DOI:
10.1039/C9RA04234B
(Paper)
RSC Adv., 2019,
9, 25274-25284
Modification of a polyethersulfone membrane with a block copolymer brush of poly(2-methacryloyloxyethyl phosphorylcholine-co-glycidyl methacrylate) and a branched polypeptide chain of Arg–Glu–Asp–Val
Received
5th June 2019
, Accepted 21st July 2019
First published on 13th August 2019
Abstract
Polyethersulfone (PES) has good thermal stability, superior pH, chlorine tolerance, and excellent chemical resistance; however, the hydrophilicity and biocompatibility of PES need to be improved for its real applications. In this study, we report a surface modification method for the preparation of a functional PES membrane with hydrophilic polymer chains (MPC and GMA) via surface-initiated electrochemically-mediated atom-transfer radical polymerization (SI-eATRP) technology, and the Arg–Glu–Asp–Val polypeptide groups (REDV) were immobilized onto the modified membrane by a ring-opening reaction. XPS and SEM were used to analyze the chemical composition and morphology of the modified membrane surfaces, confirming that the hydrophilic polymer chains MPC and GMA and the polypeptide group REDV were successfully grafted onto the PES membrane surface. The static water contact angle decreased from 89° to 50–65°, and the hydrophilic property of the modified membrane was enhanced. The water flux increased from 4.29 L m−2 h−1 for the pristine PES membrane to 25 L m−2 h−1 for the modified membrane with PGMA chains grafted on it and REDV functional groups immobilized on it; note that the antifouling tests showed that all the modified membranes had the higher flux recovery ratio values (FRR) of above 80% than the pristine PES membrane (about 60%), and the APTT for the modified membrane increased from 46 s to 93 s, indicating that these modified membranes could be applied in the separation and blood purification fields.
1. Introduction
In recent years, membrane technology has become an important topic for high-efficiency, low-cost, and facile separation methods.1 Among the membrane materials, polyethersulfone (PES) is one of the significant polymeric materials, which has been widely used for the preparation of ultrafiltration membranes. Although PES has good thermal stability, superior pH characteristics, chlorine tolerance, and excellent chemical resistance, the hydrophobic nature of PES makes the membrane susceptible to fouling because of the adsorption of nonpolar solutes, hydrophobic particles, and bacteria.2–4 Moreover, when used as a basic material for blood purification, the blood compatibility of PES was not good adequate. The introduction of desired physicochemical characteristics into the membrane surface provides new strategies, such as chemical treatment,5 blending,6–8 surface coating,9 and surface grafting and immobilization,10,11 for the improvement of various surface performances. Among these modification methods, grafting and immobilization are widely used, which involve covalent immobilization of many functional groups onto the membrane surface by surface-initiated polymerization and or simple chemical reactions.1,12,13 For instance, sulfonated hyperbranched polyglycerol (SHPG) polymers with dendritic architectures have been molecularly designed and then grafted on the surface of polydopamine (PDA)-coated polyethersulfone (PES) hollow fiber membranes. Compared to the pristine PES membranes, the SHPG-modified PRO membranes show significantly higher resistance to protein adhesion and bacterial attachment due to high wettability of their ionic polymer brushes.14
