DOI:
10.1039/C9RA01884K
(Paper)
RSC Adv., 2019,
9, 14331-14342
Co-cultivation of Rhodotorula glutinis and Chlorella pyrenoidosa to improve nutrient removal and protein content by their synergistic relationship
Received
12th March 2019
, Accepted 10th April 2019
First published on 7th May 2019
Abstract
With the continuous development of the livestock breeding industry, the amount of piggery wastewater discharged increases year by year, and the pressure of controlling environmental pollution continuously increases. A novel method using a co-culture of Chlorella pyrenoidosa and Rhodotorula glutinis in piggery wastewater was proposed in this study, which was aimed at treating piggery wastewater and producing useful products. The results showed that the optimal inoculum ratio of algae to yeast was 3
:
1 in the wastewater, which achieved the removal efficiencies of 58.53%, 36.07%, 33.20% and 56.25% for ammoniacal nitrogen (NH3-N), total nitrogen (TN), total protein (TP) and chemical oxygen demand (COD), respectively, after 6 d. The synergistic relationship of C. pyrenoidosa and R. glutinis was preliminarily validated using the oxygen/carbon dioxide exchange balance and scanning electron microscopy images. The co-cultivation system gained 59.8% (w/w) protein within 5 d which can be used as a feed additive, and produces aquatic animals with better growth and quality. Thus, the 1000 litre pilot scale bioreactor was used indoors and removed 82.65% of TN, 53.51% of TP, 93.48% of NH3-N and 85.44% of COD in 21 d which gave a better performance for TN (p < 0.05) than the bench scale results. This system improves the nutrition removal and protein production efficiencies, and is a promising method for piggery wastewater treatment and the pig breeding industry.
1 Introduction
The issue of non-point source pollution in agriculture has been plaguing countries all over the world.1,2 In China, the annual discharge of piggery wastewater, which has a high chemical oxygen demand (COD) and a high level of suspended solids, is over one billion tons per year,3 and this poses serious threats to the environment. Traditional wastewater treatment technologies, including artificial wetlands, and, aerobic and anaerobic treatments, have been widely used for piggery wastewater remediation because of the effective removal efficiency of soluble organic matters and nutrients. However, the environmental problems caused by piggery wastewater still have not been solved effectively.4 The generation of secondary pollution, such as carbon dioxide (CO2) converted during the anaerobic fermentation and the incomplete degradation of a considerable amount of ammonia, phosphate and organic compounds, is one of the main concerns of traditional piggery wastewater treatment technologies. The treatment systems such as oxidation ponds, artificial wetlands, aerobic and anaerobic treatments and so on, require a high investment and high ongoing operational costs. Under the dual pressure of pollution and the market economy,5 it might not be sustainable to use traditional technologies for wastewater treatment. Therefore, there is an urgent need to explore more sustainable methods with sensible costs for piggery wastewater treatment.
Microalgae cultivation is promising for nutrient recovery in the wastewater treatment field.6 As shown in a previous study, Rawat et al.7 used Chlorella pyrenoidosa and Scenedesmus obliquus to treat the wastewater so that it could be used to cultivate valuable components. Harvested biomass, which contains abundant protein (39% w/w) and carbohydrate (22% w/w), has the potential of being used as animal feed or fertilizer. However, microalgae did not show a good performance in the removal of organic suspended solids in wastewater in another study by Rawat et al.8 Because organic suspended solids can block sunlight this considerably affected algal growth. In addition, the turbidity of the wastewater was too high, which adversely affected photosynthesis and affected the growth of the algae. It was reported that the co-cultivation of heterotrophic microorganisms and microalgae could effectively remove suspended organic solids in wastewater, and there was a 40–50% increase in the algal biomass when Rhodotorula glutinis and Scenedesmus obliquus were co-cultured to treat distillery and domestic inoculum wastewater.9 Kim et al.10 conducted an experiment that showed that after using green microalgae for 40 d, total protein (TP), total nitrogen (TN), and total organic carbon in piggery wastewater were reduced by 83.2%, 87.0%, and 12.9%, respectively. It was reported11 that Chlorella was used to treat meat processing wastewater, and the removal rates of ammonia and TN were 68–91% and 30–51%, respectively, whereas the protein content of the algal products was as high as 61–69%. However, the removal efficiency of TN was a little low. The results of the inoculum culture study with Chlorella and Rhodotorula conducted in wastewater by Cheirsilp et al.12 suggested that the COD removal rate in the inoculum culture achieved 79%, which was higher than the removal rate of Chlorella or yeast alone (60% and 71%, respectively). The cultivation of microalgal consortia to removing nutrients has a great performance in wastewater. However, as far as is known to the authors, a similar method with the co-cultivation of the yeast R. glutinis and the microalga C. pyrenoidosa to enhance the removal efficiency of TN and protein content has not been reported.
Yeast–algae is a good animal feed additive, but its application is limited because of its low yield and high cost. Microalgae are rich in protein, polysaccharides, unsaturated fatty acids, cytochromes (such as chlorophyll, astaxanthin and lutein) and other important bio-active substances, which can be widely used in animal feed.13 At present, the main functions of microalgae, such as Arthrospira (Spirulina), Chlorella, Scenedesmus, Nannochloropsis, and Cryptocaryon sinensis, used as feed additives14 are as follows: (1) they are nutrient-rich with a protein content of as high as 50% or more, which significantly increases its nutritional value,15 (2) it enriches the feed with p-carotene, astaxanthin and lutein and other high-efficiency antioxidants, which can improve the immune function of animals, and (3) it enriches the feed with polysaccharides, which regulate the life process of cells and improve the body's immunity. By feeding animals with algae or yeast biomass, it can significantly improve the saturated fatty acid content of meat, eggs and milk. Herber and Van Elswyk added up to 4.8% of golden marine algae to the diet of laying hens, which increased the docosahexaenoic acid (DHA) composition and reduced the n-6 fatty acid content. The addition of 2% microalgae in the diet increased the survival rate and weight gain rate of the chicks by 16.8% and 33.4%, respectively, so the addition of 0.8% and 4.3% microalgae increased the DHA content of each egg by 134 and 220 mg, respectively.16 The rapid development of the aquaculture industry had led to a serious shortage of feed ingredients. However, the health status of farmed animals had been severely tested. As feed accounts for more than 70% of the cost of breeding, it is the cause of much criticism and expectation of the industry. Therefore, it is a key issue for the current intensive aquaculture industry to develop a new generation of green functional healthy feeds and comprehensively upgrade the existing feed technology level to match the current high yield farming model.
