DOI:
10.1039/C9RA01787A
(Paper)
RSC Adv., 2019,
9, 12146-12152
Pseudonectrins A–D, heptaketides from an endophytic fungus Nectria pseudotrichia†
Received
8th March 2019
, Accepted 8th April 2019
First published on 17th April 2019
Abstract
Four new heptaketides, pseudonectrins A–D (1–4), and four known compounds (5–8) were isolated from cultures of an endophytic fungus Nectria pseudotrichia. Their structures were elucidated primarily by NMR experiments. The absolute configurations of 1–3 and 4 were assigned by electronic circular dichroism calculations and the modified Mosher method, respectively. Compound 1–3 showed moderate cytotoxicity, with IC50 values of 11.6–41.2 μM.
Introduction
Heptaketides are a subgroup of polyketides showing diverse structural features and biological effects. To date, a variety of heptaketides including pyranonaphthoquinones,1–3 naphthoquinones,4 and other rearranged,5 ring-opened,6,7 or dimeric8 derivatives, have been encountered as fungal secondary metabolites, and showed a broad spectrum of biological activities, such as antibacterial, antifungal, and antitumor effects.1–4 Natural products incorporating a quinone moiety have been the subject of intensive investigations due to their potent antitumor activity. Notable examples are clinically used drugs anthracyclines and mitomycins,9 and vitamin K and its synthetic derivative menadione.10–12
The species of fungal genus Nectria are well-known for the production of highly colored naphthoquinone derivatives structurally related to fusarubin and bostrycoidin.10,13–18 While Nectria pseudotrichia, usually considered as a plant pathogen, has been reported to produce naphthoquinones, isocoumarins, and terpenoids.19–21 During our continuous search for new cytotoxic metabolites from the plant endophytic fungi,22–29 a strain of Nectria pseudotrichia, isolated from the twigs of an identified tree on Tiantangzhai Mountain, Anhui, People's Republic of China, was grown in a solid-substrate fermentation culture. An ethyl acetate (EtOAc) extract of the culture showed cytotoxic effects towards a small panel of four human tumor cell lines. Fractionation of the extract afforded four new heptaketides, which we named pseudonectrins A–D (1–4; Fig. 1), and four known compounds (5–8; Fig. 1). Details of the isolation, structure elucidation, and cytotoxicity evaluation of these compounds are reported herein.
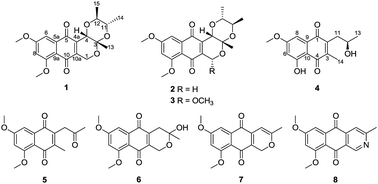 |
| Fig. 1 Structures of compounds 1–8. | |
Results and discussion
Pseudonectrin A (1) was assigned a molecular formula of C20H22O7 (10 degrees of unsaturation) by HRESIMS. Its UV spectrum showed absorptions at 216, 267, and 415 nm, implying the presence of a pyranonaphthoquinone moiety.30 Its IR absorption bands at 1665 cm−1 suggested the presence of quinone carbonyl functions. Analysis of its NMR data (Table 1) revealed the presence of five methyl groups including two O-methyls, one oxygenated methylene, three oxymethines, one doubly oxygenated quaternary carbon (δC 95.2), eight aromatic carbons with two oxygenated (δC 162.2 and 165.1) and two protonated (δC 103.9 and 104.2), and two ketone carbons (δC 181.7 and 182.9). These data accounted for six of the 10 unsaturation calculated from the molecular formula, which suggested that 1 was a tetracyclic compound. The 1H–1H COSY NMR data showed isolated spin-system of C-11–C-12 (including C-14 and C-15). On the basis of HMBC correlations from H-6 to C-5, C-5a, and C-9a, and from H-8 to C-9a and C-10, a 1,2,3,5-tetrasubstituted phenyl unit fusing at C-5a/C-9a was deduced. While those correlations from H-1a to C-3, C-4a, and C-10a, H-1b to C-4a, and C-10a, and from H-4 to C-4a, and C-10a established a pyran unit fused to the 1,4-naphthoquinone moiety at C-4a/C-10a, completing the pyranonaphthoquinone partial structure in 1. The 1,4-dioxane moiety was fused to the pyran ring at C-3/C-4 as evidenced by the correlations from H-4 to C-12, and a four-bond W-type correlation observed from H-13 to C-11 in the HMBC spectrum. Other correlations from the two oxygenated methyl protons to C-7 and C-9, respectively, indicated that these two carbons each bear a methoxy group, while those from H3-13 to C-3 located the C-13 methyl group at C-3. On the basis of these data, the gross structure of 1 was established as shown.
