADAP-impregnated MWCNTs |
6.3 |
0.1 |
83.40 |
42 |
MNPs@PAO |
6.0 |
0.5 |
216.45 |
43 |
Polypyrrole |
5.0 |
1.0 |
87.72 |
44 |
Pseudomonas monteilii |
6.0 |
0.3 |
267.30 |
45 |
AO-MWCNTs |
5.0 |
1.0 |
67.90 |
46 |
Tetraphenylimidodiphosphinate |
4.5 |
0.5 |
99.86 |
47 |
HKUST-1 |
6.0 |
0.4 |
787.40 |
29 |
HKUST-1@H3PW12O40 |
6.0 |
0.2 |
14.58 |
30 |
HKUST-1 |
4.0 |
0.25 |
430.98 |
This work |
HLV |
4.0 |
0.25 |
424.88 |
This work |
3.5. Adsorption efficiency for real wastewater samples
Under the optimum adsorption conditions, the removal efficiency of U(VI) by HKUST-1 and HLV for low-level uranium-bearing real wastewater from five different batches was evaluated. Some micro-quantity metal ions (e.g., Al, B, Ca, Be, Fe, Cu, Mn, Mg, Si, Ni and Mo) were found to exist in the real wastewater samples. All elements in real samples were analyzed by inductively coupled plasma-atomic emission spectrometry (ICP-AES) (Thermo Fisher iCAP 6300, USA). The adsorption experiments results are presented in Fig. 7. As shown in Fig. 7, HKUST-1 and HLV had favourable adsorption capacity for high-level uranium-bearing wastewater (>1.0 mg L−1), and the co-existing ions had no effect on the removal efficiency for uranium. However, the uranium concentration after treatment with HKUST-1 and HLV was not significantly reduced when the uranium concentration was very low.
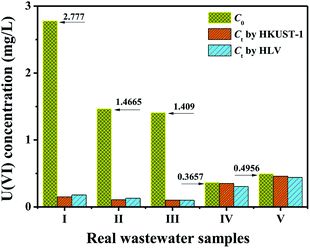 |
| Fig. 7 Column chart of the adsorption efficiency of HKUST-1 and HLV for real uranium-bearing nuclear waste effluents. pH = 4.0, C(U)initial = 0.3–3.0 mg L−1, m/V = 0.25 g L−1, and contact time = 30 min. | |
4. Conclusions
In this work, HKUST-1 and HLV were synthesized in a facile manner by hydrothermal methods and exhibited high-efficiency adsorption capacity for uranium from aqueous solutions, especially high-level uranium-containing wastewater. The optimal adsorption conditions included a pH of 4.0, 0.25 g L−1 of adsorbent dose and 30 min of contact time when the initial uranium concentration was 10 mg L−1. The effect of co-existing ions (K+, Mg2+, Ca2+, Al3+ and Sr2+) on the uranium removal by HLV was studied. Results showed that some co-existing ions (such as Mg2+ and Ca2+) had significantly effect on the adsorption efficiency for U(VI) by HLV at pH 4 while some ions (such as Al3+ and Sr2+) had no effect on the adsorption efficiency for U(VI). The adsorption processes of HKUST-1 and HLV were well described by the Langmuir isotherm model and pseudo-second-order kinetic model. The maximum adsorption capacities of HKUST-1 and HLV were 430.98 and 424.88 mg g−1, respectively, which is much higher than that of other reported adsorbents. Moreover, HKUST-1 and HLV exhibited favorable adsorption performance for real U(VI)-bearing wastewater samples in practical application. Compared with HKUST-1, low-cost HLV is a very promising potential adsorbent for the removal of uranium from aqueous solutions.
Conflicts of interest
There are no conflicts to declare.
Acknowledgements
This work is financially supported by National Natural Science Foundation of China (21407132) and Environmental Protection Foundation of China Academy of Engineering Physics (YAHZY-2018-008).
References
- A. M. Alansi, W. Z. Alkayali, M. H. Al-qunaibit, T. F. Qahtan and T. A. Saleh, RSC Adv., 2015, 5(87), 71441–71448 RSC.
- R. Soury, M. Jabli, T. A. Saleh, W. S. Abdul-Hassan, E. Saint-Aman, F. Loiseau, C. Philouze and H. Nasri, RSC Adv., 2018, 8(36), 20143–20156 RSC.
- M. M. Al-Shalalfeh, T. A. Saleh and A. A. Al-Saadi, RSC Adv., 2016, 6(79), 75282–75292 RSC.