Microalgae have been used as a feed additive in foreign countries for decades. However, because of the high price of microalgae in China, its promotion and application are limited. With the advancement of culture technology in recent years, especially through the research of the yeast–microalgae co-culture system, the cost has been greatly reduced. Therefore, future studies are needed to measure the feasibility for the application of the co-culture system in the feed industry. The current research on algae–yeast co-culture show that the nutrient removal efficiencies were improved and the growth of algae was improved,17–19 however, the relationship and interaction between them in piggery wastewater has not been investigated. The co-culture algae–yeast and microbiota alterations exert profound effects on wastewater treatment.20,21 Thus, integrated algae–yeast bioreactor and further studies could be performed to verify the cooperation mechanism between algae and yeast. It was hypothesized that the algae and yeast cooperation may be mutually beneficial through the processes of respiration or substrate exchange. To verify this hypothesis, a three part of study was conducted: (1) determination of the effects of activities of C. pyrenoidosa and R. glutinis on nutrient removal and wastewater properties, (2) investigation of the relationship between C. pyrenoidosa and R. glutinis, (3) verification and exploration of the co-cultivation experiments using batch tests and pilot-scale tests, and (4) experimental work to determine whether protein from the co-culture biomass improves dramatically.
2 Methods and materials
2.1 Piggery wastewater
Piggery wastewater was collected from the effluent of a pre-treated digestion reactor at the Aochun Dairy Co., Ltd. (Foshan, Guangdong Province, China). Before each run, the piggery wastewater was sterilized for 30 min under ozone and the ozone was allowed to dissipate for more than 3 h. The nutrient concentrations of the sterilized piggery wastewater are shown in Table 1.
Table 1 Basic characteristics of piggery wastewater
Parameter |
Values |
COD |
1250 ± 6.57 mg L−1 |
Total nitrogen (TN) |
810.7 ± 8.45 mg L−1 |
Ammonia nitrogen (NH3-N) |
499 ± 3.85 mg L−1 |
Total phosphate (TP) |
202 ± 8.54 mg L−1 |
Phosphate |
61.63 ± 1.13 mg L−1 |
pH |
6.67 |
2.2 Strains and culture conditions
Two algal strains were used in this work. Chlorella pyrenoidosa, a microalga isolated from a wastewater plant in the Runfeng Pig Breeding Co-operative, Guangdong, China, was preserved on Bold's basal medium agar slant at 4 °C. Rhodotorula glutinis, purchased from the Guangdong Microbiology Culture Centre, China, was maintained on a yeast malt (YM) medium agar slant at 4 °C. The cultivation of C. pyrenoidosa and R. glutinis was performed in 250 mL flasks containing 100 mL of Tris-acetate-phosphate (TAP) medium or YM media for 3 d until the algal growth had reached the logarithmic phase. Batch cultures were performed at 28 °C and 120 rpm under 40 μmol photons per m2 per s light intensity for 10 d using modified TAP medium with 1.6 g L−1 yeast extract as nitrogen source instead of sodium nitrate and adding 0.6 g L−1 glucose as carbon source. In co-cultivation experiments, 100 mL of piggery wastewater was added to a 250 mL flask. Chlorella pyrenoidosa and R. glutinis were inoculated into the wastewater with ratios of 0.5
:
1, 1
:
1, 3
:
1, 6
:
1 and 9
:
1, and the cell density of the algae was kept at 0.10–10.00 × 105 cells per mL. The culturing was conducted in a constant temperature shaker at 28 °C, 40 μmol photons per m2 per s light intensity and 150 rpm for 6 d.
2.3 Experimental design
This aim of this experiment, was to exploit microalgae and yeast for piggery wastewater remediation, and was carried out in five steps.
Firstly, the inoculation ratio of microalgae and yeast was optimized to achieve a high biomass yield from the piggery wastewater. In this part, five mixed inoculation ratios of algae and yeast were studied in the piggery wastewater. Secondly, the effect of the contents of the microalgae and yeast co-culture on nutrients in various ratios were measured and calculated to show the effect of co-culture on nutrient removal. Thirdly, the hypotheses to explain nutrients (macromolecule organism as indicators) were tested at the optimum inoculation ratio and mono-culture, respectively, to show the advantage of co-cultivation and then the cooperation mechanisms between microalgae and yeast were validated using scanning electron microscopy (SEM). Fourthly, the optimum inoculation ratio and mono-culture on modified TAP medium were conducted to identify the synergistic relationship by measuring the emission of carbon dioxide (CO2) and the value of oxidation–reduction potential (ORP). Lastly, the co-cultivation of microalgae with yeast at the pilot-scale was conducted in a photoreactor based on the results of the previously mentioned experiments.
2.4 Pilot-scale reactor set-up and operation
The pilot-scale experiment was located in Guangzhou, Guangdong province, China. The 1000 L bioreactor is shown in Fig. 1. The bioreactor consisted of 16 glass columns (2 m long, 10 cm in diameter), a 30 L circulating bucket, and a peristaltic pump for recycling connected to the inlet pipes at the bottom of the reactors. The bioreactor was operated as a sequencing batch reactor with a 5–7 d cycle with the following sequence depending on climate. The solids retention time of 5–6 d were achieved by decanting after settling to a volume of 500 L (removing 50% of the effluent) and approximate 500 L of piggery wastewater was pumped into the reactor. Samples were collected every day from the bioreactor. In this study, co-cultivation in pilot-scale reactor was divided into three phases with two harvests at 7 d and 8 d. The temperature in Guangzhou city in the summer was 20–38 °C when the experiment was conducted. Because the carbon supplement device existed, the pH of the culture was controlled to be between 7 and 7.5.
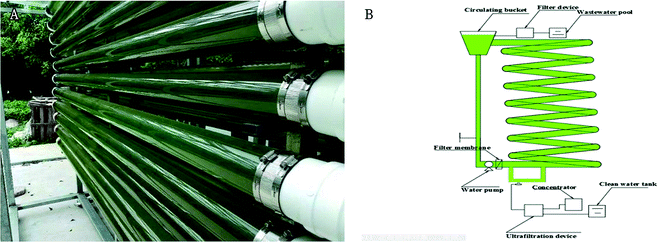 |
| Fig. 1 Structure of a pilot-scale bioreactor for microalgae cultivation [(A) actual diagram (B) concept diagram]. | |
2.5 Parameter analysis
2.5.1 Measurement of the biomass yield. The samples which were collected from the experiments were transferred with a pipette, to a weighed 2 mL centrifugal tube, and the contents were then centrifuged at 12
000 rpm for 5 min, and the supernatant was then discarded. The samples were dried in an oven at 105 °C and then weighed until constant weight was reached. The cell number was measured using hemocytometry. The equation of the dry weight cell concentration (g L−1) was calculated as follows:where W1 and W2 represent the initial and final weights, respectively, and V is the sample volume.
2.5.2 Observation of microstructure. The surface characteristics of the microalgae, yeast and their co-cultivation during culture were observed using a JSM-6330F SEM (Jeol, Japan) at 10
000, 5000, and 10
000× magnification.22 The samples for SEM analysis were collected from the reactor and fixed with 2.5% glutaraldehyde in 0.1 M phosphate buffer at pH 7.2 for 4 h. Subsequently, the samples were dehydrated using a graded series of ethanol solutions: 25%, 50%, 75%, 90% and 100% (three times for each concentration), and then sputter coated with gold.
