DOI:
10.1039/C9RA01731C
(Paper)
RSC Adv., 2019,
9, 13543-13549
Incorporating polyoxometalates and organic ligands to pursue 3d–4f heterometallic clusters: a series of {Cr4Ln4} clusters stabilized by phthalic acid and [SiW12O40]4−†
Received
7th March 2019
, Accepted 23rd April 2019
First published on 1st May 2019
Abstract
By introduction of trilacunary Keggin-type polyoxometalate to the hydrothermal reaction system of Cr3+, Ln3+ and phthalic acid, a series of novel {Cr4Ln4} heterometallic clusters with the formula Cs2[Cr4Ln4(μ4-O)4(μ3-O)4(C8H4O4)4(H2O)12](H3SiW12O40)Cl·23H2O (1-Ln, Ln = Ce, Pr, Nd) and [Cr4Ln4(μ4-O)4(μ3-O)4(C8H4O4)4(H2O)10](H6SiW12O40)Cl2·18H2O (2-Ln, Ln = Sm, Eu, Gd, Tb, Dy, Ho, Er) have been obtained. Single-crystal structural analyses show that 1-Ln and 2-Ln constitute the first cases of Cr–Ln heterometallic clusters stabilized by inorganic polyoxometalate anions and organic ligands. Optical spectra studies demonstrate that 1-Ln and 2-Ln are narrow-gap semiconductors with band gaps of about 1.5 eV. Magnetic investigation shows that compound 2-Dy is a potential single molecule magnet.
Introduction
The syntheses and investigation of unique 3d–4f heterometallic clusters have long been a field of great interest in modern inorganic chemistry.1 The inherent contribution of the 3d and 4f electrons as well as the distinct magnetic couplings between two different metal ions within the heterometallic cluster often result in remarkable physicochemical, electronic, catalytic and magnetic properties, which makes 3d–4f heterometallic clusters promising functional materials to be used as single molecule magnets, molecular magnetic coolers, bifunctional catalysts and so on.2 However, the preparation of 3d–4f heterometallic clusters is still not a simple task because of the various synthetic obstacles, such as different coordination number/geometries/affinity of 3d and 4f metal ions, charge matching and balance, and low yield caused by competing reactions between 3d and 4f metal ions with the same organic donor.3 So far, most of reported works in this area focus on 3d–4f systems applying the later 3d transition metal including Fe3+, Co2+, Ni2+, and Cu2+ ions mixed with lanthanide ions, resulting in several representative examples with intriguing structures and interesting properties.4 In contrast, less efforts currently have been placed to employ the early transition metal ion Cr3+ to combine with lanthanide ions for constructing 3d–4f heterometallic clusters, probably due to the inert nature and rigid coordination characteristic of Cr3+ ion. The Cr3+ ion shows weaker anisotropy than those later transition metal ions such as Ni2+, Co2+.5 Recent studies have also shown that the introduction of Cr3+ ion to combine with lanthanide ions with large anisotropy may generates new possibilities to obtain heterometallic Cr–Ln clusters with SMM behaviors.6 This is because the weak Cr–Ln magnetic exchange interactions might strengthen the quantum tunneling of the magnetization, leading to the observation of the slow relaxation of the magnetization with a high energy barrier.7 In this regard, the search of feasible approaches to design and construct new Cr–Ln heterometallic clusters is a highly desirable but challenging task.
Up to now, a few of Cr–Ln heterometallic clusters have been made by using Cr3+, Ln3+ ions to react with specific organic chelating ligands. Typical examples include {CrLn2},8 {Cr2Ln2},6b,7b {Cr2Ln3},9 {Cr4Ln},10 {Cr2Ln4},11 {Cr3Ln3},6b {Cr4Ln4},6b,12 {Cr3Ln6},13 {Cr6Ln6}5 and {Cr8Ln8}.6a Notably, the above Cr–Ln heterometallic clusters are all stabilized by only one type of organic ligand or simple inorganic oxo-anions such as sulfate radicals. It is believed that the introduction of a second ligand to the reaction system would bring new possibilities to achieve new Cr–Ln heterometallic clusters. In our exploration strategy, we are especially interested to introduce inorganic polyoxometalates (POMs) as a second ligand to cooperate with organic ligands for making new Cr–Ln heterometallic clusters, considering that anionic POM anions can serve as polydentate ligands, and transform into suitable configurations in situ to meet the coordination requirement in the final product.14 Fortunately, in this contribution, Keggin-type polyanions have been successfully involved in the reaction system, and a series of novel Cr–Ln heterometallic clusters with general formula of Cs2[Cr4Ln4(μ4-O)4(μ3-O)4(C8H4O4)4(H2O)12](H3SiW12O40)Cl·23H2O (1-Ln, Ln = Ce, Pr, Nd) and [Cr4Ln4(μ4-O)4(μ3-O)4(C8H4O4)4(H2O)10](H6SiW12O40)Cl2·18H2O (2-Ln, Ln = Sm, Eu, Gd, Tb, Dy, Ho, Er) have been hydrothermally synthesized and fully characterized (Scheme 1). These compounds contain similar four-layered prismatic [Cr4Ln4] cores, which to our knowledge constitute the first cases of Cr–Ln heterometallic clusters stabilized by both organic ligands and inorganic POM species.