Table 1 NMR data of 1–4
No. |
1 |
2 |
3 |
4 |
δCa, type |
δHb (J in Hz) |
δCa, type |
δHb (J in Hz) |
δCa, type |
δHb (J in Hz) |
δCa, type |
δHb (J in Hz) |
Recorded at 150 MHz. Recorded at 600 MHz. |
1a |
62.3, CH2 |
5.03, d (20.3) |
62.5, CH2 |
5.03, d (20.5) |
96.2, CH |
5.74, s |
185.2, qC |
|
1b |
4.67, d (20.3) |
4.68, d (20.5) |
2 |
|
|
|
|
|
|
144.6, qC |
|
3 |
95.2, qC |
|
94.9, qC |
|
95.1, qC |
|
145.7, qC |
|
4 |
58.7, CH |
4.58, s |
66.5, CH |
4.44, s |
66.3, CH |
4.42, s |
188.6, qC |
|
4a |
136.0, qC |
|
135.8, qC |
|
136.3, qC |
|
|
|
5 |
182.9, qC |
|
182.7, qC |
|
183.6, qC |
|
164.2, qC |
|
5a |
136.2, qC |
|
136.2, qC |
|
135.8, qC |
|
|
|
6 |
103.9, CH |
7.28, d (2.4) |
104.0, CH |
7.29, d (2.4) |
103.5, CH |
7.28, d (2.4) |
106.1, CH |
6.62, d (2.0) |
7 |
165.1, qC |
|
165.1, qC |
|
164.9, qC |
|
165.9, qC |
|
8 |
104.2, CH |
6.71, d (2.4) |
104.1, CH |
6.71, d (2.4) |
104.5, CH |
6.73, d (2.4) |
107.8, CH |
7.16, d (2.0) |
9 |
162.2, qC |
|
162.2, qC |
|
162.2, qC |
|
133.6, qC |
|
9a |
114.2, qC |
|
114.3, qC |
|
114.8, qC |
|
|
|
10 |
181.7, qC |
|
181.8, qC |
|
180.9, qC |
|
109.7, qC |
|
10a |
145.7, qC |
|
145.6, qC |
|
141.8, qC |
|
|
|
11a |
66.6, CH |
4.54, dq (3.0, 6.7) |
70.7, CH |
3.94, dq (6.3, 9.0) |
70.7, CH |
3.90, dq (6.1, 9.0) |
36.8, CH2 |
2.81, s |
11b |
2.80, d (2.2) |
12 |
72.0, CH |
3.84, dq (3.0, 6.7) |
76.9, CH |
3.52, dq (6.3, 9.0) |
76.6, CH |
3.48, dq (6.1, 9.0) |
67.9, CH |
4.04, m |
13 |
20.9, CH3 |
1.29, s |
20.8, CH3 |
1.27, s |
25.2, CH3 |
1.43, s |
24.4, CH3 |
1.31, d (6.1) |
14 |
10.6, CH3 |
1.41, d (6.7) |
17.3, CH3 |
1.14, d (6.3) |
17.3, CH3 |
1.16, d (6.4) |
12.9, CH3 |
2.21, s |
15 |
16.8, CH3 |
1.08, d (6.7) |
17.2, CH3 |
1.14, d (6.3) |
17.2, CH3 |
1.11, d (6.4) |
|
|
1-OCH3 |
|
|
|
|
57.0, CH3 |
3.62, s |
|
|
7-OCH3 |
56.6, CH3 |
3.94, s |
56.6, CH3 |
3.95, s |
56.6, CH3 |
3.95, s |
56.1, CH3 |
3.89, s |
9-OCH3 |
56.1, CH3 |
3.95, s |
56.1, CH3 |
3.95, s |
56.1, CH3 |
3.95, s |
|
|
OH-5 |
|
|
|
|
|
|
|
12.37, s |
The relative configuration of 1 was proposed by analysis of the 1H–1H coupling constants (Table 1) and NOESY correlations (Fig. 2). NOESY correlations of H-1b with H3-13 and of H-4 with H3-13 and H3-15 indicated that these protons are all on the same face of the ring system, whereas those of H-12 with H3-14 placed the protons on the opposite face. In addition, the small coupling constant observed between H-11 and H-12 (3.0 Hz) suggested their cis relationship,31 thereby establishing the relative configuration of 1.
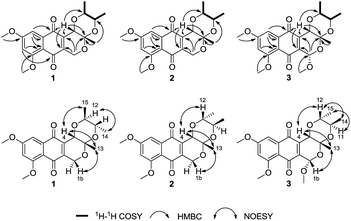 |
| Fig. 2 Key 1H–1H COSY, HMBC and NOESY correlations for compounds 1–3. | |
The absolute configuration of 1 was deduced by comparison of the experimental and simulated electronic circular dichroism (ECD) spectra calculated using the time-dependent density functional theory (TDDFT).32 The ECD spectra of the two possible enantiomers 1a and 1b (Fig. S25†) were calculated. A random conformational analysis was performed for 1a and 1b using the MMFF94 force field followed by reoptimization at the B3LYP/6-311G(d,p) level afforded the lowest energy conformers (Fig. S25†). The overall calculated ECD spectra of 1a and 1b were then generated according to Boltzmann weighting of their lowest energy conformers by their relative energies (Fig. 3). The experimental CD spectrum of 1 correlated well to the calculated ECD curve of (3S,4S,11S,12S)-1 (1a; Fig. 3), suggesting the 3S,4S,11S,12S absolute configuration for 1.
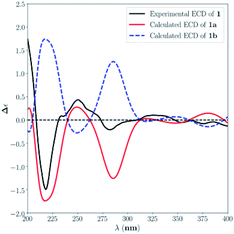 |
| Fig. 3 Experimental CD spectrum of 1 in MeOH and the calculated ECD spectra of 1a and 1b. | |
Pseudonectrin B (2) was determined to have the same molecular formula C20H22O7 (10 degrees of unsaturation) as 1 by HRESIMS. Analysis of its 1H and 13C NMR data (Table 1) revealed nearly identical structural features to those found in 1, except that the chemical shift values for the C-11 and C-12 oxymethines in 2 (δH/δC 3.94/70.7 and 3.52/76.9) were different from those in 1 (δH/δC 4.54/66.6 and 3.84/72.0). Interpretation of the NMR data established the same planar structure as 1, which was supported by relevant 1H–1H COSY and HMBC data, suggesting that 2 is a stereoisomer of 1. The relative configuration of 2 was also proposed by analysis of the 1H–1H coupling constants and NOESY data. NOESY correlations of H-1b with H3-13 and of H-4 with H3-13 and H-12 indicated that these protons are on the same face of the molecule. While a large trans-diaxial-type coupling constant of 9.0 Hz observed between H-11 and H-12 revealed that these protons were axially oriented.31 Therefore, the relative configuration of 2 was proposed.