2.5.3 Protein from the symbiotic culture. After centrifugation of the co-culture medium at 1200 × g for 15 min, the supernatant was removed and washed twice with sterile water. The algae mud obtained after co-culture was measured precisely and slowly mixed with 180 mL of distilled water (1
:
20), followed by the addition of 7.2 g sodium hydroxide with gentle mixing at 47 °C. After ultrasonic processing for 25 min at 47 °C and pH = 7, the mixture was centrifuged at 1200 × g for 15 min. The supernatant was then washed four times with 95% ethanol at 4 °C. The sample solution was then stirred for 1 min and left to stand for 5 h. After centrifugation at 1200 × g for another 15 min, the sediment was lyophilized and stored at 4 °C until use. The sample processed with a single culture of C. pyrenoidosa with the same method was treated as the control protein.
2.5.4 Other parameters. All the nutrients, including COD, NH3-N, TN, and TP, were measured using a HI83200 Multi-parameter Bench Photometer and analysis kits (Hanna Instruments). The ORP of the piggery wastewater was tested using a MultiLine 3630 IDS ORP analyzer (Xylem Analytics Germany GmbH).23 The CO2 produced during culture on modified TAP medium was collected and transferred into a sealed conical flask containing calcium hydroxide. The calcium carbonates (CaCO3) generated with filtration and dying were weighed to calculate the content of CO2. The equation to determine the CO2 content (kg) used is: |
Xdw = (W4 − W3) × (MCO2/MCaCO3)
| (2) |
where W3 and W4 represent the initial and final weight of CaCO3, respectively, MCO2 and MCaCO3 are the molar mass of CO2 and the molar mass of CaCO3, respectively.
2.6 Statistical analysis
The experiments at the pilot-scale were performed once whereas all the test experiments were performed in triplicate. The results are expressed as mean ± standard deviation values. Statistical analysis such as analysis of variance (ANOVA) or the Kruskal test were performed using the R 3.50 or Origin 9.0 software.
3 Results and discussion
3.1 Optimization of the inoculation ratio
As shown in Fig. 2(A), there was a considerable growth of biomass yield in wastewater when microalgae and yeast were inoculated into it. The biomass yield of the microalgae and yeast in piggery wastewater reached a peak value when the inoculation ratio was algae
:
yeast = 3
:
1, achieving 5.35 g L−1 after six days of cultivation, whereas the lowest biomass yield (3.96 g L−1) was observed when the inoculation ratio was 9
:
1. As microalgae and yeast compete for the nutrients in piggery wastewater, an unbalanced ratio of microalgae and yeast will limit the accumulation of biomass.24 The biomass yield percentage of algae increased when the inoculation ratio of algae to yeast increased [Fig. 2(B)]. This indicated that the inoculation ratios of microalgae and yeast directly impacted on the composition of the harvested biomass. As a result, in the harvested biomass, the percentage of microalgal biomass achieved 96.51% when the inoculation ratio was 9
:
1. In a real-world application, the composition of the harvested biomass can be adjusted by modifying the inoculation ratio of microalgae and yeast. Thus, to gain the highest total biomass yield, the inoculation ratio of algae and yeast was set as 3
:
1 for the following experiments. Yen et al.24 discovered that microalgae and yeast competed for nutrients in culture medium, while Gok et al.25 reported a potential cooperation between microalgae and yeast. Consequently, there was a balanced relationship between the microalgae and yeast. As a previous study26 showed, the high pH resulting from utilizing organic acid by microalgae would dramatically prevent the growth of yeast. When the inoculum ratio of the microalgae increased, the pH constantly increased in piggery wastewater, and therefore, inhibited the growth of the yeast dramatically and prevented the microalgae growth to a certain extent. It was reported27 that although microalgae utilize some of the yeast metabolites, such as organic acid, to reduce the inhibition of acid to yeast, this effect is usually maintained for 5 d in co-cultivation. As the pH continued to increase to 7, which was higher than the optimized conditions (pH = 6–7), the growth of yeast began to be prevented.28 As shown in Fig. 2(B), the inoculation of the algae led to a decrease in the biomass yield on the yeast. In contrast, microalgal growth was inhibited by the excessive consumption of nutrients caused by the yeast.
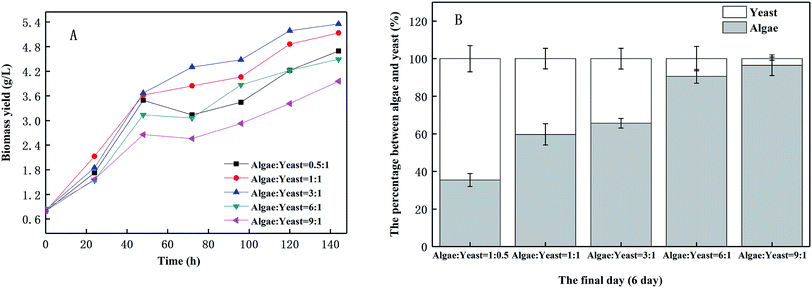 |
| Fig. 2 The change of biomass yield (A) and ultimate proportions between algae and yeast (B) at different inoculation ratios. | |
3.2 Effects of the microalgae and yeast co-culture on nutrients and COD removal
3.2.1 Nutrient removal. The TN and NH3–H was removed efficiently by the microorganisms in the piggery wastewater as shown in Fig. 3(A) and (B). The removal of TN from wastewater cultivated for 6 d with the inoculation ratios of algae
:
yeast from 0.5
:
1 to 9
:
1 was 183.83 mg L−1, 207.07 mg L−1, 287.37 mg L−1, 200.11 mg L−1 and 125.07 mg L−1. The highest removal efficiency of TN was 36.07% when the inoculum ratio was 3
:
1. For various inoculum ratios from 0.5
:
1 to 9
:
1, the removal efficiency of TN was the lowest (15.76%) when the percentage of inoculated algae was the highest. Fig. 3(B) shows that the NH3–H concentration of every inoculum ratio declined during culture, and the NH3–H concentration with the 3
:
1 inoculum ratio declined the most (58.53%). As a major component of TN (about 60%) in piggery wastewater, NH3-N was the direct cause of the removal of TN.29,30 Therefore, as shown in Fig. 3(B), the change of the trend of TN was similar to that of NH3-N. In general, the nitrogen content of the microalgal biomass in wastewater accounted for 3.49–4.98% (34.9–49.8 mg of nitrogen was assimilated to generated 1 g of biomass)31 and the removal of TN from the culture system in this study was 300 mg L−1 of TN, so if the uptake by microbes was complete, that would correspond to production of 6.03–8.59 g L−1 of biomass. However, it was apparent that not all of the nitrogen was removed by the assimilation of microbes because only 5.35 g biomass was generated. But the removal efficiency of TN was just higher than values found in the literature.10,11,32 The removal rate with the 3
:
1 inoculum ratio was more optimized than the other ratios, and exceeded one-third of the total TN in the wastewater. The removal of TP with six days of cultivation in piggery wastewater with various ratios from 0.5
:
1 to 9
:
1 were 43.18 mg L−1, 47.82 mg L−1, 73.71 mg L−1, 43.18 mg L−1, 56.38 mg L−1 and 49.92 mg L−1 [Fig. 3(C)]. The ratios of a higher inoculation percentage of algae were associated with a higher removal efficiency of TP. Phosphorus is closely related to the growth and metabolism of microalgae and is one of the essential elements required to form DNA, RNA, ATP and cell membranes,33 however, it was rarely consumed by algae and not even absorbed by yeast at all when compared to nitrogen. Lack of orthophosphate in the environment induced the production of alkaline phosphatase in algal cells, which allowed the algae to utilize organic phosphorus and inorganic polyphosphorus as phosphorus sources.34 In this study, the removal efficiency of TP using an inoculum ratio of 3
:
1, which gave a TP removal 33.20% in 6 d, was higher than those with other inoculum ratios. Undertaking a comprehensive analysis of the net increase of biomass (5.35 g L−1 with the inoculum ratio of 3
:
1) and the net removal of TP (223.21 mg L−1), it can be concluded that algal utilization was the main cause of the TP removal in this study. In addition, phosphorus removal caused by the formation of insoluble macromolecules could not be assimilated because the phosphate in the water could produce insoluble precipitates.34
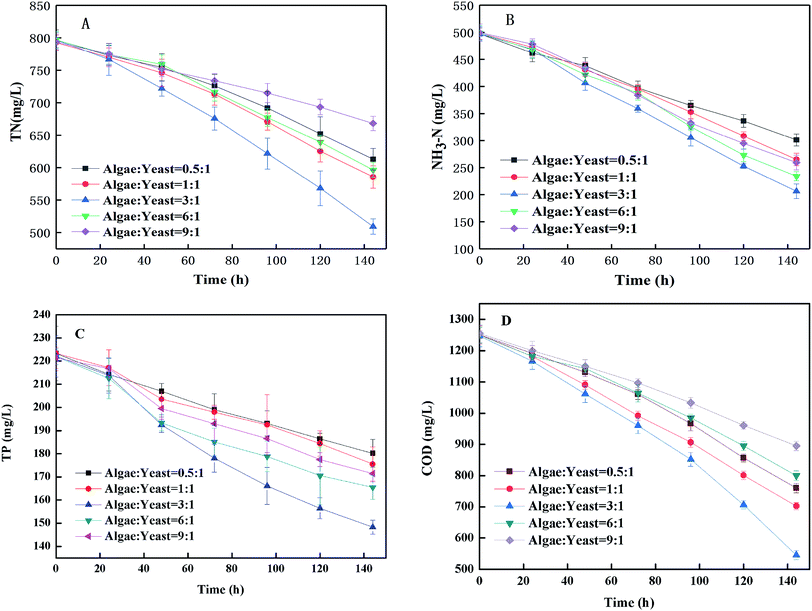 |
| Fig. 3 Effects of microalgae and yeast co-culture on the removal of TN (A), NH3-N (B), TP (C) and COD (D). | |
3.2.2 The COD removal rate on co-cultivation. According to Fig. 3(D), after 6 d of culture, the COD concentration had the maximum removal rate with 56.25% with an inoculum ratio of 3
:
1, which decreased from 1250 mg L−1 to 545 mg L−1, a much higher removal efficiency than that obtained with the other inoculum ratios. The COD of piggery wastewater containing a large amount of macromolecular organic contaminants was consumed by algae and yeast to produce biomass. Algae and yeast could grow collaboratively by utilizing the nutrients in the piggery wastewater. In addition, the result suggested that the suspended organic solids that were difficult to utilize by the mono-algae contributed to the growth of yeast. In the first two days of co-culture, the COD changes did not appear obviously different in any of the inoculum ratios. This result might be because the number of microalgae and yeast was too small to remove the large amount of COD. It was found by Lei Qin et al.31 that the highest biomass yield achieved was 1.26 g L−1 by using a microalgae–yeast culture to treat the liquid digestate of dairy wastewater, which was much lower than the 5.35 g L−1 found in this study. One of the reasons for this might be because the piggery wastewater contains more nutrients, including NH3-N and COD, than the liquid digestate of the dairy wastewater.
3.3 Comparison of the use of mono-culture/co-culture on nutrient removal and surface observation by SEM
As shown in Fig. 4, the COD and TN removal efficiencies with the co-culture of the algae and yeast were larger than those in either of the mono-cultures. The removal of COD and TN in a co-culture cultivated for 6 d were 705.47 and 193.37 mg L−1, respectively. The removal of COD and TN (56.57% and 46.33%, respectively) in the co-culture was dramatically higher than that with the mono-culture of yeast (40.07% and 24.15%, respectively) or algae (27.29% and 16.35%, respectively), on the sixth day, indicating that yeast could promote the growth of algae and that there was a certain synergistic reaction. The removal of COD and TN from the mono-yeast was superior to that from the mono-microalgae. This could be because of the effect of the diameter of the transportation channel on the plasma membrane was small so that large sized, suspended solids could not be directly assimilated by the microalgae.11,35 Therefore, adding yeast into the microalgal culture was necessary. The nutrients in piggery wastewater were removed to gain more biomass. The SEM cell images are shown in Fig. 5 with the mono-yeast (A), the mono-algae (B) and the co-culture (C). The yeast cells are oval whereas the algal cells are spherical. In co-culture, the yeast was closely attached on the surface of the algae. The SEM image of the co-culture and the significant improvements of COD and TN (Fig. 4) in co-culture support the occurrence of a synergistic relationship between the algae and yeast.