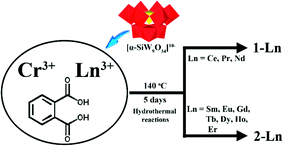 |
| Scheme 1 The synthetic method for 1-Ln and 2-Ln. | |
Experimental section
Materials and measurements
Na10[α-SiW9O34]·16H2O was synthesized on the basis of literature method and proved by IR spectroscopy.15 Other raw materials were analytical grade reagents, all of which were obtained from commercial sources and used without further purification. Infrared (IR) spectra (KBr particles) were tested on an Opus Vertex 70 FT-IR infrared spectrophotometer with a wavelength in the range of 500–4000 cm−1. Elemental analyses of C, H were measured on a Vario EL Cube Germany Elementar. Powder X-ray diffraction (PXRD) patterns were tested on a Rigaku DMAX 2500 diffractometer with CuKα radiation (λ = 1.54056 Å). Thermogravimetric analyses (TGA) were carried out on a DSC/DTA-TG Netzsch STA 449F5 analyzer under a nitrogen flow atmosphere with a heating rate of 10 °C min−1 at a temperature of 25–800 °C. The UV-Vis diffuse reflectance spectrum was carried out at UV-2600 Shimadzu Japan UV-Vis spectrophotometer ranging from 200 to 1200 nm with BaSO4 as a reference. The PPMS-9T magnetometer with quantum design was used to measure the magnetization rate of polycrystalline samples at variable temperature within the range of 2–300 K under 0.1 tesla magnetic field. The experimental permittivity was modified to Pascal's constant. Ac impedance measurements were tested on zennium/IM6 impedance analyzer which the applied voltage was 50 mV and the frequency was in the scope of 0.1 Hz to 5 MHz. Constant temperature and humidity conditions were carried out by a STIK Corp. CIHI-150B incubator. The samples were pressed to form carbon-sample-carbon three layers of cylindrical sample (∼3 mm thickness × 5 mm ϕ) coated with C-pressed electrodes. Two silver electrodes were attached to both sides of cylindrical sample to form four end terminals (quasi-four-probe method).
Synthesis of Cs2[Cr4Ln4(μ4-O)4(μ3-O)4(C8H4O4)4(H2O)12](H3SiW12O40)Cl·23H2O (1-Ln, Ln = Ce, Pr, Nd). A mixture of Na10[α-SiW9O34]·16H2O (0.255 g, 0.093 mmol), CrCl3·6H2O (0.102 g, 0.383 mmol), Ln(NO3)3·6H2O (0.461 mmol), phthalic acid (0.050 g, 0.301 mmol), Na2CO3 (0.051 g, 0.481 mmol), CsCl (0.053 g, 0.315 mmol), H2O (5 mL) was adequately stirred for 1 h (pHs = 3.8), and then was sealed in a 35 mL stainless steel reactor with a Teflon liner and kept at 140 °C. After 5 days, the reactor was cooled to room temperature naturally (pHe = 3.2). Black block crystals were collected by filtration, washed with distilled water, and dried in air (Fig. S1†). Yield: ca. 43.0 mg (8.63% based on Na10[α-SiW9O34]·16H2O). Elemental analysis calculated (%) for C32H89O99SiClCr4Cs2Ce4W12 (Fw = 5361.86): C 7.16, H 1.67. Found (%): C 7.73, H 1.75. IR (KBr, cm−1): 3346(s), 1570(vs), 1477(w), 1409(vs), 1386(w), 1172(w), 1007(w), 958(m), 910(vs), 783(s), 757(s), 690(m), 655(m), 577(w), 535(w).
Synthesis of [Cr4Ln4(μ4-O)4(μ3-O)4(C8H4O4)4(H2O)10](H6SiW12O40)Cl2·18H2O (2-Ln, Ln = Sm, Eu, Gd, Tb, Dy, Ho, Er). 2-Ln was prepared by a similar procedure in 1-Ln. Keeping all reaction parameter the same as 1-Ln, equal amount of heavy rare earth salts were used in the reactions (pHs = 3.7, pHe = 2.8). Black block crystals were collected by filtration, washed with distilled water, and dried in air (Fig. S1†). Yield: ca. 171 mg (36.21% based on Na10[α-SiW9O34]·16H2O). Elemental analysis calculated (%) for C32H78O92SiCl2Cr4Tb4W12 (Fw = 5083.66): C 7.56, H 1.54. Found (%): C 7.80, H 1.60. IR (KBr, cm−1): 3324(m), 1560(vs), 1478(m), 1413(vs), 1386(w), 1173(w), 1007(w), 956(m), 910(vs), 788(s), 757(s), 689(m), 657(m), 586(s), 549(m).
Single-crystal structure analysis
Single crystals of 1-Ce and 2-Tb were selected as examples for crystal structure data collection. The crystallographic data of 1-Ce and 2-Tb were collected on a Bruker APEX2 duo CCD diffractometer under a nitrogen atmosphere (150 K) using graphite-monochromated Mo Kα radiation (λ = 0.71073). The direct method was used to solve the crystal structures, and the full-matrix least-squares refinement was performed based on F2 according to the SHELX-2014 package. All non-hydrogen atoms were refined by using anisotropic thermal parameters. In 1-Ce and 2-Tb, the SiO4 tetrahedra in Keggin-type [SiW12O40]4− polyanion were disordered into cubes, which is common in POM chemistry.16 The determination of Cl− anions in 1-Ce and 2-Tb was according to the results of SCXRD and EDS elemental mapping (Fig. S2–S3†). The identities of the other isostructural compounds were proved by single crystal indexing, PXRD characterizations and EDS spectra (Fig. S4†). The final formula of 1-Ce and 2-Tb were defined by combining the SCXRD results with C, H elemental analyses, TGA (Fig. S5†). An overview of the crystallographic data and structural refinements of 1-Ce and 2-Tb are summarized in Table 1. CCDC 1892101 (1-Ce) and 1892102 (2-Tb) comprise the supplementary crystallographic data for this paper.