The absolute configuration of 2 was similarly deduced by comparison of the experimental CD spectrum with the simulated ECD spectra predicted using the TDDFT at the B3LYP/6-311G(d,p) level. The ECD spectra of the four possible isomers 2a–d (Fig. S26†) were calculated to represent all possible configurations. The experimental CD spectrum of 2 was nearly identical to that calculated for 2a (Fig. 4), suggesting that 2 has the 3S,4S,11R,12R absolute configuration.
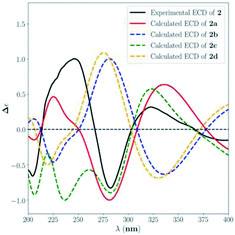 |
| Fig. 4 Experimental CD spectrum of 2 in MeOH and the calculated ECD spectra of 2a–d. | |
The molecular formula of pseudonectrin C (3) was determined to be C21H24O8 (10 degrees of unsaturation) based on HRESIMS and the NMR data (Table 1), which is 30 mass units higher than that of 2. Analysis of the 1H and 13C NMR data for 3 revealed the presence of structural features similar to those found in 2, except that H-1a (δ 5.03) was replaced by a methoxy unit (δH/δC 3.62/57.0), and this observation was supported by the HMBC correlations from these newly observed methoxy protons to C-1. Therefore, the gross structure of 3 was established. The relative configuration of 3 was also deduced by analysis of 1H–1H coupling constants and NOESY data, and by comparison of its 1H NMR data with those of 2.
The absolute configuration of 3 was assigned by comparison of the experimental CD spectrum with the simulated ECD spectra generated by excited state calculation using TDDFT. The ECD spectra of the two enantiomers 3a and 3b (Fig. S27†) were calculated to represent all possible configurations. The MMFF94 conformational search followed by reoptimization at the B3LYP/6-311G(d,p) level afforded the lowest-energy conformers (Fig. S27†). The overall ECD spectra were then generated according to Boltzmann weighting of each conformer. The CD spectrum of 3 correlated well to the calculated curve of 3a (Fig. 5), suggesting the 1S,3R,4S,11R,12R absolute configuration.
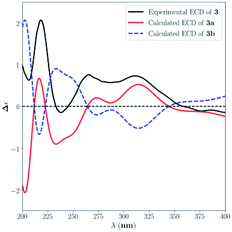 |
| Fig. 5 Experimental CD spectrum of 3 in MeOH and the calculated ECD spectra of 3a and 3b. | |
Pseudonectrin D (4) gave a molecular formula of C15H16O5 (eight degrees of unsaturation) by analysis of its HRESIMS. The 1H and 13C NMR data of 4 revealed structural features closely resembled those of the known compound, 2-acetonyl-5,7-dimethoxy-3-methyl-1,4-naphthoquinone (5).33 Comparison of their NMR data revealed the presence of an oxymethine (δH/δC 4.04/67.9) in 4 instead of the ketone functionality in 5 (δC 203.9), suggesting that the C-12 carbonyl group in 5 was reduced to a free hydroxy group in 4. In addition, the methoxy unit (δH/δC 3.96/56.4) in 5 was replaced by a phenolic hydroxy in 4 (δH 12.37), which was supported by the HMBC correlations from the newly observed phenolic proton to C-5, C-6, and C-10. Therefore, the gross structure of 4 was proposed. The absolute configuration for the C-12 secondary alcohol in 4 was assigned using the modified Mosher method.34,35 Treatment of 4 with (R)- and (S)-MTPA–Cl afforded the (S)-(4a) and (R)-MTPA (4b) esters, respectively. The difference in chemical shift values (Δδ = δS − δR) for the diastereomeric esters 4a and 4b was calculated to assign the 12R absolute configuration (Fig. 6).
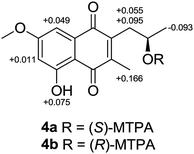 |
| Fig. 6 Δδ values (in ppm) = δS − δR for (S)- and (R)-MPTA esters of 4. | |
The other known compounds 6–8 isolated from the crude extract were identified as herbarin (6),6 dehydroherbarin (7),33 and scorpinone (8),36 respectively, by comparison of their NMR and MS data with those reported. The low specific rotation value of 6 {[α]25D +3.7 (c 0.1, MeOH)} was consistent with the reported one {[α]25D +4.8 (c 0.06, CHCl3)},6 suggesting that the sample is racemic due to the labile cyclic hemiacetal moiety.5
Compounds 1–8 were tested for cytotoxicity against the human tumor cell lines, MCF-7 (breast cancer), HepG2 (hepatocellular carcinoma), SH-SY5Y (glioma), and ACHN (rental cell carcinoma). Compound 1–3, 6, and 7 showed moderate cytotoxic effects, with IC50 values of 11.6–45.9 μM (the positive control cisplatin showed IC50 values of 11.7–18.3 μM).