Because of the level of control and high degree of living character in the synthesized polymer, controlled radical polymerization is suitable for the design of macromolecular structures such as block copolymers, star polymers, and other types of polymers.15–17 Controlled radical polymerization has three main types: nitroxide-mediated polymerization, atom-transfer radical polymerization, and reversible addition fragmentation chain transfer.18–25 Surface-initiated eATRP (SI-eATRP) is an effective controlled radical polymerization method, which allows the preparation of well-defined polymer brushes on various types of substrates.26–29 The concept behind eATRP is that the ratio of catalyst activator to deactivator is precisely controlled by an electrochemical redox process on the electrode surface, thereby rendering the eATRP process operational via an external stimulus.30–32 In the eATRP systems, Cu(II)/L as the initial catalyzer replaces Cu(I)/L in the ATRP system. At sufficient potential in a three-electrode system, the reduction of Cu(II)/L to Cu(I)/L occurs at the working electrode. The polymerization rate and degree can be controlled because of the high (X–Cu(II)/L)/(Cu(I)/L) ratio and low [R]. In the present study, we grafted a homopolymer on the PES membrane by SI-eATRP and studied the effects of reaction conditions including monomer concentration, applied potential, and reaction time. We also combined the SI-eARTP method with a further grafting step to modify the surface properties and performance of the PES membrane with functional polymer brushes.33–35
Zwitterionic-based materials and peptide have been considered as the most suitable alternatives for the preparation of ultralow fouling surfaces and have high resistance to bacterial adhesion and biofilm formation.36–39 In this study, well-defined poly(glycidyl methacrylate) (P(GMA)) brushes and poly 2-(methacryloyloxy)ethyl-2-2(trimethylamine)ethyl phosphate were subsequently grafted via SI-eATRP polymerization. Consequently, the filtration property was significantly enhanced. After this, we grafted Arg–Glu–Asp–Val (REDV) peptides onto the membrane surface via a reaction between REDV and PGMA to improve the biocompatibility of the PES membrane. The chemical composition of the modified PES membrane was characterized by X-ray photoelectron spectroscopy and scanning electron microscopy, and the water contact angle, ultrafiltration property of the membrane and activated partial thromboplastin times (APTTs) were also investigated.
2. Experimental
2.1 Materials
Polyethersulfone (PES, Ultrason E6020P, Mn = 58
000 Da) and glycidyl methacrylate (GMA) were obtained from BASF, Germany. 2-Methacryloyloxyethyl phosphorylcholine (MPC, 98%) was purchased from NanJing LeTianRan Institute of Technology. Arg–Glu–Asp–Val (REDV, 99%) was purchased from KeTai Biology Technology Company. Dibromo butyryl bromine (C4H6Br2O, 98%), and tetramethylethylenediamine (TMEDA) were obtained from Aladdin Reagent. Cupric bromide (CuBr2, 98%) and stannous chloride (SnCl2, 98%) were purchased from Shanghai Zhongtai Chemical Reagent Company. Ethyl alcohol, hydrochloric acid (HCl, 37%), nitric acid (HNO3, 65%) and sulfuric acid (H2SO4, 98%) were purchased from Changliao Chemical Reagent Company. Triethylamine (TEA) was purchased from DaMao Chemical Reagent Factory. Ethanol and anhydrous diethyl ether were purchased from KeLong Chemical Reagent Factory. N-Vinylpyrrolidone (N-VP, 99%) was purchased from Alfa Aesarand.
2.2 Synthesis of PES-NH2
(i) HNO3 (30 ml, 0.66 mol) and H2SO4 (40 ml, 0.66 mol) were mixed in a 250 ml round-bottom flask at room temperature, and then, PES (10 g, 0.167 mmol) was added slowly. After being continuously stirred for 6 h at 65 °C, the resulting mixture was washed and filtered three times with deionized water. Granular PES-NO2 was obtained after vacuum drying for 24 h. (ii) SnCl2 (20 g, 0.105 mol) was dissolved in HCl (20 g, 37%, 0.203 mol) in a flask, followed by the addition of 50 ml ethyl alcohol.40 Then, 3 g ground PES-NO2 was added followed by stirring for 6 h. Finally, the mixture was filtered and vacuum dried for 24 h to obtain PES-NH2.