Table 1 The X-ray crystallographic data for 1-Ce and 2-Tba
|
1-Ce |
2-Tb |
R1 = ∑‖Fo| − |Fc‖/∑|Fo|. wR2 = [∑w(Fo2 − Fc2)2/∑w(Fo2)2]1/2; w = 1/[σ2(Fo2) + (xP)2 + yP], P = (Fo2 + 2Fc2)/3, where x = 0.0897, y = 336.90 for 1-Ce; where x = 0.0635, y = 302.83 for 2-Tb. |
Empirical formula |
Cs2Cr4Ce4O99C32 H89Si1W12Cl1 |
Cr4Tb4O92C32 H78Si1W12Cl2 |
Formula weight |
5361.87 |
5083.66 |
Crystal system |
Triclinic |
Triclinic |
Space group |
P![[1 with combining macron]](https://www.rsc.org/images/entities/char_0031_0304.gif) |
P![[1 with combining macron]](https://www.rsc.org/images/entities/char_0031_0304.gif) |
a (Å) |
13.5174(11) |
12.4544(9) |
b (Å) |
13.5216(11) |
13.4312(9) |
c (Å) |
29.869(2) |
31.842(2) |
α (°) |
81.6102(15) |
80.2079(13) |
β (°) |
82.6279(16) |
80.4562(12) |
γ (°) |
89.5941(17) |
73.7358(12) |
V (Å3) |
5356.0(8) |
4999.4(6) |
Z |
2 |
2 |
F(000) |
4466 |
4328 |
ρcalcd |
3.125 |
3.240 |
Temperature (K) |
150(2) |
150(2) |
μ (mm−1) |
15.682 |
17.117 |
Refl. Collected |
41 293 |
37 382 |
Independent relf. |
18 701 |
17 215 |
Parameters |
1267 |
1240 |
GOF on F2 |
1.091 |
1.066 |
Final R indices |
R1 = 0.0658 |
R1 = 0.0574 |
(I = 2σ(I)) |
wR2 = 0.1818 |
wR2 = 0.1463 |
R indices (all data) |
R1 = 0.0751 |
R1 = 0.0676 |
wR2 = 0.1897 |
wR2 = 0.1527 |
Results and discussion
Crystal structure description of 1-Ln and 2-Ln
In this contribution, compounds 1-Ce and 2-Tb were used as examples for the structural description. SCXRD analyses reveal that both 1-Ce and 2-Tb crystallize in monoclinic P
space group, and contain similar octanuclear heterometallic clusters, [Cr4Ce4(μ4-O)4(μ3-O)4(μ2-O)2(C8H4O4)4(H2O)12] (Cr4Ce4) and [Cr4Tb4(μ4-O)4(μ3-O)4(μ2-O)2(C8H4O4)4(H2O)10] (Cr4Tb4) (Fig. 1a and b), and identical Keggin-type [SiW12O40]4− polyanions (Fig. 1c). Cr4Ce4 is consist of four Cr3+, four Ce3+, four μ4-O atoms, four μ3-O atoms, two μ2-O and twelve water ligands. All Cr3+ ions adopt a regular octahedral geometry with two μ4-O atoms, two μ3-O atoms and two O atoms from two carboxylatic groups of two phthalic ligands. Each Ce3+ employs a nine-coordinated conformation. For Ce1 and Ce2, each of them is surrounded by two μ3-O atoms, one μ4-O atom, two carboxylate O atoms, one μ2-H2O (O3w/O17w) ligand and three terminal water molecules. While for Ce3 and Ce4, their coordination environments are similar with Ce1 and Ce2 except for the replacement of one terminal water ligand with one μ2-O atom (O29/O28) from [SiW12O40]4− polyanion. The composition and structure of Cr4Tb4 is similar with Cr4Ce4 except for the removal of two μ2-H2O ligands. Additionally, all Tb3+ ions are eight-coordinated in Cr4Tb4. The oxidation states of Cr3+, Ce3+ and Tb3+ are confirmed through bond valence sum calculations (Tables S1–S2†).17 The deprotonated phthalic ligand exhibit a μ4-η1:η1:η1:η1 coordination mode (Fig. 1d).18 The Keggin-type [SiW12O40]4− polyanions were generated by in situ structural transformation from trilacuanry [SiW9O34]10− starting material, which is common in hydrothermal reactions.19 In Cr4Ce4/Cr4Tb4, four Cr3+ ions are integrated by four μ4-O atoms to form a cubane-like Cr4O4 cluster. Then, two dinuclear [Ce2(μ3-O)2(H2O)]/[Tb2(μ3-O)2] clusters cap on the top and bottom of the Cr4O4 cluster in a mutually perpendicular style, resulting in a four-layered heterometallic core [Cr4Ce4(μ4-O)4(μ3-O)4(H2O)2]/[Cr4Tb4(μ4-O)4(μ3-O)4] (Fig. 1e and f). Such cores are much different from planar square-like {Cr4Ln4} cores in Powell's work (Fig. S6†).6b,12a The four-layered cores are further stabilized by four deprotonated phthalic ligands, ten terminal water molecules and two μ2-O atoms from two [SiW12O40]4− polyanions, giving rise to the neutral Cr4Ce4/Cr4Tb4 clusters. The inter-connection between Cr4Ce4/Cr4Tb4 clusters and [SiW12O40]4− polyanions further produces two kinds of 1-D zigzag cluster chains (Fig. 2). The difference between these chains is that half of [SiW12O40]4− polyanions exhibit different linking styles. Finally, the stacking of these cluster chains leads to the formation of 3-D structures which show irregular channels (Fig. S7–S8†).