Experimental
General experimental procedures
Optical rotations were measured on a Rudolph Research Analytical automatic polarimeter, and UV data were recorded on a Shimadzu Biospec-1601 spectrophotometer. CD spectra were recorded on a JASCO J-815 spectropolarimeter. IR data were recorded using a Nicolet Magna-IR 750 spectrophotometer. 1H and 13C NMR spectra were acquired with Bruker Avance III-600 spectrometers using solvent signals (CDCl3: δH 7.26/δC 77.16) as references. The HSQC and HMBC experiments were optimized for 145.0 and 8.0 Hz, respectively. ESIMS and HRESIMS data were obtained on an Agilent Accurate-Mass-Q-TOF LC/MS G6550 instrument equipped with an ESI source. HPLC analysis and separation were performed using an Agilent 1260 instrument equipped with a variable-wavelength UV detector.
Fungal material
The culture of N. pseudotrichia was isolated from twigs of an identified tree on Tiantangzhai Mountain, Anhui, People's Republic of China, in August 2011. The isolate was identified based on morphology and sequence (GenBank accession no. MK305970) analysis of the ITS region of the rDNA. The fungal strain was cultured on slants of potato dextrose agar (PDA) at 25 °C for 10 days. Agar plugs were cut into small pieces (about 0.5 × 0.5 × 0.5 cm3) under aseptic conditions, and 25 pieces were used to inoculate in five 250 mL Erlenmeyer flasks, each containing 50 mL of media (0.4% glucose, 1% malt extract, and 0.4% yeast extract), and the final pH of the media was adjusted to 6.5 and sterilized by autoclave. Five flasks of the inoculated media were incubated at 25 °C on a rotary shaker at 170 rpm for 5 days to prepare the seed culture. Fermentation was carried out in 20 Fernbach flasks (500 mL) each containing 80 g of rice. Distilled H2O (120 mL) was added to each flask, and the contents were soaked overnight before autoclaving at 15 psi for 30 min. After cooling to room temperature, each flask was inoculated with 5.0 mL of the spore inoculum and incubated at 25 °C for 40 days.
Extraction and isolation
The fermentation material was extracted repeatedly with EtOAc (4 × 4.0 L), and the organic solvent was evaporated to dryness under vacuum to afford 8.6 g of crude extract. The crude extract was fractionated by silica gel vacuum liquid chromatography (VLC) using petroleum ether–EtOAc–MeOH gradient elution. The fraction (45 mg) eluted with 4
:
1 petroleum ether–EtOAc was separated by Sephadex LH-20 column chromatography (CC) eluting with 1
:
1 CH2Cl2–MeOH and the resulting subfractions were combined and purified by semipreparative RP HPLC (Agilent Zorbax SB-C18 column; 5 μm; 9.4 × 250 mm; 78% MeOH in H2O for 30 min; 2 mL min−1) to afford 7 (2.0 mg, tR 24.1 min). The fraction (110 mg) eluted with 3
:
1 petroleum ether–EtOAc was separated by Sephadex LH-20 CC eluting with 1
:
1 CH2Cl2–MeOH, and the resulting subfractions were combined and further purified by RP HPLC to afford 1 (1.0 mg, tR 38.0 min; 42% CH3CN in H2O for 50 min; 2 mL min−1), 2 (4.5 mg, tR 42.5 min; the same gradient as in purification of 1), 3 (1.8 mg, tR 32.5 min; 65% CH3CN in H2O for 40 min; 2 mL min−1) and 8 (5.5 mg, tR 22.5 min; the same gradient as in purification of 1). The fraction (80 mg) eluted with 3
:
2 petroleum ether–EtOAc was separated by Sephadex LH-20 CC eluting with 1
:
1 CH2Cl2–MeOH, and the subfractions were combined and purified by RP HPLC to afford 4 (2.8 mg, tR 53.5 min; 40% CH3CN in H2O for 60 min; 2 mL min−1) and 5 (4.0 mg, tR 38.0 min; 51% MeOH in H2O for 45 min; 2 mL min−1). The fraction (50 mg) eluted with 1
:
1 petroleum ether–EtOAc was separated by Sephadex LH-20 CC eluting with 1
:
1 CH2Cl2–MeOH, and the subfractions were further purified by RP HPLC to afford 6 (4.0 mg, tR 25.2 min; 57% MeOH in H2O for 30 min; 2 mL min−1).
Pseudonectrin A (1). Yellow powder, mp 213–214 °C; [α]25D +37.0 (c 0.1, MeOH); UV (MeOH) λmax (log
ε) 216 (4.39), 267 (4.02), 415 (3.39) nm; CD (c 5.3 × 10−4 M, MeOH) λmax (Δε) 218 (−1.50), 250 (+0.44), 282 (−0.21) 325 (+0.10) nm; IR (neat) νmax 2928, 1665, 1592, 1456, 1332, 1276, 1200, 1094, 960, 829, 728 cm−1; 1H and 13C NMR data see Table 1; HMBC data (CDCl3, 600 MHz) H-1a → C-3, 4, 4a, 10, 10a; H-1b → C-4, 4a, 5, 10a; H-4 → C-3, 4a, 5, 10a, 12, 13; H-6 → C-5, 5a, 7, 8, 9a; H-8 → C-6, 7, 9, 9a, 10; H-11 → C-12, 14; H-12 → C-4; H3-13 → C-3, 4, 11; H3-14 → C-11, 12; H3-15 → C-11, 12; 7-OCH3 → C-7; 9-OCH3 → C-9; NOESY correlations (CDCl3, 600 MHz) H-1b ↔ H3-13; H-4 ↔ H3-13, H3-15; H-12 ↔ H3-14; HRESIMS m/z 375.1441 [M + H]+ (calcd for C20H23O7, 375.1438).