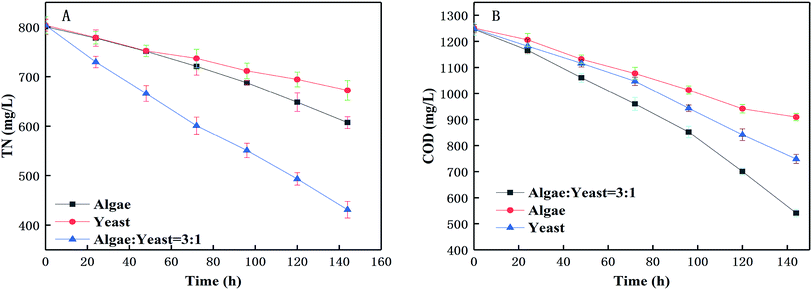 |
| Fig. 4 Effects of mono-microalgae, mono-yeast and optimized co-culture on nutrient removal [(A) TN change and removal; (B) COD change and removal]. | |
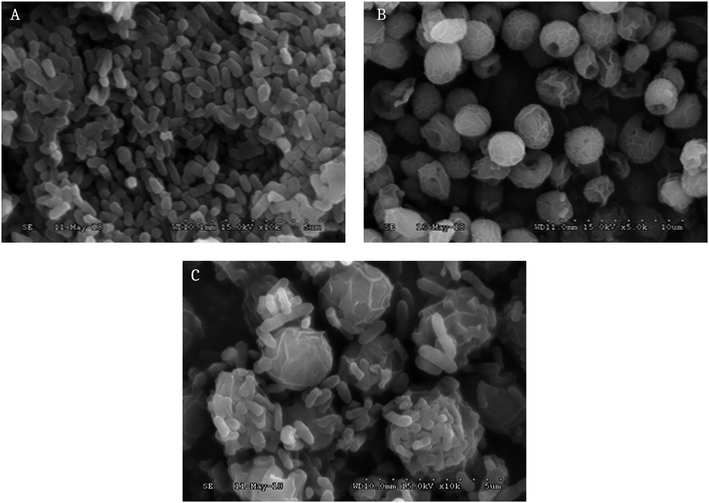 |
| Fig. 5 The SEM images of cells of mono-yeast (A), mono-algae (B) and a co-culture with them both (C). | |
3.4 Revalidation of cooperation mechanism in TAP medium
The purpose of this was to investigate the relationship of the co-cultured algae and yeast in the TAP medium under ideal conditions. Firstly, there was an evident decrease in COD in the three serial experiments (from 1250.00 mg L−1 to 541.66 mg L−1, 909.66 mg L−1, 748.66 mg L−1, respectively) shown in Fig. 6(A). It was supposed that that algae and yeast may not have competed seriously during the wastewater treatment because there were enough nutrients left at the end of the culture period. The CO2 and COD could reveal the relationship between the algae and the yeast. Fig. 6(A) shows that co-the culture group (3
:
1) degraded more COD than other groups after 140 h. The ANOVA at the 95% confidence level showed that COD removals were significantly different (ANOVA; F = 3412, p < 0.05) between the different groups. Fig. 6(B) also showed that the CO2 concentration produced in co-culture group (3
:
1) was significantly lower than that in the yeast group and higher than that in the algae group (ANOVA; p < 0.05).
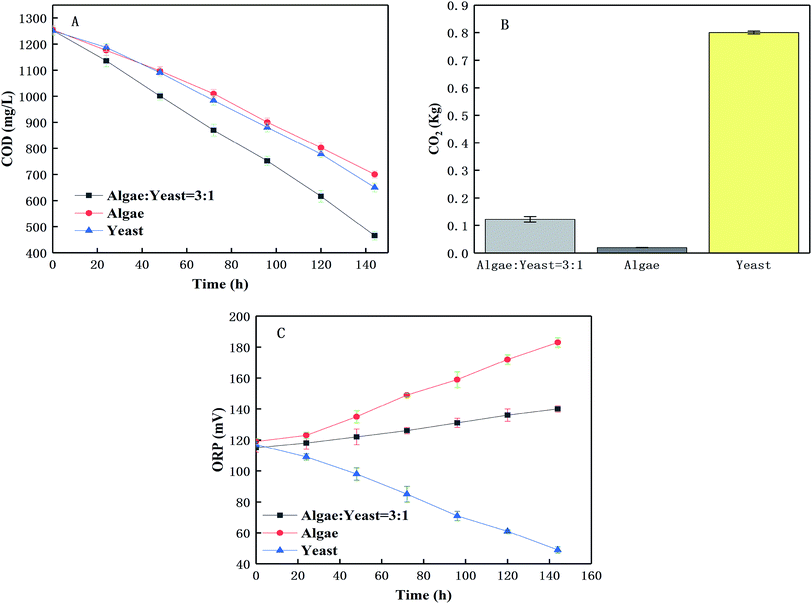 |
| Fig. 6 The change of COD (A) removal and the emission of CO2 (B) and the value of ORP (C) in modified Bold's basal medium. | |
Lots of macromolecular organic pollutants could not be decomposed by algae in the piggery wastewater, which may support the yeast growth. The batch experiment with the highest algae-to-yeast inoculum ratio (9
:
1) had the lowest biomass yield (Fig. 2(A)). When yeast and algae were co-cultured, they utilized the carbon source together to remove organic contaminants,36 and the CO2 and oxygen (O2) were commutatively used to form a favourable symbiotic system, producing a higher biomass yield including value added co-culture products, (e.g. lipid and protein) than the mono culture of each organism.37 The ORPs for different groups were determined and Fig. 6(C) shows that the ORP in the co-culture group (3
:
1) slightly increased from 120 mV to 130 mV during the tests, whereas the ORP of the algae group continuously increased from 120 mV to 180 mV and in the yeast group obviously decreased from 120 mV to less than 60 mV. The ORPs were significantly different between the three groups and changed during the tests (ANOVA; p < 0.05). The ORP is related to O2 production and consumption. The ORP adjustment and substance exchange between microalgae and yeast in a synergistic effect are beneficial to the biomass enhancement. Therefore, these factors existed in a balanced condition with an optimal inoculation ratio between the algae and the yeast. It was revealed that the growth of yeast would reduce the ORP to form an anaerobic environment, which was not conducive to the release of O2 from the growing microalgae to maintain the ORP stability.38
All these results indicated that photosynthetic oxygenation was sufficient to support the heterotrophic growth in the co-culture group (inoculation ratio = 3
:
1), and the enrichment and O2/CO2 exchange balance could be maintained. The higher COD degradation rate in the co-culture group with little CO2 production and slightly increased ORP and energy cycle and nutrition exchange. These results suggested that there were interactions between C. pyrenoidosa and R. glutinis in the co-culture group during the piggery wastewater treatment process.
3.5 Protein in the culture as animal feed additive
Because of their abundant protein, microalgae are usually regarded as animal feed or feed additives.39 In this research, the protein was measured to try and determine whether the co-culture substance could be a good feed or additive. It is indicated from Fig. 7 that for protein of all kinds, the culture ratios obviously increased in the piggery wastewater, whereas it barely changed in the blank group which was used as control. The sudden rise was shown with all the inoculation ratios after 4 d, suggesting that this time might be the optimal time for accumulating protein, which gave the optimal time to harvest the protein and produce feed in the future. All co-culture situations had a more dramatic increase than the mono-algae group, which indicated that the co-culture improved the content of protein in wastewater.40 The protein content with the inoculation ratio of algae–yeast of 3
:
1 with 59.8% (w/w) was outstanding, and was over 0.4 mg mL−1 higher than that obtained with the other inoculation ratios, therefore 3
:
1 was chosen as the optimal inoculation ratio. The result was a higher protein content than found in previous studies,7,41 4% and 20%, respectively, and this might be the reason that the nutrients in piggery wastewater suited microorganism growth. It was reported that N was the important component of protein, which indicated that the increase of protein content also contributed to the total N (NH3-N, nitrate-nitrogen) increase.42,43 In addition, several studies44–46 showed that the carbon/nitrogen (C/N) also had effects on protein content, which suggested that the C/N was necessary to optimize the system to gain more protein as feed.