 |
| Fig. 1 (a) Structure of the [Cr4Ce4(μ4-O)4(μ3-O)4(μ2-O)2(C8H4O4)4(H2O)12] cluster; (b) structure of the [Cr4Tb4(μ4-O)4(μ3-O)4(μ2-O)2(C8H4O4)4(H2O)10] cluster; (c) structure of the Keggin-type [SiW12O40]4− polyanion; (d) view of the coordination mode of deprotonated phthalic ligand. In (a and b) and (e and f), the thermal ellipsoids were drawn at 50% level. Color codes: Ce, cyan; Tb, olive; Cr, green; W, blue; O red; C, gray; Si yellow. WO6, red; SiO4, yellow. | |
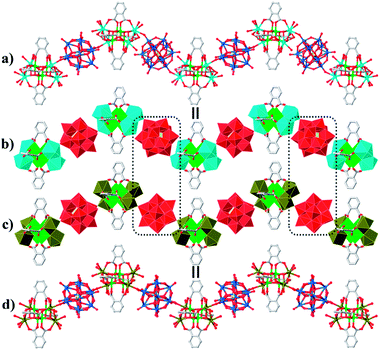 |
| Fig. 2 (a and b) Polyhedral and stick representation of the 1-D heterometallic cluster chains in 1-Ce. (c and d) Polyhedral and stick representation of the 1-D heterometallic cluster chains in 2-Tb. Color codes: Ce, cyan; Tb, olive; Cr, green; W, blue; O red; C, gray; Si yellow; WO6, red; SiO4, yellow; CrO6, green; CeO9, cyan; TbO8, olive. | |
It is noteworthy that Xu Yan and coworkers reported two heterometallic clusters containing similar four-layered [Cr4Ln4(μ4-O)4(μ3-O)4] (Ln = Gd, Dy) cores very recently.12b However, 1-Ce and 2-Tb are much different from those in Xu Yan's work, mainly in the following areas: (1) the heterometallic cores in 1-Ce and 2-Tb are stabilized by both phthalic ligands and Keggin-type [SiW12O40]4− polyanions, while those in Xu Yan's work are only coordinated with isonicotinic ligands; (2) 1-Ce and 2-Tb are 1-D extended structures, whereas the compounds in Xu Yan's work are discrete clusters; (3) the rare earth ions in 1-Ce and 2-Tb can be replaced by other lanthanide ions (Pr3+, Nd3+, Sm3+, Eu3+, Gd3+, Dy3+, Ho3+, Er3+) to form another eight isologues, nonetheless, only Gd/Dy-based compounds were obtained in Xu Yan's work. In addition, a new methodology for clearly describing, classifying and searching high-nuclear clusters has been established and developed recently,20–23 which consider each metal center as a node and every monatomic bridge as a linker. The resulting graph of each polynuclear compound can be described by a unique NDk-m symbol,24 where N is the set of coordination numbers of topologically nonequivalent nodes, D is dimensionality; D = M for finite (molecular) clusters, k is the number of nodes in the cluster, and m enumerates topologically different clusters with the same NDk symbol. An isolated metal atom is designated (0). Thus, every NDk-m symbol denotes a topological type, i.e., a set of topologically equivalent but likely conformationally different CC skeletons. Such method is also applicable to POM clusters, and has been successfully applied in compound like 4,4,5,6M52-1 for [{Co4(OH)3PO4}4(A-α-XW9O34)4]32− (X = SiIV, GeIV) and 3,6M16-1 for the central {Co16} unit.25,26 In this context, compounds 1-Ce and 2-Tb can be described as a motif enumerated as 3,4,4,5,5M20-1 (Fig. S9†), and the 3d–4f heterometallic clusters unit can be described as 3,5M8-1 (Fig. S10†).
Syntheses
Because of the inert characteristic, Cr3+ ion is not easy to combine with lanthanide ions to form high-nuclear heterometallic clusters. During the course of preparing 1-Ln and 2-Ln, we found that the following parameters have important influences on the crystal growth. First, Na2CO3 and CsCl are indispensible for making 1-Ln and 2-Ln. If anyone was removed from the reaction system, 1-Ln and 2-Ln cannot be obtained. Next, the optimal reaction temperature is about 140 °C. If the reactions were run at temperatures higher than 160 °C or lower than 120 °C, the yields decrease sharply. Additionally, we introduced other carboxylate ligands such as benzoic acid, isonicotinic acid, 4,5-imidazoledicarboxylic acid, to replace phthalic acid for further exploration. But, we were fruitless. What's more, we used different POMs precursors such as [GeW11O39]8−, [PW9O34]9−, [GeW9O34]10− to substitute [α-SiW9O34]10− for further exploration. Unfortunately, we were failed to obtain suitable crystals. Finally, the radius of lanthanide ions show impact on the crystallization of the final structures. As well known, the radius of lanthanide ions are very close due to the presence lanthanide contraction. However, in our work, the lighter rare earth ions (Ce3+, Pr3+, Nd3+) tend to form 1-Ln series, whereas the heavier rare earth ions (Sm3+, Eu3+, Gd3+, Tb3+, Dy3+, Ho3+, Er3+) prefer to crystallize in 2-Ln series. These results indicate that the minor radius difference between rare earth ions still have obvious impact on the formation of the final products.