- H. A. Sani, M. B. Ahmad and T. A. Saleh, RSC Adv., 2016, 6(110), 108819–108827 RSC.
- Z. A. Jamiu, T. A. Saleh and S. A. Ali, RSC Adv., 2015, 5(53), 42222–42232 RSC.
- J. M. Yu, L. H. Xie, J. R. Li, Y. G. Ma, J. M. Seminario and P. B. Balbuena, Chem. Rev., 2017, 117, 9674–9754 CrossRef CAS PubMed.
- K. Xie, Q. Fu, C. L. Xu, H. Lu, Q. H. Zhao, R. Curtain, D. Y. Gu, P. A. Webley and G. G. Qiao, Energy Environ. Sci., 2018, 11, 544–550 RSC.
- R. V. Jagadeesh, K. Murugesan, A. S. Alshammari, H. Neumann, M. M. Pohl, J. Radnik and M. Beller, Science, 2017, 358, 326–332 CrossRef CAS PubMed.
- M. M. Wan, X. L. Zhang, M. Y. Li, B. Chen, J. Yin, H. C. Jin, L. Lin, C. Chen and N. Zhang, Small, 2017, 13, 1701395 CrossRef PubMed.
- D. Sheberla, J. C. Bachman, J. S. Elias, C. J. Sun, Y. Shao-Horn and M. Dincă, Nat. Mater., 2017, 16, 220–225 CrossRef CAS PubMed.
- Y. H. Pi, X. Y. Li, Q. B. Xia, J. L. Wu, Y. W. Li, J. Xiao and Z. Li, Chem. Eng. J., 2018, 337, 351–371 CrossRef CAS.
- N. A. A. Qasem, R. Ben-Mansour and M. A. Habib, Appl. Energy, 2018, 210, 317–326 CrossRef CAS.
- D. Bahamon, A. Díaz-Márquez, P. Gamallo and L. F. Vega, Chem. Eng. J., 2018, 342, 458–473 CrossRef CAS.
- V. K. Gupta, I. Ali, T. A. Saleh, A. Nayak and S. Agarwal, RSC Adv., 2012, 2(16), 6380–6388 RSC.
- L. H. Wee, M. R. Lohe, N. Janssens, S. Kaskel and J. A. Martens, J. Mater. Chem., 2012, 22, 13742–13746 RSC.
- C. Volkringer, C. Falaise, P. Devaux, R. Giovine, V. Stevenson, F. Pourpoint, O. Lafon, M. Osmond, C. Jeanjacques, B. Marcillaud, J. C. Sabroux and T. Loiseau, Chem. Commun., 2016, 52, 12502–12505 RSC.
- H. Y. Zhang, Z. R. Zhang, C. Yang, L. X. Ling, B. J. Wang and H. L. Fan, J. Inorg. Organomet. Polym. Mater., 2018, 28, 694–701 CrossRef CAS.
- W. X. Yang, J. Wang, Q. F. Yang, H. N. Pei, N. Hu, Y. R. Suo, Z. H. Li, D. H. Zhang and J. L. Wang, Chem. Eng. J., 2018, 339, 230–239 CrossRef CAS.
- H. M. Abd El Salam and T. Zaki, Inorg. Chim. Acta, 2018, 471, 203–210 CrossRef CAS.
- N. Chen, N. D. Chen, F. H. Wei, S. Q. Zhao and Y. Luo, Chem. Phys. Lett., 2018, 705, 23–30 CrossRef CAS.
- S. Yekta and M. J. Sadeghi, J. Inorg. Organomet. Polym. Mater., 2018, 28, 1049–1064 CrossRef CAS.
- M. R. Faradonbeh, A. A. Dadkhah, A. Rashidi, S. Tasharofi and F. Mansourkhani, J. Inorg. Organomet. Polym. Mater., 2018, 28, 829–836 CrossRef.
- C. Zhang, Y. Xiao, Y. Qin, Q. C. Sun and S. H. Zhang, J. Solid State Chem., 2018, 261, 22–30 CrossRef CAS.
- S. B. Wu, Y. J. Ge, Y. Q. Wang, X. Chen, F. F. Li, H. Xuan and X. Li, Environ. Technol., 2018, 39, 1937–1948 CrossRef CAS PubMed.
- G. P. Li, S. L. Pang, Y. W. Wu and J. Ouyang, Chem. Eng. Commun., 2018, 205, 698–705 CrossRef CAS.