2.3 Preparation of the PES-Br membrane
(i) PES (1.6 g, 0.027 mmol) and PES-NH2 (0.2 g, 0.003 mmol) were dissolved in DMAC (8.2 ml, 0.088 mol) followed by stirring for 12 h to obtain a homogeneous solution. The resultant uniform solution was degassed completely under vacuum for 20 min. After this, the casting solution was spread on a glass surface, followed by spin-coating to achieve homogeneous thickness using a swell gluing film machine and immersion in deionized water at room temperature. The membranes were obtained by a liquid–liquid phase separation method and immersed continually in deionized water for 24 h to remove residual DMAC. (ii) After this, 50 mg of the PES-NH2 membrane was rinsed in a beaker containing 54 μl TEA (0.389 mmol) and 30 ml diethyl ether (0.289 mol) in an ice-water bath, and another solution with 0.39 mol BIBB and 20 ml diethyl ether (0.192 mol) was added at the rate of one drop per second. The reaction was continued for 0.5 h in an ice-water bath after titration. The resultant solution was stirred at room temperature for 12 h and subsequently washed with diethyl ether, methylbenzene, methyl alcohol, and deionized water. The PES-Br membrane was obtained after drying under vacuum for 12 h.33,41
2.4 Grafting of MPC and GMA on the PES-Br membrane by SI-eATRP
The SI-eATRP reaction was carried out in a three-electrode system comprising two platinum gauzes as the working electrode and counter electrode and a saturated calomel electrode as the reference electrode. Cyclic voltammograms (CV) were obtained at the scan rate of 0.05 V s−1 using 45 ml of H2O/methanol solution (2
:
1 v/v) containing MPC and GMA as monomers at different ratios (detailed amounts are listed in Table 1), ligand TMEDA (0.06 g, 0.516 mmol), catalyst CuBr2 (0.09 g, 0.403 mmol), and the mixed solvent H2O/MeOH (30 ml/15 ml). The membrane was fixed on the platinum sheet of the working electrode. The three electrodes were immersed in the electrolyte, and the reaction was performed by setting the applied potential and polymerization time. After reaction, the membrane was soaked in ethanol for 24 h to remove the absorbed solvents and then dried at 40 °C for 24 h.42 During polymerization, the GMA and MPC monomer ratio was used to mediate the composition of the grafted polymer chains.
Table 1 The ratio of the monomer to electrolyte concentration
Membrane ID |
GMA (mol) |
MPC (mol) |
REDV (mg ml−1 in PBS solution) |
M-1-1 |
0.005 |
0.035 |
— |
M-1-2 |
0.005 |
0.035 |
0.2 |
M-2-1 |
0.02 |
0.02 |
— |
M-2-2 |
0.02 |
0.02 |
0.2 |
M-3-1 |
0.035 |
0.005 |
— |
M-3-2 |
0.035 |
0.005 |
0.2 |
2.5 Immobilization of REDV on the modified PES membrane
The immobilization of REDV was performed as follows: REDV was dissolved in a phosphate buffer solution (PBS, pH was in the range of 7.2–7.4) to obtain a 0.2 mg ml−1 solution. Then, the copolymer-grafted PES membrane was immersed in the solution, which was stirred at 4 °C for 24 h. After this, the membrane was washed 3 times with distilled water and dried in vacuum for 24 h.43
2.6 Characterization of the membranes
The chemical composition of the membrane surfaces was characterized by X-ray photoelectron spectroscopy (XPS) (XSAM800, KRATOS Co., Britain) and scanning electron microscopy (SEM) (JSM-6701F, JEOL, Japan). Surface hydrophilicity of the membrane was evaluated using static water contact angles (WCA, PHS-3C, Precision Science Co., Shanghai, China) determined by the sessile drop method, with 5 μl of water per drop. The final data listed in the study are the mean values of three different site measurements for every sample.
2.7 Filtration and anti-fouling experiments
Membrane filtration and anti-fouling performance evaluation was carried out using a dead-end filtration device (HL-2, Huxi Co., China) with the effective area of 1 cm2 (A) loaded into the membrane cell for each filtration test. The membrane was pre-compacted at 0.15 MPa by deionized water for 0.5 h. Then, deionized water was pushed through the membrane under 0.1 MPa, and the water flux at the end of 1 h was called the initial pure water flux (JW1) and calculated by eqn (1).44 |
 | (1) |
where Vw (L) is the volume of the permeated water; S (m2) is the effective membrane area; and t (h) is the operation time.
After 1 h, the filter was fed with the BSA solution, which was used as a model protein solution to evaluate the protein-resistance characteristics. BSA was dissolved in the phosphate-buffered saline solution (PBS, pH 7.2–7.4) at the concentration of 1.0 mg ml−1, and the BSA solution flux (Jp) was calculated by eqn (2).
|
 | (2) |
where
Vp (L) is the volume of the permeated BSA solution;
S (m
2) is the effective membrane area; and
t (h) is the operation time.