PXRD patterns, IR spectra, UV-Vis diffuse reflectance spectra
The good agreement between the experimental PXRD patterns and the calculated ones by Mercury software based on SCXRD results testifies that the as-prepared samples of 1-Ln and 2-Ln are pure phases (Fig. S11–S12†). In the IR spectra (Fig. S13–S14†), the peaks around 3359 cm−1 ascribe to the stretching vibration of –OH of the coordination water ligands. The vibration peaks located at 1400–1580 cm−1 correspond to the presence of the phenyl ring, carboxylate groups. The peaks at 957, 908, 879, 783 cm−1 attribute to the vibration of W–Ot, Si–Oc, W–Ob and W–Oc (Ot: terminal O atom; Ob: μ2-O atom; Oc: μ4-O atom), which attribute to characteristic vibrations of Keggin-type [SiW12O40]4− skeleton.27 In addition, the adsorption peaks in the range of 780–600 cm−1 derive from the stretching vibrations of the Cr–O28 and Ln–O12b bonds in the heterometallic cluster. The ultraviolet-visible diffuse reflectance spectra with BaSO4 as reference were also measured to evaluate the light-harvesting properties of 1-Ce and 2-Tb (Fig. S15†). It can be seen from the spectra that the two crystals have obvious absorption in the visible-ultraviolet region. The band gap energies were 1.49 eV for 1-Ce and 1.50 eV for 2-Tb according the fitting curve of Kubelka–Munk Function29 vs. energy (eV), indicating the potential applications of compounds 1-Ce and 2-Tb as semiconductor materials.
Magnetic properties
Considering the presence of large unquenched orbital angular momentum in Tb3+ and Dy3+, 2-Tb and 2-Dy were selected for magnetic studies. The temperature-dependent magnetic susceptibility of 2-Tb and 2-Dy were measured at the temperature range of 2 to 300 K with the applied magnetic field of 1 kOe. As shown in Fig. 3a and b, the experimental χmT values of 2-Tb/2-Dy are 54.95/66.61 cm3 K mol−1 at room temperature, which are conforming to the theoretical values of 50.80/61.59 cm3 K mol−1 based on four non-interacting Cr3+ (S = 3/2 and g = 2) ions and four isolated Tb3+ (7F6, S = 3, L = 3, g = 3/2, C = 11.82 cm3 K mol−1)/Dy3+ (6H15/2, S = 5/2, L = 5, g = 4/3, C = 14.17 cm3 K mol−1) ions. In 2-Tb, the experimental χmT value decreases slowly from 54.95 to 51.54 cm3 K mol−1 along with the temperature decreasing from 300 to 36 K, and then falls sharply to 21.47 cm3 K mol−1 at 2 K. The continuous downward behaviour demonstrates the existence of overall antiferromagnetic interactions with 2-Tb. Such result is consistent with that in Xu Yan's work.12b In 2-Dy, upon cooling, the χmT value increases to a maximum of 91.05 cm3 K mol−1 at 28 K. Upon cooling below 28 K, the χmT value decreases sharply to reach to the minimum value of 32.16 cm3 K mol−1 at 2 K. Such a behaviour suggests the coexistence of both ferromagnetic and antiferromagnetic interactions between metal centers in 2-Dy. The fittings of 1/χm vs T curves found that 2-Tb/2-Dy abide the Curie–Weiss rule in the range of 2–300/50–300 K, respectively, giving Curie constants and Weiss constants of 54.56 cm3 mol−1 and −3.07 K for 2-Tb, and 62.58 cm3 mol−1 and 14.97 K for 2-Dy. The negative Weiss constant further support the occurrence of antiferromagnetic interactions within 2-Tb, while the positive Weiss constant proves the presence of main ferromagnetic interactions in 2-Dy.
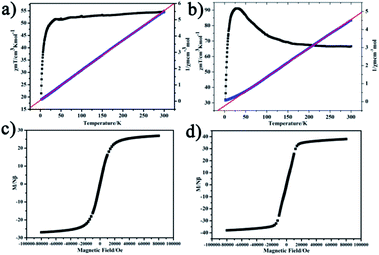 |
| Fig. 3 (a and b) The temperature-dependent χmT (black) and 1/χm (blue) curves of 2-Tb (a) and 2-Dy (b) in the applied magnetic field of 1 kOe with the temperature range of 2–300 K. The red lines are the fitting of 1/χm versus T curves according to the Curie–Weiss rule. (c and d) Field variation of magnetic susceptibility in 2 K for 2-Tb (c) and 2-Dy (d). | |
A more detailed analyses of the field-dependent isothermal magnetization behaviors of 2-Tb/2-Dy were carried out under 0–80 kOe at 2 K (Fig. 3c and d). In 2-Tb, the M value rises rapidly to 24.21 Nβ at 28 kOe, then it grows steady to the maximum value of 26.87 Nβ at 80 kOe, which much lower than the theoretical value of 36 Nβ according to four non-interacting Tb3+ and four Cr3+ ions. Such deviation illustrates the presence of a significant magnetic anisotropy and/or low lying excited states.5 In 2-Dy, the M value shows a similar trend in 2-Tb. the maximum value of 37.97 Nβ occur at 80 kOe, is in good agreement of theoretical value of 32 Nβ according to four non-interaction Dy3+ and four Cr3+. Such a saturation in magnetization is also found in previous works.11b
In order to investigate whether the magnetic interaction between metal ions can cause the properties of single molecule magnets, the variable-temperature alternating current susceptibility of 2-Tb and 2-Dy at frequencies from 111 to 2311 Hz in the scope of 2–20 K were tested (Fig. 4). The in-phase (χ′) and out-of-phase (χ′′) plots of 2-Tb do not exhibit obvious frequency-dependent behaviours. Nevertheless, the in-phase (χ′) and out-of-phase (χ′′) values of 2-Dy shows weak frequency dependent, which indicates the potential single molecule magnetic behaviour. Such a slow relaxation might arise from the anisotropic Dy3+ ions associated with its relatively large ground spin states.11b There is no maximum in χ′′, which may be caused by fast quantum tunnelling effect.30