- G. Wu, J. P. Ma, S. Li, J. Guan, B. Jiang, L. Y. Wang, J. H. Li, X. Y. Wang and L. X. Chen, J. Colloid Interface Sci., 2018, 528, 360–371 CrossRef CAS PubMed.
- J. P. Ma, G. Wu, S. Li, W. Q. Tan, X. Y. Wang, J. H. Li and L. X. Chen, J. Chromatogr. A, 2018, 1553, 57–66 CrossRef CAS PubMed.
- J. P. Ma, Z. D. Yao, L. W. Hou, W. H. Lu, Q. P. Yang, J. H. Li and L. X. Chen, Talanta, 2016, 161, 686–692 CrossRef CAS PubMed.
- Y. F. Feng, H. Jiang, S. N. Li, J. Wang, X. Y. Jing, Y. R. Wang and M. Chen, Colloids Surf., A, 2013, 431, 87–92 CrossRef CAS.
- H. Zhang, J. H. Xue, N. Hu, J. Sun, D. X. Ding, Y. D. Wang and L. Li, J. Radioanal. Nucl. Chem., 2017, 308, 865–875 CrossRef.
- M. R. Azhar, H. R. Abid, H. Q. Sun, V. Periasamy, M. O. Tadé and S. B. Wang, J. Colloid Interface Sci., 2016, 478, 344–352 CrossRef CAS PubMed.
- K.-Y. A. Lin and Y.-T. Hsieh, J. Taiwan Inst. Chem. Eng., 2015, 50, 223–228 CrossRef.
- Z. Q. Li, L. G. Qiu, T. Xu, Y. Wu, W. Wang, Z. Y. Wu and X. Jiang, Mater. Lett., 2009, 63, 78–80 CrossRef CAS.
- T. A. Saleh, Nanomaterial and polymer membranes, Elsevier, 2016, ISBN-13: 978-0128047033 Search PubMed.
- T. A. Saleh, Advanced nanomaterials for water engineering, treatment, and hydraulics, IGI Glob., 2017, ISBN-13: 978-1522521365 Search PubMed.
- A. W. Thornton, R. Babarao, A. Jain, F. Trousselet and F.-X. Coudert, Dalton Trans., 2015, 45, 4352–4359 RSC.
- F. Zare, M. Ghaedi, A. Daneshfar, S. Agarwal, I. Tyagi, T. A. Saleh and V. K. Gupta, Chem. Eng. J., 2015, 273, 296–306 CrossRef CAS.
- T. A. Saleh, K. Naeemullah, M. Tuzen and A. Sarı, Chem. Eng. Res. Des., 2017, 117, 218–227 CrossRef CAS.
- E. A. Elshehy, Sep. Sci. Technol., 2017, 52, 1852–1861 CrossRef CAS.
- X. Guo, Y. R. Feng, L. Ma, D. Z. Gao, J. Jing, J. C. Yu, H. B. Sun, H. Y. Gong and Y. J. Zhang, Appl. Surf. Sci., 2017, 402, 53–60 CrossRef CAS.
- W. Y. Wu, D. Y. Chen, J. W. Li, M. H. Su and N. Chen, Environ. Sci. Pollut. Res., 2018, 25, 18096–18108 CrossRef CAS PubMed.
- R. Khamirchi, A. Hosseini-Bandegharaei, A. Alahabadi, S. Sivamani, A. Rahmani-Sani, T. Shahryari, I. Anastopoulos, M. Miri and H. N. Tran, Ecotoxicol. Environ. Saf., 2018, 150, 136–143 CrossRef CAS PubMed.
- Z. R. Dai, H. Zhang, Y. Sui, D. X. Ding, N. Hu, L. Li and Y. D. Wang, J. Radioanal. Nucl. Chem., 2018, 316, 369–382 CrossRef CAS.
- S. Abdi, M. Nasiri, A. Mesbahi and M. H. Khani, J. Hazard. Mater., 2017, 332, 132–139 CrossRef CAS PubMed.
- X. Y. Deng, Y. L. Feng, H. R. Li, F. Yuan, Q. Teng and H. J. Wang, J. Radioanal. Nucl. Chem., 2018, 315, 243–250 CrossRef CAS.
- J. L. Wu, K. Tian and J. L. Wang, Prog. Nucl. Energy, 2018, 106, 79–86 CrossRef CAS.
- J. Tan, Y. F. Wang, N. Liu and M. W. Liu, J. Radioanal. Nucl. Chem., 2018, 315, 119–126 CrossRef CAS.
|
This journal is © The Royal Society of Chemistry 2019 |
Click here to see how this site uses Cookies. View our privacy policy here.