After another 1 h, the sample was repeatedly fed with deionized water. After cycling for 5 h, the membrane sample was completely washed with PBS solution and shaken slowly in deionized water for 24 h. Finally, the water flux (Jw2) was measured again by eqn (2). The flux recovery ratio (FRR) and total fouling ratio (Rt) were calculated by eqn (3) and (4), respectively:45
|
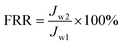 | (3) |
|
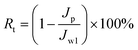 | (4) |
To further evaluate the anti-fouling properties of the membrane, reversible fouling ratio (Rr) and irreversible fouling ratio (Rir) were calculated by eqn (5) and (6), respectively.46
|
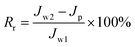 | (5) |
|
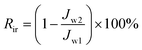 | (6) |
Moreover, the BSA rejection ratio (R) was calculated by eqn (7).
|
R = (1 − Cp/Cb) × 100%
| (7) |
where
Cp and
Cb (mg ml
−1) are the BSA concentrations of the permeated and bulk solutions, respectively, which have been measured by a UV-Vis spectrophotometer at the wavelength of 280 nm.
2.8. Clotting time
To evaluate the antithrombogenicity of the modified membranes, the activated APTT was measured by the automated blood coagulation analyzer CA-50 (Sysmex Corporation, Kobe, Japan), and the test method was performed as follows: at the beginning of the APTT test, healthy human fresh blood (male, Chinese) was obtained in vacuum tubes containing sodium citrate as an anticoagulant (anticoagulant to blood ratio, 1
:
9 v/v), and the platelet-poor plasma (PPP) was obtained after centrifugation at 4000 rpm for 15 min. Moreover, the membrane (0.5 cm, three pieces) was immersed in a 0.2 ml PBS solution (pH = 7.4) for 1 h. The PBS solution was removed, and then, 0.1 ml of fresh PPP was introduced. After incubation at 37 °C for 30 min, 50 ml of the incubated PPP was added to a test cup, followed by the addition of 50 ml APTT agent (Dade Actin Activated Cephaloplastin Reagent obtained from SIEMENS) (incubated 10 min before use) and incubation at 37 °C for 3 min. After this, 50 ml of 0.025 M CaCl2 solution was added, and then, the APTT was measured. At least three measurement values were averaged to obtain a reliable value, and the results were analyzed by the statistical method.47
Healthy human fresh blood (from three 24 year-old male donors) was obtained using vacuum tubes (5 mL, Jiangsu Kangjian Inc., China) containing sodium citrate or ethylenediaminetetraacetic acid as an anticoagulant (anticoagulant-to-blood ratio, 1
:
9 (v/v)). For plasma collection, the blood was centrifuged at 1000 rpm for 15 minutes to obtain platelet-rich plasma or at 4000 rpm for 15 minutes to obtain platelet-poor plasma. The same blood samples were used for all blood tests. The experiments were approved and performed by West China Hospital, Sichuan University, and all experiments were performed in compliance with the relevant laws and national guidelines (GB/T 16886.4-2003/ISO 10993-4:2002, General Administration of Quality Supervision, Inspection and Quarantine of the People's Republic of China, Standardization Administration of the People's Republic of China). Informed consent was obtained for any experimentation with human subjects, and all regulations (e.g. IRB) were fulfilled for using human blood.
3. Results and discussion
3.1 Surface modification and characterization of the membrane
The functional copolymer brushes were introduced by the SI-eATRP method, which was conducted in an electrochemically mediated system of three-electrodes. As shown in Fig. 1a, the reaction equipment included two platinum gauzes used as the counter electrode (CE) and working electrode (WE) and a saturated electrode as the reference electrode. Prior to surface-initiated polymerization, a uniform monolayer of the -Br initiator was immobilized on the surface of the PES membrane, which was synthesized through three steps: the syntheses of aminated PES (PES-NH2), the blending membrane PES/PES-NH2 (M–NH2), and the initiator-immobilized membrane (M–Br), as shown in the experimental section. Note that the blending of PES and PES-NH2 into the membrane would form an –NH2-rich PES membrane surface because during the liquid–liquid phase-separation process, hydrophilic PES macromolecules containing-NH2 would migrate to the surface of the membrane (M–NH2) but cannot elute from the membrane due to the amphiphilic nature of PES-NH2 and fast solvent exchange.