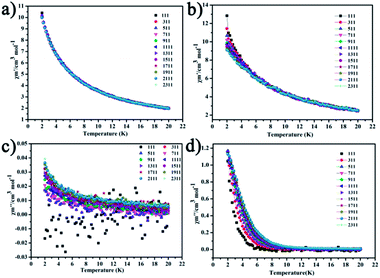 |
| Fig. 4 Frequency-dependent behavior of and for 2-Tb (a and c) and 2-Dy (b and d) in zero static field at 2–20 K. | |
Proton conduction properties
Proton-conductive materials are an important class of promising materials in the fuel cells and battery preparation.31 Considering that there are a lot of lattice water molecules, and the good water stability of 1-Ln and 2-Ln, we selected 1-Ce and 2-Tb as examples to evaluate their proton conductive ability. The proton conductivities were measured by using compacted granular crystalline powder samples, AC impedance measurements and evaluated from the half-circles in the Nyquist plots. By using the equivalent circuit simulation of the ZSimpWin software, the resistance value is extrapolated from the impedance data. The following equation was used to calculate the conductivity: σ = L/RS, where σ is the conductivity, L is the thickness (∼0.160 cm for 1-Ce; ∼0.178 cm for 2-Tb) and S is the cross section of the particle (0.5 cm ϕ). The volume resistance R is extracted directly from the impedance graph. Firstly, the conductivities were measured on relative humidity (RH) with 55%, 70%, 85%, 98% RH respectively at a constant temperature of 30 °C for 1-Ce and 2-Tb. As shown in Fig. 5a 6a, the conductivities of 1-Ce and 2-Tb at 30 °C with 55% RH are 1.04 × 10−6 S cm−1 and 1.17 × 10−6 S cm−1, respectively. When the RH increases from 55% to 98%, the conductivities increase to 1.16 × 10−5 S cm−1 and 1.80 × 10−5 S cm−1 for 1-Ce and 2-Tb, respectively. The enhanced conductivities should be ascribed to that more water molecules entered into the crystal lattice. Furthermore, the conductivities were tested at temperature of 30 °C, 40 °C, 50 °C, 60 °C, 70 °C, 80 °C with constant RH of 98% for 1-Ce and 2-Tb (Fig. 5b and c, 6b and c). When the temperatures increased from 30 °C to 80 °C, the conductivities of 1-Ce and 2-Tb enhanced from 1.16 × 10−5 S cm−1 to 8.72 × 10−4 S cm−1 and from 1.80 × 10−5 S cm−1 to 2.37 × 10−3 S cm−1, separately. These values are comparable to most proton-conducting metal–organic framework materials32 and some POM-based materials.33 The enhancement of conductivities should be attributed to that the ion transition rates in the crystal lattice were accelerated as the temperatures increased.34 Meanwhile, these values also indicate that temperatures exhibit more important impact on conductivities than RH. Finally, according to the Arrhenius equation σT = σ0
exp(−Ea/kbT), the activation energies Ea at 98% RH were estimated to be 0.81 and 0.94 eV for 1-Ce and 2-Tb (Fig. 5d, 6d), respectively. The mechanism of ion conduction is that vehicular mechanism plays a leading role, on account of the activation energies greater than 0.4 eV, which is expounded that there are ion conduction channels in the structure, and ions conduct electricity in the structure by means of transportation.35 The proton conduction in our materials is mainly carried out through hydrogen-bonding network among water molecules and protons, which is resulted from a combination of POMs, 3d–4f heterometalic clusters and organic species.36,37
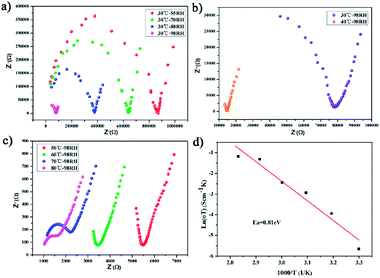 |
| Fig. 5 (a) Nyquist plots for 1-Ce at different RHs and T = 30 °C; (b and c) Nyquist plots for 1-Ce at different temperatures and 98% RH; (d) Arrhenius plots of the conductivity of 1-Ce. | |
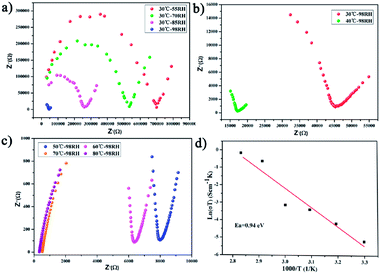 |
| Fig. 6 (a) Nyquist plots for 2-Tb at different RHs and T = 30 °C; (b and c) Nyquist plots for 2-Tb at different temperatures and 98% RH; (d) Arrhenius plots of the conductivity of 2-Tb. | |
Conclusions
A family of octanuclear 3d–4f heterometallic clusters, 1-Ln and 2-Ln, have been hydrothermally made. SCXRD structural analyses reveal that all compounds are constructed from novel Cr4Ln4 cores stabilized by phthalic ligands and Keggin-type POMs. Optical spectra studies demonstrate that these materials are narrow-gap semiconductors with band gaps of about 1.5 eV. The magnetic investigation reveals the presence of antiferromagnetic interactions within the Cr4Tb4 core and the coexistence of ferromagnetic and antiferromagnetic couplings with Cr4Dy4 core. What's more, 2-Dy shows a single molecule magnet behaviour. Finally, proton conductive measurements reveal that 1-Ce and 2-Tb exhibit moderate conductivities at 80 °C with 98% RH. Our results also prove that the introduction of POM clusters to combine with organic chelating ligands as structure directing agents is an effective route to pursue unique 3d–4f heterometallic clusters. Further work focused on pursuing higher-nuclear Cr–Ln heterometallic clusters is underway in our group.