Pseudonectrin B (2). Yellow powder, mp 219–220 °C; [α]25D −96.65 (c 0.1, MeOH); UV (MeOH) λmax (log
ε) 217 (4.39), 268 (4.03), 415 (3.44) nm; CD (c 5.3 × 10−4 M, MeOH) λmax (Δε) 204 (−0.06), 246 (+1.00), 282 (−0.83) 322 (+0.32) nm; IR (neat) νmax 2916, 1653, 1595, 1457, 1331, 1270, 1216, 1094, 927, 828, 730 cm−1; 1H and 13C NMR data see Table 1; HMBC data (CDCl3, 600 MHz) H-1a → C-3, 4, 4a, 5, 10a; H-1b→ C-4, 4a, 5, 10a; H-4 → C-3, 4a, 5, 10a, 12; H-6 → C-5, 5a, 7, 8, 9a; H-8 → C-6, 7, 9, 9a; H-11 → C-12, 14; H-12 → C-4, 11, 15; H3-13 → C-3, 4, 11; H3-14 → C-11, 12; H3-15 → C-11, 12; 7-OCH3 → C-7; 9-OCH3 → C-9; NOESY correlations (CDCl3, 600 MHz) H-1b ↔ H3-13; H-4 ↔ H-12, H3-13; HRESIMS m/z 375.1443 [M + H]+ (calcd for C20H23O7, 375.1438).
Pseudonectrin C (3). Yellow powder, mp 220–221 °C; [α]25D +74.53 (c 0.1, MeOH); UV (MeOH) λmax (log
ε) 218 (4.36), 264 (4.02), 415 (3.43) nm; CD (c 7.2 × 10−4 M, MeOH) λmax (Δε) 218 (+2.07), 238 (−0.15), 265 (+0.76) 311 (+0.73) nm; IR (neat) νmax 2919, 1662, 1597, 1467, 1330, 1269, 1197, 1099, 912, 872, 735 cm−1; 1H and 13C NMR data see Table 1; HMBC data (CDCl3, 600 MHz) H-1 → C-3, 4a, 10a; H-4 → C-3, 4a, 5, 10a, 12, 13; H-6 → C-5, 5a, 7, 8, 9a; H-8 → C-6, 7, 9, 9a; H-11 → C-12, 15; H-12 → C-11, 14; H3-13 → C-3, 4; H3-14 → C-3, 11, 12; H3-15 → C-11, 12; 1-OCH3 → C-1; 7-OCH3 → C-7; 9-OCH3 → C-9; NOESY correlations (CDCl3, 600 MHz) H-1 ↔ H3-13; H-4 ↔ H-12, H3-13; H-11 ↔ H3-15; H-12 ↔ H3-14; HRESIMS m/z 427.1362 [M + Na]+ (calcd for C21H24O8Na, 427.1363).
Pseudonectrin D (4). Yellow powder, mp 130–131 °C; [α]25D +40.0 (c 0.1, MeOH); UV (MeOH) λmax (log
ε) 217 (4.14), 268 (3.61), 290 (3.48), 427 (3.11) nm; CD (c 1.8 × 10−3 M, MeOH) λmax (Δε) 223 (+0.32), 252 (+0.28), 299 (−0.10), 351 (+0.10), 372 (+0.09) nm; IR (neat) νmax 3384 (br), 2937, 1663, 1631, 1606, 1449, 1338, 1316, 1209, 1031 cm−1; 1H and 13C NMR data see Table 1; HMBC data (CDCl3, 600 MHz) H-6 → C-5, 8; H-8 → C-1, 6, 7, 10; H-11a → C-1, 2, 12, 13; H-11b → C-1, 2, 12, 13; H-12 → C-2; H3-13 → C-11, 12; H3-14 → C-3, 4; 7-OCH3 → C-7; 5-OH → C-5, 6, 10; HRESIMS m/z 277.1073 [M + H]+ (calcd for C15H17O5, 277.1071).
Preparation of (S) and (R)-MTPA esters
A sample of 4 (0.6 mg, 0.002 mmol) was dissolved in 500 μL of anhydrous pyridine. (R)-MTPA–Cl (5.0 μL, 0.026 mmol) was quickly added under nitrogen protection, the mixture was stirred at room temperature for 5 h. The mixture was purified by RP HPLC (Agilent Zorbax SB-C18 column; 5 μm; 9.4 × 250 mm; 95% CH3CN in H2O for 15 min; 2 mL min−1) to afford (S)-MTPA ester 4a (0.3 mg, tR 12.0 min): 1H NMR (CDCl3, 600 MHz) δ 12.34 (1H, s, 5-OH), 7.17 (1H, d, J = 2.3 Hz, H-8), 6.65 (1H, d, J = 2.3 Hz, H-6), 5.30 (1H, m, H-12), 3.91 (3H, s, 7-OCH3), 2.98 (1H, dd, J = 13.4, 4.5 Hz, H-11a), 2.85 (1H, dd, J = 13.4, 9.5 Hz H-11b), 2.01 (3H, s, H3-14), 1.41 (3H, d, J = 6.1 Hz, H3-13).