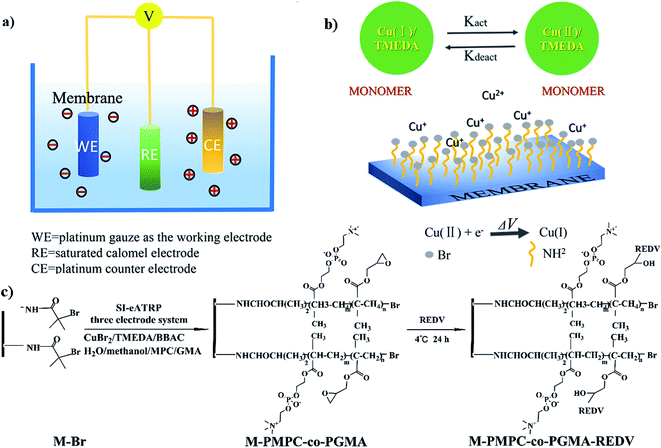 |
| Fig. 1 (a and b) Schematic of the strategy of grafting functional copolymers on the membrane by SI-eATRP and (c) synthesis of M-PMPC-co-PGMA-REDV. | |
The –Br group-immobilized membrane (M–Br) would further provide active sites for surface-initiated polymerization and copolymerization; in the rational potential window, the redox reaction would occur, and surface-initiated polymerization at the interface of the WE electrode/electrolyte would be triggered at a catholic current generated by the Cu(I)/TMEDA complex obtained from Cu(II)/TMEDA, as shown in Fig. 1b. Via the SI-eATRP method, the monomers MPC and GMA were grafted on the membrane surface by sequentially feeding the monomers in the order of MPC and GMA. The obtained membrane was named as M-PMPC-co-PGMA. The reactivity of the epoxide groups in the grafted P(GMA) brushes on the modified membrane led to the further immobilization of REDV peptides, and the final surface-functionalized membrane was named as M-PMPC-co-PGMA-REDV. The reaction conditions and macromolecular structure are presented in Fig. 1c.
For constructing different compositions of the polymer brush, we changed the ratio of the concentrations of these two monomers during the experiment process. When the mol ratios of GMA to MPC were 0.005/0.035, 0.02/0.02, and 0.035/0.005 (mol/mol), the prepared membranes were labeled as M-1-1, M-2-1, and M-3-1, respectively. Furthermore, the M-1-1, M-2-1, and M-3-1 membranes grafted with REDV were named M-1-2, M-2-2, and M-3-3, respectively. The synthesis conditions of these membranes are presented in Table 1 (Fig. 2).
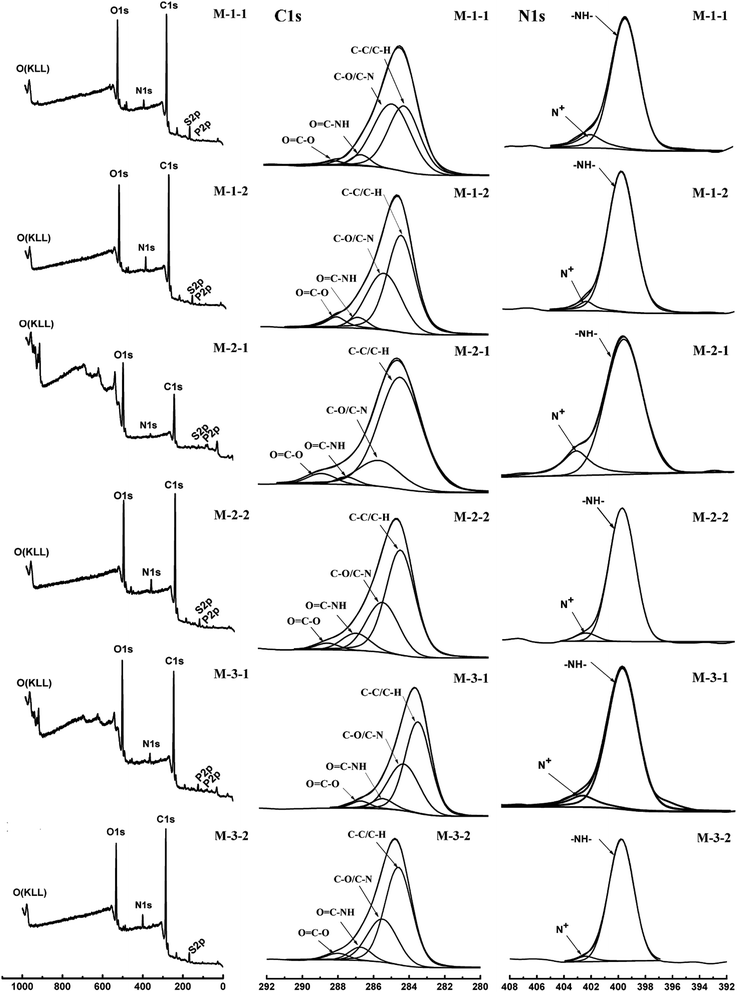 |
| Fig. 2 XPS spectra of the modified PES membranes. | |
The chemical compositions of the PES membrane surfaces at various stages of surface modification were determined by X-ray photoelectron spectroscopy (XPS), which are shown in Fig. 3 and Table 2. The C ls core-level spectra can be curve-fitted into four peak components with binding energies at about 284.6, 285.7, 286.8, and 288.1 eV, attributed to C–H and C–C, C–O and C–N, and O