Conflicts of interest
The authors declare no competing financial interest.
Acknowledgements
This work was financially supported by National Natural Science Foundation of China (No. 21671040 and 21773029) and the Natural Science Foundation of Fujian Province (No. 2017J01579).
Notes and references
-
(a) K. Griffiths and G. E. Kostakis, Dalton Trans., 2018, 47, 12011 RSC;
(b) S. Fan, S. H. Xu, X. Y. Zheng, Z. H. Yan, X. J. Kong, L. S. Long and L. S. Zheng, CrystEngComm, 2018, 20, 2120 RSC;
(c) J. W. Zhao, Y. Z. Li, L. J. Chen and G. Y. Yang, Chem. Commun., 2016, 52, 4418 RSC.
-
(a) D. P. Liu, X. P. Lin, H. Zhang, X. Y. Zheng, G. L. Zhuang, X. J. Kong, L. S. Long and L. S. Zheng, Angew. Chem., Int. Ed., 2016, 55, 4532 CrossRef CAS PubMed;
(b) Y. N. Gu, Y. Chen, Y. L. Wu, S. T. Zheng and X. X. Li, Inorg. Chem., 2018, 57, 2472 CrossRef CAS PubMed;
(c) S. K. Singh, M. F. Beg and G. Rajaraman, Chem.–Eur. J., 2016, 22, 672 CrossRef CAS PubMed;
(d) L. Jiang, B. Liu, H. W. Zhao, J. L. Tian, X. Liu and S. P. Yan, CrystEngComm, 2017, 19, 1816 RSC.
-
(a) Y. N. Gu, H. Yu, L. D. Lin, Y. L. Wu, Z. Li, W. Y. Pan, J. He, L. Chen, Q. Li and X. X. Li, New J. Chem., 2019, 43, 3011 RSC;
(b) Q. Lin, Y. Zhang, W. Cheng, Y. Liu and Y. Xu, Dalton Trans., 2017, 46, 643 RSC.
-
(a) S. Chen, V. Mereacre, C. E. Anson and A. K. Powell, Dalton Trans., 2016, 45, 98 RSC;
(b) K. R. Vignesh, S. K. Langley, K. S. Murray and G. Rajaraman, Chem.–Eur. J., 2017, 23, 1654 CrossRef CAS PubMed;
(c) X. Y. Zheng, X. J. Kong, Z. Zheng, L. S. Long and L. S. Zheng, Acc. Chem. Res., 2018, 51, 517 CrossRef CAS PubMed.
- S. Chen, V. Mereacre, Z. Zhao, W. Zhang, M. Zhang and Z. He, Dalton Trans., 2018, 47, 7456 RSC.
-
(a) L. Qin, J. Singleton, W. P. Chen, H. Nojiri, L. Engelhardt, R. E. P. Winpenny and Y. Z. Zheng, Angew. Chem., Int. Ed., 2017, 56, 16571 CrossRef CAS PubMed;
(b) J. Rinck, Y. Lan, C. E. Anson and A. K. Powell, Inorg. Chem., 2015, 54, 3107 CrossRef CAS PubMed.
-
(a) S. Chen, V. Mereacre, C. E. Anson and A. K. Powell, Dalton Trans., 2016, 45, 9336 RSC;
(b) S. K. Langley, D. P. Wielechowski, B. Moubaraki and K. S. Murray, Chem. Commun., 2016, 52, 10976 RSC.
- P. E. Car, A. Favre, A. Caneschi and R. Sessoli, Dalton Trans., 2015, 44, 15769 RSC.
- X. Q. Wang, Z. Y. Li, Z. X. Zhu, J. Zhu, S. Q. Liu, J. Ni and J. J. Zhang, Eur. J. Inorg. Chem., 2013, 5153 CrossRef CAS.
- O. Blacque, A. Amjad, A. Caneschi, L. Sorace and P. E. Car, New J. Chem., 2016, 40, 3571 RSC.
-
(a) H. Xiang, W. G. Lu, W. X. Zhang and L. Jiang, Dalton Trans., 2013, 42, 867 RSC;
(b) H. Xiang, W. G. Lu, L. Jiang, W. X. Zhang and Y. Lan, Eur. J. Inorg. Chem., 2016, 6, 907 CrossRef.
-
(a) J. Rinck, G. Novitchi, W. Van den Heuvel, L. Ungur, Y. Lan, W. Wernsdorfer, C. E. Anson, L. F. Chibotaru and A. K. Powell, Angew. Chem., Int. Ed., 2010, 49, 7583 CrossRef CAS PubMed;
(b) C. Cui, J. P. Cao, X. M. Luo, Q. F. Lin and Y. Xu, Chem.–Eur. J., 2018, 24, 15295 CrossRef CAS PubMed;
(c) M. Perfetti1, J. Rinck, G. Cucinotta, C. E. Anson, X. Gong, L. Ungur, L. Chibotaru, M.-E. Boulon1, A. K. Powell and R. Sessoli1, Front. Chem., 2019, 7, 6 CrossRef PubMed.