Similarly, a sample of 4 (0.6 mg, 0.002 mmol), (S)-MTPA–Cl (5.0 μL, 0.026 mmol), and pyridine (500 μL) were processed as described above for 4a. The mixture was purified by RP HPLC (Agilent Zorbax SB-C18 column; 5 μm; 9.4 × 250 mm; 95% CH3CN in H2O for 15 min; 2 mL min−1) to afford (R)-MTPA ester 4b (0.3 mg, tR 11.5 min): 1H NMR (CDCl3, 600 MHz) δ 12.26 (1H, s, 5-OH), 7.12 (1H, d, J = 2.4 Hz, H-8), 6.64 (1H, d, J = 2.4 Hz, H-6), 5.27 (1H, m, H-12), 3.92 (3H, s, 7-OCH3), 2.93 (1H, dd, J = 13.4, 3.8 Hz, H-11a), 2.75 (1H, dd, J = 13.4, 10.2 Hz, H-11b), 1.84 (3H, s, H3-14), 1.50 (3H, d, J = 6.1 Hz, H3-13).
Computational details
Conformational analyses for 1–3 were performed via the Molecular Operating Environment (MOE) version 2009.10 (Chemical Computing Group, Canada) software package with LowModeMD at the MMFF94 force field. The MMFF94 conformers were further optimized using TDDFT at the B3LYP/6-311G(d,p) basis set level in MeOH with the IEFPCM model. The stationary points have been checked as the true minima of the potential energy surface by verifying that they do not exhibit vibrational imaginary frequencies. The 25 lowest electronic transitions were calculated, and the rotational strengths of each electronic excitation were given using both dipole length and velocity representations. ECD spectra were simulated in SpecDis23 (ref. 37) using a Gaussian function with half-bandwidths of 0.30 eV. The overall ECD spectra were then generated according to Boltzmann weighting of each conformer. The systematic errors in the prediction of wavelength and excited-state energies are compensated by employing UV correlation. All quantum computations were performed using the Gaussian 09 package.38
Cytotoxicity assays
The cytotoxic activity of compounds 1–8 were tested using 96 well plates according to a literature MTS method with slight modification.39 Cells were seeded in 96 well plates at a density of 1.0 × 104 cells per well in 100 μL of complete culture medium. After cell attachment overnight, the medium was removed, and each well was treated with 100 μL of medium containing 0.1% DMSO or appropriate concentrations of the test compounds and the positive control cisplatin and incubated with cells at 37 °C for 48 h in a 5% CO2-containing incubator. Proliferation was assessed by adding 20 μL of MTS (Promega) to each well in the dark, after 90 min of incubation at 37 °C. The optical density was recorded on a microplate reader at 490 nm. Three duplicate wells were used for each concentration, and all the tests were repeated three times.
Conclusions
Pseudonectrins A–C (1–3) are structurally related to a pyranonaphthoquinone metabolite herbarin (6), but differ in possessing an additional 1,4-dioxane ring fused to the dihydropyran moiety at C-3/C-4 of the pyranonaphthoquinone core. Natural products incorporating the 1,4-dioxane unit are relatively rare, most of which were derived from glycosides through the formation of acetal and hemiacetal between aglycone and glycosyl.40–44 To our knowledge, pyranonaphthoquinone derivatives with a 1,4-dioxane ring fusing to the dihydropyran moiety have not been reported previously. Pseudonectrin D (4) is structurally related to 2-acetonyl-5,7-dimethoxy-3-methyl-1,4-naphthoquinone (5), but differs by having a hydroxy group at C-12 instead of the carbonyl functionality. Compound 5, previously reported as synthetic compound, was isolated as a natural product for the first time. Pyranonaphthoquinone derivatives 1–3, 6, and 7 showed moderate cytotoxic effects, whereas 4 did not show detectable cytotoxicity at 50 μM (Table 2). Biogenetically, 1–8 could be originated from the nonreducing iterative polyketide synthases (NR-PKS),5,18 and the hypothetical biosynthetic pathways leading to the generation of these metabolites are illustrated in Scheme S1.†
Table 2 Cytotoxicity of compound 1–8
Compound |
IC50a (μM) |
MCF-7 |
HepG2 |
SH-SY5Y |
ACHN |
IC50 values were averaged from at least three independent experiments. No activity was detected at 50 μM. Positive control. |
1 |
19.2 ± 4.5 |
12.5 ± 3.5 |
17.2 ± 5.1 |
NAb |
2 |
34.2 ± 5.8 |
29.7 ± 4.7 |
19.0 ± 2.6 |
16.8 ± 2.1 |
3 |
41.2 ± 4.4 |
13.3 ± 2.2 |
NAb |
11.6 ± 2.5 |
4 |
NAb |
NAb |
NAb |
NAb |
5 |
29.9 ± 3.4 |
NAb |
NAb |
NAb |
6 |
19.2 ± 4.5 |
45.9 ± 4.0 |
33.0 ± 4.2 |
37.0 ± 2.0 |
7 |
20.6 ± 4.4 |
NAb |
19.3 ± 3.8 |
12.6 ± 3.3 |
8 |
NAb |
NAb |
NAb |
NAb |
Cisplatinc |
16.2 ± 2.6 |
12.8 ± 0.8 |
18.3 ± 3.6 |
11.7 ± 2.8 |
Conflicts of interest
The authors declare no conflict interest.