C–NH and O–C
O, respectively. For M-1-1, the peak at 285.7 eV in the C 1s curve and the peak at 402.5 eV in the N 1s curve were observed, indicating that PGMA and PMPC were grafted successfully. Compared with that of M-1-1, the intensity of the N 1s signal peak of M-1-2 was significantly enhanced due to the immobilization of the REDV peptide; moreover, the decrease in the peak area of the N+ signal confirmed the change in the chemical composition due to the immobilization of REDV. Note that the monomer ratio in the copolymer brush could be mediated via SI-eATRP, and the REDV molecular brush could also be grafted successfully on the surface of all the modified PES membranes.
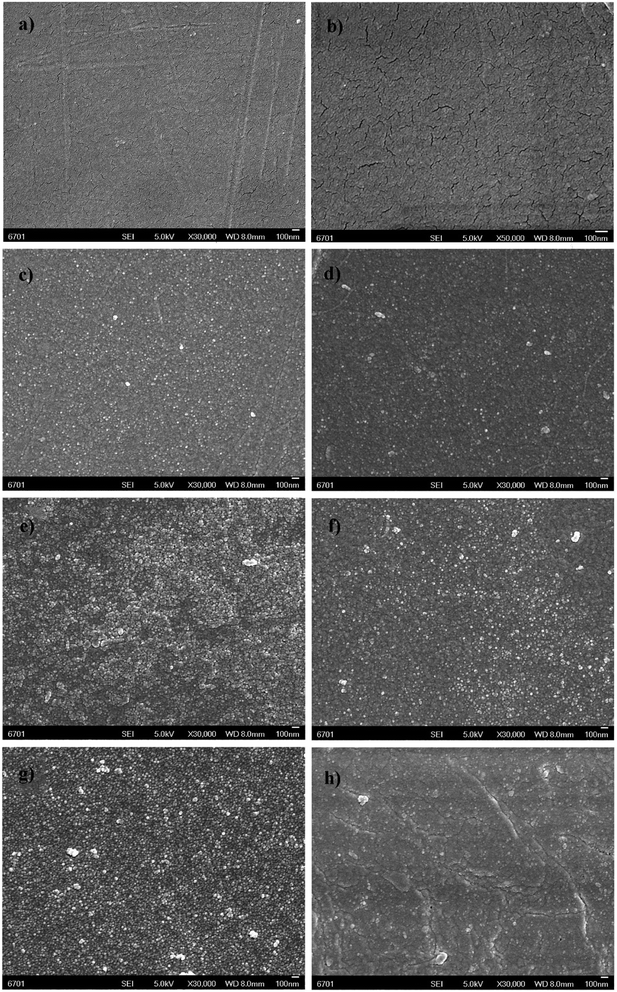 |
| Fig. 3 SEM images of the surface morphologies for (a) and (b) M-PES, (c) M-1-1, (d) M-1-2, (e) M-2-1, (f) M-2-2, (g) M-3-1, and (h) M-3-2. | |
Table 2 Surface elemental compositions of the modified membranes determined by XPS
Membrane ID |
C (at%) |
N (at%) |
O (at%) |
S (at%) |
P (at%) |
M-1-1 |
71.02 |
2.74 |
22.57 |
3.33 |
0.34 |
M-1-2 |
72.11 |
4.79 |
20.7 |
2.15 |
0.26 |
M-2-1 |
59.78 |
2.37 |
34.84 |
1.18 |
1.84 |
M-2-2 |
70.72 |
4.59 |
22.51 |
1.62 |
0.56 |
M-3-1 |
70.19 |
3.68 |
24.35 |
1.55 |
0.23 |
M-3-2 |
72.23 |
4.77 |
20.77 |
2.03 |
0.19 |
Changes in the surface morphologies of the modified membranes were characterized by SEM, as presented in Fig. 4. From the figure, it was observed that the pristine PES membrane possessed a smooth and flat surface (Fig. 4a and b), whereas different surfaces were observed for the membranes modified with both GMA/MPC and REDV peptides. Compared with that of M-PES, the surface of M-1-1 (Fig. 4c) shows nanoparticles with the size of about 20 nm, which are equally distributed because of the shrunken grafted