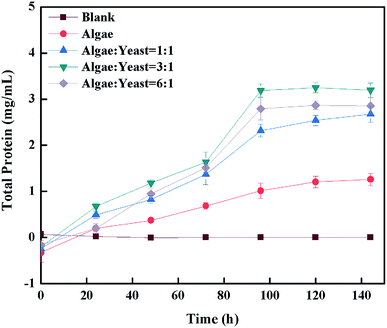 |
| Fig. 7 The change of protein content during cultivation. | |
3.6 Co-cultivation at pilot-scale with wastewater
The pilot-scale co-culture of microalgae and yeast using the optimized inoculation ratio (3
:
1) were conducted in the 1000 L photobioreactor as shown Fig. 1. The changes of biomass yield, and four nutrients (TN, NH3-N, TP, COD) with culture time are shown in Fig. 8. Yeast was firstly inoculated in wastewater to consume the organic acids in the wastewater to prevent the inhibition of the microalgal growth by the low pH value. After the first two days after the yeast inoculation, the biomass yield had apparently increased [Fig. 8(A)]. Then the biomass yield was augmented after adding the microalgae which suggested that using the co-culture between the microalgae and yeast had certain advantages. This can be because the concentration of microorganisms was relatively low after harvest and the exponential growth could be seen after 2–3 d of co-culture. After each harvest, all the nutrient concentrations increased (TN, NH3-N, TP and COD) because the fresh piggery wastewater was added to fill the photoreactor. The trends of the change of all types of nutrients were similar to those obtained in the bench scale experimental results. However, the average daily removal efficiencies of COD, TN and TP appeared to clearly decline, from 7.13%, 6.90%, 3.44% per day at the bench scale to 9.38%, 9.76%, 5.53% per day in pilot scale, respectively. The reason for this might be that the outdoor environment, including the temperature, light intensity and so on, had effected the nutrient.21,47,48 Conversely, the NH3-N showed a higher average daily removal efficiency than in the indoor flask experiments (9.54%, 6.01% per day, respectively). This may be because the outdoor environment was incapable of ensuring an absolutely sterile environment and aerobic bacteria may consume some of the NH3-N. Early studies49,50 also showed the effects of aerobic bacteria on NH3-N. Meanwhile, in this study, it was found that most of the nutrients had great removal efficiencies, such as 82.65% for TN, 53.51% for TP, 93.48% for NH3-N, and 85.44% for COD after 21 d (p < 0.05), which showed a better performance of the removal efficiency of TN than another study.11 The method of adding wastewater step-by-step not only provided sufficient nutrients for cell growth, but also shortened the cultivation period and improved the wastewater treatment capacity as well as the wastewater purification effect. The removal rate of COD, N and P in wastewater by a mixed culture in an outdoor photobioreactor was higher than that obtained in an indoor medium, which provided an effective and large-scale method for the utilization of yeast in wastewater treatment.
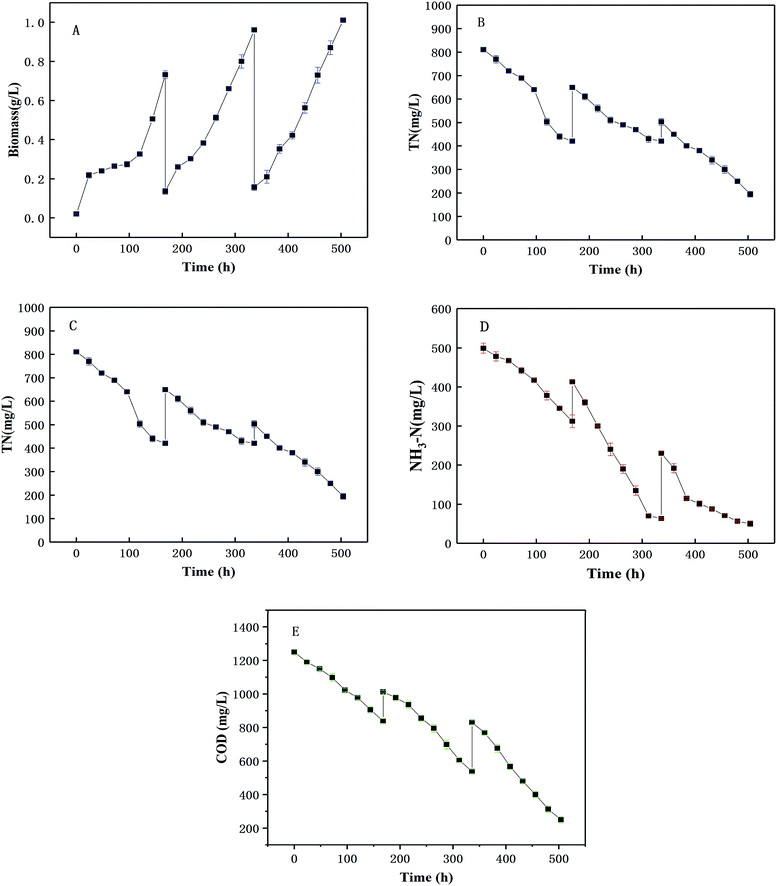 |
| Fig. 8 The co-cultivation of C. pyrenoidosa and R. glutinis in pilot-scale with wastewater (A) biomass production, (B) TN change and removal, (C) NH3-N change and removal, (D) TP change and removal, (E) COD change and removal. | |
3.7 Discussion
In this study, the co-culture between C. pyrenoidosa and R. glutinis with a 3
:
1 algae–yeast inoculation ratio achieved higher nutrient removal rates as well as algal biomass yield than the other experimental groups. It was observed that the CO2 production, biomass, COD degradation rate and ORP exhibited more clear advantages with the inoculation ratio of 3
:
1 than with the other inoculation ratios. This suggested that this inoculation ratio was the condition where the yeast had the most effect on accelerating the algal growth rate. They might have the mutual benefit in utilizing the carbon resource, which was reflected by the change of CO2 and COD levels. Likewise, the variety of ORP indicated that the algae and yeast had a cooperative relationship in the respiratory process.
However, the optimal inoculation ratio showed little difference to results obtained in a previous study,12 whose best condition was an algae
:
yeast ratio of 1
:
1, although they had a similar initial microalgal cell density. It was possible that the piggery wastewater had less organic substances and lower COD than that used in study by Cheirsilp et al.,12 which benefited in more algae because the algae only took up nutrients because of the mutualism. Rhodotorula glutinis basically depended on heterotrophic nutrition, whereas C. pyrenoidosa showed not only photoautotrophic growth, but also heterotrophic growth. The inoculation of yeast was mainly to decompose the macromolecular organics and to provide nutrients for the algae which demonstrated the synergistic relationship.51 Thus, in order to get more nutrients decomposed for the algae, in the study of Cheirsilp et al., more yeast was inoculated than in this study. In addition, the study did not control too many factors in order to reduce the cost of treatment and imitate indoor conditions, which lead to the difference of the optimal inoculation ratio when compared with the of study Cheirsilp et al. The biomass produced in this study could be used as biodiesel feedstock, but could also be used as a feed additive. Thus, there will be no major problem with biomass changes due to different optimal vaccination rates.