Acknowledgements
P. F., T. Z., S. Z., F. R., and Y. Z. were supported in part by the National Program of Drug Research and Development (2012ZX09301-003); Y. C. was supported by the CAMS Innovation Fund for Medical Sciences (2018-I2M-3-005).
Notes and references
- M. A. Brimble, L. J. Duncalf and M. R. Nairn, Nat. Prod. Rep., 1999, 16, 267–281 RSC.
- J. Sperry, P. Bachu and M. A. Brimble, Nat. Prod. Rep., 2008, 25, 376–400 RSC.
- B. J. Naysmith, P. A. Hume, J. Sperry and M. A. Brimble, Nat. Prod. Rep., 2017, 34, 25–61 RSC.
- A. G. Medentsev and V. K. Akimenko, Phytochemistry, 1998, 47, 935–959 CrossRef CAS PubMed.
- W. Geng, X. Wang, T. Kurtán, A. Mándi, H. Tang, B. Schulz, P. Sun and W. Zhang, J. Nat. Prod., 2012, 75, 1828–1832 CrossRef CAS PubMed.
- P. A. Paranagama, E. M. K. Wijeratne, A. M. Burns, M. T. Marron, M. K. Gunatilaka, A. E. Arnold and A. A. L. Gunatilaka, J. Nat. Prod., 2007, 70, 1700–1705 CrossRef CAS PubMed.
- L. Wang, J. Dong, H. Song, K. Shen, L. Wang, R. Sun, C. Wang, Y. Gao, G. Li, L. Li and K. Zhang, Planta Med., 2009, 75, 1339–1343 CrossRef CAS PubMed.
- S. Lösgen, O. Schlörke, K. Meindl, R. Herbst-Irmer and A. Zeeck, Eur. J. Org. Chem., 2007, 2191–2196 CrossRef.
- A. M. Heapy, A. V. Patterson, J. B. Smaill, S. M. F. Jamieson, C. P. Guise, J. Sperry, P. A. Hume, K. Rathwell and M. A. Brimble, Bioorg. Med. Chem., 2013, 21, 7971–7980 CrossRef CAS PubMed.
- K. Takemoto, S. Kamisuki, P. T. Chia, I. Kuriyama, Y. Mizushina and F. Sugawara, J. Nat. Prod., 2014, 77, 1992–1996 CrossRef CAS PubMed.
- F. Y. Wu, W. Liao and H. Chang, Life Sci., 1993, 52, 1797–1804 CrossRef CAS PubMed.
- H. Okayasu, M. Ishihara, M. Satoh and H. Sakagami, Anticancer Res., 2001, 21, 2387–2392 CAS.
- D. Parisot, M. Devys, J. Férézou and M. Barbier, Phytochemistry, 1983, 22, 1301–1303 CrossRef CAS.
- D. Parisot, M. Devys and M. Barbier, Phytochemistry, 1990, 29, 3364–3365 CrossRef CAS.
- D. Parisot, M. Devys and M. Barbier, J. Antibiot., 1991, 44, 103–107 CrossRef CAS PubMed.
- D. Parisot, M. Devys and M. Barbier, J. Antibiot., 1992, 45, 1799–1801 CrossRef CAS PubMed.
- J. Kornsakulkarn, K. Dolsophon, N. Boonyuen, T. Boonruangprapa, P. Rachtawee, S. Prabpai, P. Kongsaeree and C. Thongpanchang, Tetrahedron, 2011, 67, 7540–7547 CrossRef CAS.
- T. Awakawa, T. Kaji, T. Wakimoto and I. Abe, Bioorg. Med. Chem. Lett., 2012, 22, 4338–4340 CrossRef CAS PubMed.
- N. R. Ariefta, P. Kristiana, H. H. Nurjanto, H. Momma, E. Kwon, T. Ashitani, K. Tawaraya, T. Murayama, T. Koseki, H. Furuno, N. Usukhbayar, K. Kimura and Y. Shiono, Tetrahedron Lett., 2017, 58, 4082–4086 CrossRef CAS.
- N. R. Ariefta, P. Kristiana, T. Aboshi, T. Murayama, K. Tawaraya, T. Koseki, N. Kurisawa, K. Kimura and Y. Shiono, Fitoterapia, 2018, 127, 356–361 CrossRef CAS PubMed.
- B. B. Cota, L. G. Tunes, D. N. B. Maia, J. P. Ramos, D. M. Oliveira, M. Kohlhoff, T. M. A. Alves, E. M. Souza-Fagundes, F. F. Campos and C. L. Zani, Mem. Inst. Oswaldo Cruz, 2018, 113, 102–110 CrossRef PubMed.
- E. Li, R. Tian, S. Liu, X. Chen, L. Guo and Y. Che, J. Nat. Prod., 2008, 71, 664–668 CrossRef CAS PubMed.
- G. Ding, L. Jiang, L. Guo, X. Chen, H. Zhang and Y. Che, J. Nat. Prod., 2008, 71, 1861–1865 CrossRef CAS PubMed.
- L. Liu, S. Liu, L. Jiang, X. Chen, L. Guo and Y. Che, Org. Lett., 2008, 10, 1397–1400 CrossRef CAS PubMed.