- S. Schmitz, J. van Leusen, N. V. Izarova, Y. Lan, W. Wernsdorfer, P. Kögerler and K. Y. Monakhov, Dalton Trans., 2016, 45, 16148 RSC.
-
(a) S. T. Zheng and G. Y. Yang, Chem. Soc. Rev., 2012, 41, 7623 RSC;
(b) X. X. Li, Y. X. Wang, R. H. Wang, C. Y. Cui, C. B. Tian and G. Y. Yang, Angew. Chem., Int. Ed., 2016, 55, 6462 CrossRef CAS PubMed.
- A. P. Ginsberg, Inorg. Synth., 1990, 27, 87 Search PubMed.
-
(a) J. X. Lin, J. Lü, H. X. Yang and R. Cao, Cryst. Growth Des., 2010, 10, 1966 CrossRef CAS;
(b) X. X. Li, L. Chen, W. H. Fang and G. Y. Yang, Acta Chim. Sin., 2013, 71, 179 CrossRef CAS.
- I. D. Brown and D. Altermatt, Acta Crystallogr., Sect. B: Struct. Sci., 1985, 41, 244 CrossRef.
- X. Li and R. Cao, J. Solid State Chem., 2012, 196, 182 CrossRef CAS.
-
(a) Y. W. Li, L. Y. Guo, H. F. Su, M. Jagodič, M. Luo, X. Q. Zhou, S. Y. Zeng, C. H. Tung, D. Sun and L. S. Zheng, Inorg. Chem., 2017, 56, 2481 CrossRef CAS PubMed;
(b) Y. Ghandour, A. B. Khelifa, M. S. Belkhiria, C. Daiguebonne, S. Freslon, O. Guillou and T. Roisnel, Polyhedron, 2016, 115, 1 CrossRef CAS.
- G. E. Kostakis and A. K. Powell, Coord. Chem. Rev., 2009, 253, 2686 CrossRef CAS.
- G. E. Kostakis, V. A. Blatov and D. M. Proseprio, Dalton Trans., 2012, 41, 4634 RSC.
- C. Wu, S. Datta, W. Wernsdorfer, G. H. Lee, S. Hill and E. C. Yang, Dalton Trans., 2010, 39, 10160 RSC.
- V. A. Grillo, Z. Sun, K. Folting, D. N. Hendrickson and G. Christou, Chem. Commun., 1996, 2233 RSC.
- E. V. Alexandrov, V. A. Blatov, A. V. Kochetkov and D. M. Proserpio, CrystEngComm, 2011, 13, 3947 RSC.
- M. Ibrahim, A. Haider, Y. Xiang, B. S. Bassil, A. M. Carey, L. Rullik, G. B. Jameson, F. Doungmene, I. M. Mbomekallė, P. de Oliveira, V. Mereacre, G. E. Kostakis, A. K. Powell and U. Kortz, Inorg. Chem., 2015, 54, 6136 CrossRef CAS PubMed.
- M. Ibrahim, A. Haider, Y. Lan, B. S. Bassil, A. M. Carey, R. Liu, G. Zhang, B. Keita, W. Li, G. E. Kostakis, A. K. Powell and U. Kortz, Inorg. Chem., 2014, 53, 5179 CrossRef CAS PubMed.
-
(a) R. Thouvenot, M. Fournier, R. Franck and C. Rocchiccioli-Deltcheff, Inorg. Chem., 1984, 23, 598 CrossRef CAS;
(b) T. Yang, X. X. Li and S. T. Zheng, Chin. J. Struct. Chem., 2017, 36, 1729 CAS.
- X. Li, H. Xu, F. Kong and R. Wang, Angew. Chem., Int. Ed., 2013, 52, 13769 CrossRef CAS PubMed.
- V. M. Huxter, T. Mirkovic, P. S. Nair and G. D. Scholes, Adv. Mater., 2008, 20, 2439 CrossRef CAS.
- S. Y. Lin, G. F. Xu, L. Zhao, Y. N. Guo, Y. Guo and J. Tang, Dalton Trans., 2011, 40, 8213 RSC.
- B. Gil-Hernández, S. Savvin, G. Makhloufi, P. Núñez, C. Janiak and J. Sanchiz, Inorg. Chem., 2015, 54, 1597 CrossRef PubMed.
- W. H. Fang, L. Zhang, J. Zhang and G. Y. Yang, Chem.–Eur. J., 2016, 22, 2611 CrossRef CAS PubMed.
- Z. Li, X. X. Li, T. Yang, Z. W. Cai and S. T. Zheng, Angew. Chem., Int. Ed., 2017, 129, 2708 CrossRef.
- Z. Li, X. X. Li and S. T. Zheng, Inorg. Chem. Commun., 2017, 80, 27 CrossRef CAS.
- K. D. Kreuer, A. Rabenau and W. Weppner, Angew. Chem., Int. Ed., 1982, 21, 208 CrossRef.
- Z. Li, L. D. Lin, H. Yu, X. X. Li and S. T. Zheng, Angew. Chem., Int. Ed., 2018, 57, 15777 CrossRef CAS PubMed.
- K. Niinomi, S. Miyazawa, M. Hibino, N. Mizuno and S. Uchida, Inorg. Chem., 2017, 56, 15187 CrossRef CAS PubMed.
Footnote |
† Electronic supplementary information (ESI) available: Additional characterizations, additional tables, structural figures, and spectrum data. CCDC 1892101 and 1892102. For ESI and crystallographic data in CIF or other electronic format see DOI: 10.1039/c9ra01731c |
|
This journal is © The Royal Society of Chemistry 2019 |
Click here to see how this site uses Cookies. View our privacy policy here.