Microalgae are now regarded as an important new way to potentially generate bioproducts, such as fish food, animal feed, and fish supplements, because freshwater microalgae have a high production yield and grow easily to obtain the nutrients.52 For example, Chlorella sp. and Spirulina sp. have been used as fish feeds and are currently used for the cultivation of larvae and juvenile shells, and zooplankton.53–55 The microalgal biomass have great potential for the enhancement of the nutrition contents of aquafeeds and feed preparation, because of their chemical composition in terms of carbohydrates, proteins, amino acids, fatty acids, lipids, and other valuable elements.53,54 The application of microalgae in aquaculture, for example as fish feeds, includes larval nutrition for farmed salmonids. The quality of the culture medium, described as a ‘green water technique’, was improved by microalgal biomass, inducing biological activities and the immune systems of aquatic animals.55,56 There are many studies that record that Scenedesmus sp., Chlorella spp., Isochrysis spp., and Spirulina spp. have enough potential to be applied as a protein source for carnivorous and omnivorous fish and prawns at the postlarvae/juvenile stage.54,57–60 Therefore, this method described in this paper, which has a high protein content, has great potential, will be promising for the development of the wastewater treatment and breeding industries.
4 Conclusion
This study evaluated the co-cultivation of C. pyrenoidosa and R. glutinis in piggery wastewater using bench and pilot-scale tests. It was concluded that: (1) co-cultivation with an inoculum ratio of algae to yeast of 3
:
1 was the optimal inoculation ratio for the mono-culture of algae or yeast in batch tests with wastewater, and it achieved higher removal efficiencies of 58.53%, 36.07%, 33.20% and 56.25% for NH3-N, TN, TP and COD, respectively, after 6 d of treatment. (2) The removal of COD, TN and algal biomass proved that the synergistic mechanism between algae and yeast might be achieved by the mutual exchange of CO2 and COD. (3) The 1000 litre pilot scale bioreactor removed 82.65% of TN, 53.51% of TP, 93.48% of NH3-N and 85.44% of COD in 21 d, and the synergistic relationships of the algal–yeast were also shown in the pilot-scale study for both wastewater treatment and biomass production. (4) The protein content increased considerably by 59.8% (m/m) after co-culture of algae and yeast, which laid the foundation for harvesting the microorganisms as a feed additive. This research is a step towards the future improvement of nutrition removal and biomass produce efficiencies as a feed additive of the algal-based technology in wastewater treatment reactors and the livestock breeding industry.
Conflicts of interest
There is no conflict to declare.
Acknowledgements
This research was funded by the Foreign Cooperation Project of the Department of Agriculture and Rural Affairs of Guangdong Province, Special Funds for the Cultivation of Guangdong College Students' Scientific and Technological Innovation (No. pdjh2019a0241), the Guangdong Science and Technology Planning Project (No. 2017A070702021), the Natural Science Foundation of Guangdong Province (No. 2018A03031342), and the Guangzhou Municipal Science and Technology Project (No. 201807010056).
References
- G. U. Xiao-Ming, K. X. Xing, Y. I. Li-Jun, Y. Q. Chen, Q. Y. Liu, X. U. Xiang-Bo, S. B. Gao and M. A. Zhong, J. Ecol. Rural Environ., 2017, 33, 62–69 Search PubMed.
- D. Copetti, K. Finsterle, L. Marziali, F. Stefani, G. Tartari, G. Douglas, K. Reitzel, B. M. Spears, I. J. Winfield and G. Crosa, Water Res., 2016, 97, 162–174 CrossRef CAS.
- S. Tao, Y. H. Cui, F. L. Xu, B. G. Li, J. Cao, W. X. Liu, G. Schmitt, X. J. Wang, W. R. Shen and B. P. Qing, Sci. Total Environ., 2004, 320, 11–24 CrossRef CAS.
- P. Gutwinski and G. Cema, Water Environ. Res., 2016, 88, 63–69 CrossRef CAS PubMed.
- A. A. Rentizelas, A. I. Tolis and I. P. Tatsiopoulos, Waste Manag., 2014, 34, 36–48 CrossRef.
- T. Cai, S. Y. Park and Y. Li, Renewable Sustainable Energy Rev., 2013, 19, 360–369 CrossRef CAS.
- I. Rawat, R. R. Kumar, T. Mutanda and F. Bux, Appl. Energy, 2011, 88, 3411–3424 CrossRef CAS.
- I. Rawat, R. R. Kumar, T. Mutanda and F. Bux, Appl. Energy, 2013, 103, 444–467 CrossRef CAS.
- J. Ling, Y. Tian, A. R. D. Toledo and H. Shim, Energy, 2017, 136, 135–141 CrossRef CAS.
- M. K. Kim, J. W. Park, C. S. Park, S. J. Kim, K. H. Jeune, M. U. Chang and J. Acreman, Bioresour. Technol., 2007, 98, 2220–2228 CrossRef CAS.
- Q. Lu, W. Zhou, M. Min, X. Ma, C. Chandra, Y. T. T. Doan, Y. Ma, H. Zheng, S. Cheng and R. Griffith, Bioresour. Technol., 2015, 198, 189–197 CrossRef CAS.
- B. Cheirsilp, W. Suwannarat and R. Niyomdecha, New Biotechnol., 2011, 28, 362–368 CrossRef CAS.
- W. Yang, X. Li, Z. Li, C. Tong and L. Feng, Bioresour. Technol., 2015, 196, 99–108 CrossRef CAS.
- M. M. Y. Elghandour, L. H. Vallejo, A. Z. M. Salem, M. Z. M. Salem, L. M. Camacho, R. G. Buendia and N. E. Odongo, J. Cleaner Prod., 2017, 168, 1389–1397 CrossRef CAS.
- L. D. F. Roza, F. D. C. Tavernari, D. Surek, C. Sordi, M. L. B. Da Silva, M. M. Boiago and D. Paiano, Valores de energia metabolizável aparente e digestibilidade de aminoácidos de microalga (Spirulina platensis) para frangos de corte[C]//Embrapa Suínos e Aves-Artigo em anais de congresso (ALICE), in Seminário Técnico Científico de Aves e Suínos, 16, Feira Da Indústria Latino-Americana De Aves e Suínos, 2017, Florianópolis, Anais, 2017, AVESUI 2017, Florianópolis, Gessulli Search PubMed.
- S. M. Herber and M. E. Van Elswyk, Poult. Sci., 1996, 75, 1501–1507 CrossRef CAS.
- S. Zhu, L. Qin, P. Feng, C. Shang, Z. Wang and Z. Yuan, Bioresour. Technol., 2018, 274, 313–320 CrossRef.
- L. Luo, X. Lin, F. Zeng, M. Wang, S. Luo, L. Peng and G. Tian, Biochem. Eng. J., 2019, 141, 10–18 CrossRef CAS.
- G. Mujtaba, M. Rizwan, G. Kim and K. Lee, Chem. Eng. J., 2018, 343, 155–162 CrossRef CAS.
- B. Bagheri, Evaluating the effect of environmental parameters on the dynamics of the wine yeast consortium, Stellenbosch University, Stellenbosch, 2018 Search PubMed.