- L. Liu, Y. Li, S. Liu, Z. Zheng, X. Chen, H. Zhang, L. Guo and Y. Che, Org. Lett., 2009, 11, 2836–2839 CrossRef CAS PubMed.
- L. Liu, S. Niu, X. Lu, X. Chen, H. Zhang, L. Guo and Y. Che, Chem. Commun., 2010, 46, 460–462 RSC.
- L. Liu, T. Bruhn, L. Guo, D. C. G. Götz, B. Brun, A. Stich, Y. Che and G. Bringmann, Chem.–Eur. J., 2011, 17, 2604–2613 CrossRef CAS PubMed.
- L. Liu, H. Gao, X. Chen, X. Cai, L. Yang, L. Guo, X. Yao and Y. Che, Eur. J. Org. Chem., 2010, 3302–3306 CrossRef CAS.
- L. Liu, X. Chen, D. Li, Y. Zhang, L. Li, L. Guo, Y. Cao and Y. Che, J. Nat. Prod., 2015, 78, 746–753 CrossRef CAS PubMed.
- M. V. Kadkol, K. S. Gopalkrishnan and N. Narasimhachari, J. Antibiot., 1971, 24, 245–248 CrossRef CAS PubMed.
- J. Trofast and B. Wickberg, Tetrahedron, 1977, 33, 875–879 CrossRef CAS.
- S. Zhu, F. Ren, Z. Guo, J. Liu, X. Liu, G. Liu and Y. Che, J. Nat. Prod., 2019, 82, 462–468 CrossRef CAS PubMed.
- B. Kesteleyn and N. D. Kimpe, J. Org. Chem., 2000, 65, 640–644 CrossRef CAS PubMed.
- J. A. Dale and H. S. Mosher, J. Am. Chem. Soc., 1973, 95, 512–519 CrossRef CAS.
- I. Ohtani, T. Kusumi, Y. Kashman and H. Kakisawa, J. Am. Chem. Soc., 1991, 113, 4092–4096 CrossRef CAS.
- A. Miljkovic, P. G. Mantle, D. J. Williams and B. Rassing, J. Nat. Prod., 2001, 64, 1251–1253 CrossRef CAS.
- T. Bruhn, A. Schaumlöffel, Y. Hemberger and G. Bringmann, Chirality, 2013, 25, 243–249 CrossRef CAS PubMed.
- M. J. Frisch, G. W. Trucks, H. B. Schlegel, G. E. Scuseria, M. A. Robb, J. R. Cheeseman, G. Scalmani, V. Barone, B. Mennucci, G. A. Petersson, H. Nakatsuji, M. Caricato, X. Li, H. P. Hratchian, A. F. Izmaylov, J. Bloino, G. Zheng, J. L. Sonnenberg, M. Hada, M. Ehara, K. Toyota, R. Fukuda, J. Hasegawa, M. Ishida, T. Nakajima, Y. Honda, O. Kitao, H. Nakai, T. Vreven, J. A. Montgomery Jr, J. E. Peralta, F. Ogliaro, M. Bearpark, J. J. Heyd, E. Brothers, K. N. Kudin, V. N. Staroverov, R. Kobayashi, J. Normand, K. Raghavachari, A. Rendell, J. C. Burant, S. S. Iyengar, J. Tomasi, M. Cossi, N. Rega, J. M. Millam, M. Klene, J. E. Knox, J. B. Cross, V. Bakken, C. Adamo, J. Jaramillo, R. Gomperts, R. E. Stratmann, O. Yazyev, A. J. Austin, R. Cammi, C. Pomelli, J. W. Ochterski, R. L. Martin, K. Morokuma, V. G. Zakrzewski, G. A. Voth, P. Salvador, J. J. Dannenberg, S. Dapprich, A. D. Daniels, O. Farkas, J. B. Foresman, J. V. Ortiz, J. Cioslowski and D. J. Fox, Gaussian 09, Rev D.01, Gaussian, Inc., Wallingford, CT, 2009 Search PubMed.
- N. Zhang, Y. Chen, R. Jiang, E. Li, X. Chen, Z. Xi, Y. Guo, X. Liu, Y. Zhou, Y. Che and X. Jiang, Autophagy, 2011, 7, 598–612 CrossRef CAS.
- Y. Fukuyama, M. Ochi, H. Kasai and M. Kodama, Phytochemistry, 1993, 32, 297–301 CrossRef CAS.
- H. El-askary, J. Hölzl, S. Hilal and E. El-kashoury, Phytochemistry, 1993, 34, 1399–1402 CrossRef CAS.
- Y. Tsujino, J. I. J. Ogoche, H. Tazak, T. Fujimori and K. Mori, Phytochemistry, 1995, 40, 753–756 CrossRef CAS.
- T. Lhinhatrakool and S. Sutthivaiyakit, J. Nat. Prod., 2006, 69, 1249–1251 CrossRef CAS PubMed.
- H. Kawagishi, A. Shimada, R. Shirai, K. Okamoto, F. Ojima, H. Sakamoto, Y. Ishiguro and S. Furukawa, Tetrahedron Lett., 1994, 35, 1569–1572 CrossRef CAS.
Footnote |
† Electronic supplementary information (ESI) available: UV, IR, HRESIMS, NMR spectra of compounds 1–4; ECD calculations of compounds 1–3. See DOI: 10.1039/c9ra01787a |
|
This journal is © The Royal Society of Chemistry 2019 |
Click here to see how this site uses Cookies. View our privacy policy here.