- G. Padmaperuma, R. V. Kapoore, D. J. Gilmour and S. Vaidyanathan, Crit. Rev. Biotechnol., 2018, 38, 690–703 CrossRef CAS.
- J. L. Lin, C. Huang and W. M. Wang, Sep. Purif. Technol., 2015, 151, 262–268 CrossRef CAS.
- X. Li, Y. Li, Y. Li and J. Wu, Appl. Microbiol. Biotechnol., 2018, 102, 10755–10765 CrossRef CAS PubMed.
- H. Yen, P. Chen and L. Chen, Bioresour. Technol., 2015, 184, 148–152 CrossRef CAS.
- C. Gok, D. A. Turkozu and S. Aytas, J. Radioanal. Nucl. Chem., 2011, 287, 533–541 CrossRef CAS.
- B. Cheirsilp, W. Suwannarat and R. Niyomdecha, New Biotechnol., 2011, 28, 362–368 CrossRef CAS.
- E. M. Grima, F. Fernandez, F. G. Camacho and Y. Chisti, J. Biotechnol., 1999, 70, 231–247 CrossRef.
- F. Xue, J. Miao, X. Zhang and T. Tan, Appl. Biochem. Biotechnol., 2010, 160, 498–503 CrossRef CAS.
- S. E. Vlaeminck, A. Terada, B. F. Smets, D. Van der Linden, N. Boon, W. Verstraete and M. Carballa, Environ. Sci. Technol., 2009, 43, 5035–5041 CrossRef CAS.
- S. Huo, J. Chen, X. Chen, F. Wang, L. Xu, F. Zhu, D. Guo and Z. Li, Bioresour. Technol., 2018, 270, 476–481 CrossRef CAS.
- L. Qin, L. Liu, Z. Wang, W. Chen and D. Wei, Bioresour. Technol., 2018, 264, 90–97 CrossRef CAS.
- R. A. I. Aboushanab, M. K. Ji, H. C. Kim, K. J. Paeng and B. H. Jeon, J. Environ. Manage., 2013, 115, 257–264 CrossRef CAS.
- T. Cai, S. Y. Park and Y. Li, Renewable Sustainable Energy Rev., 2013, 19, 360–369 CrossRef CAS.
- C. Ghyoot, N. Gypens, K. J. Flynn and C. Lancelot, J. Plankton Res., 2015, 37, v62 CrossRef.
- W. L. Zemke-White, K. D. Clements and P. J. Harris, J. Exp. Mar. Biol. Ecol., 2000, 245, 57–68 CrossRef CAS.
- Y. Shen, RSC Adv., 2014, 4, 49672–49722 RSC.
- Z. Zhang, H. Ji, G. Gong, X. Zhang and T. Tan, Bioresour. Technol., 2014, 164, 93–99 CrossRef CAS.
- B. K. Das, A. Roy, M. Koschorreck, S. M. Mandal, K. Wendtpotthoff and J. Bhattacharya, Water Res., 2009, 43, 883–894 CrossRef CAS.
- S. S. Roy and R. Pal, Proc. Zool. Soc., 2015, 68, 1–8 CrossRef.
- B. Grote, J. Appl. Phycol., 2016, 28, 3075–3082 CrossRef CAS.
- Q. Lu, W. Zhou, M. Min, X. Ma, Y. Ma, P. Chen, H. Zheng, Y. T. Doan, H. Liu and C. Chen, Bioresour. Technol., 2016, 201, 33–40 CrossRef CAS.
- S. O. Lourenço, E. Barbarino, P. L. Lavín, U. M. Lanfer Marquez and E. Aidar, Eur. J. Phycol., 2004, 39, 17–32 CrossRef.
- D. H. Turpin, J. Phycol., 1991, 27, 14–20 CrossRef CAS.
- N. Korbee, F. L. Figueroa and J. Aguilera, J. Photochem. Photobiol., B, 2005, 80, 71–78 CrossRef CAS.
- T. Rausch, Hydrobiologia, 1981, 78, 237–251 CrossRef CAS.
- H. Li, Z. Liu, Y. Zhang, B. Li, H. Lu, N. Duan, M. Liu, Z. Zhu and B. Si, Bioresour. Technol., 2014, 154, 322–329 CrossRef CAS.
- S. L. Chang, S. A. Lee, S. R. Ko, H. M. Oh and C. Y. Ahn, Water Res., 2015, 68, 680–691 CrossRef.
- M. Nayak, A. Karemore and R. Sen, RSC Adv., 2016, 6, 91111–91120 RSC.
- F. Ahmad, A. U. Khan and A. Yasar, Pak. J. Bot., 2013, 45, 461–465 Search PubMed.
- Z. Yin, L. Tiangang, L. Li, L. Hong and N. F. Y. Tam, Bioresour. Technol., 2011, 102, 2965–2972 CrossRef.
- L. Liu, J. Chen, P. E. Lim and D. Wei, Bioresour. Technol., 2018, 255, 140–148 CrossRef CAS PubMed.
- V. T. Duong, F. Ahmed, S. R. Thomas-Hall, S. Quigley, E. Nowak and P. M. Schenk, Frontiers in Bioengineering and Biotechnology, 2015, 3, 53 CrossRef.
- P. Spolaore, C. Joannis-Cassan, E. Duran and A. Isambert, J. Biosci. Bioeng., 2006, 101, 87–96 CrossRef CAS.
- S. Tongsiri, K. Mang-Amphan and Y. Peerapornpisal, Asian J. Agric. Sci., 2010, 2, 106–110 Search PubMed.
- F. Tulli, G. Chini Zittelli, G. Giorgi, B. M. Poli, E. Tibaldi and M. R. Tredici, J. Aquat. Food Prod. Technol., 2012, 21, 188–197 CrossRef.
- E. Tibaldi, G. C. Zittelli, G. Parisi, M. Bruno, G. Giorgi, F. Tulli, S. Venturini, M. R. Tredici and B. M. Poli, Aquaculture, 2015, 440, 60–68 CrossRef CAS.
- R. F. Attalla and S. K. Mikhail, Egyptian Journal of Aquatic Biology and Fisheries, 2008, 12, 41–60 CrossRef.
- T. M. Badwy, E. M. Ibrahim and M. M. Zeinhom, Partial replacement of fishmeal with dried microalga (Chlorella spp. and Scenedesmus spp.) in Nile tilapia (Oreochromis niloticus) diets, 2008, pp. 801–811 Search PubMed.
- A. J. Vizcaíno, G. López, M. I. Sáez, J. A. Jiménez, A. Barros, L. Hidalgo, J. Camacho-Rodríguez, T. F. Martínez, M. C. Cerón-García and F. J. Alarcón, Aquaculture, 2014, 431, 34–43 CrossRef.
- J. Macias-Sancho, L. H. Poersch, W. Bauer, L. A. Romano, W. Wasielesky and M. B. Tesser, Aquaculture, 2014, 426–427, 120–125 CrossRef.
|
This journal is © The Royal Society of Chemistry 2019 |
Click here to see how this site uses Cookies. View our privacy policy here.