polymer brush. Upon further grafting of the REDV peptide coating, the M-1-2 surface became relatively flat (Fig. 4d). With an increase in the GMA/MPC ratio in the electrolyte, denser layers containing many more nanoparticles were present on the surface of both M-2-1 and M-3-1 (Fig. 4e and g). Likewise, the M-2-2 (Fig. 4f) and M-3-2 (Fig. 4g) surfaces became flatter because of the immobilization of the REDV peptides. The SEM characterization reveals that GMA is a more reactive monomer than MPC, and thus, GMA can be easily grafted via SI-eATRP.
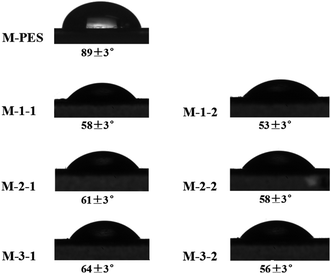 |
| Fig. 4 Water contact angles for M-PES and the modified membranes. | |
3.2 Hydrophilicity of the membranes
Water contact angle (WCA) is always used to evaluate the hydrophilicity and wettability of material surfaces. As observed from Fig. 5, the WCA of pristine M-PES was 89°, whereas after the membrane was grafted with PGMA or further immobilized with the REDV peptides, the WCA of the modified membranes declined to between 50 and 65°. These results showed that the hydrophilicity of the modified membrane surfaces was successfully improved. Moreover, the WCA values gradually increased in the order M-1-1 < M-2-1 < M-3-1 due to the hydrophobic character of GMA and increase in the GMA/MPC ratio in the electrolyte. Compared with the cases of M-1-1, M-2-1, and M-3-1, REDV was immobilized on the surfaces of M-1-2, M-2-2, and M-3-2; this led to a decreases in the CA to 53, 58, and 56° because the hydrophilicity of the M-1-2, M-2-2, and M-3-2 surfaces was increased due to the abundance of amidogen and hydroxyl groups in the REDV chains.
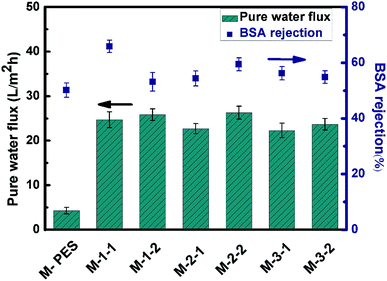 |
| Fig. 5 Pure water flux and BSA rejection of the membranes. | |
3.3 Permeation and anti-fouling properties
The results of water flux and BSA rejection are presented in Fig. 6. After the grafting of PGMA chains and immobilization of the REDV functional groups, the water flux increased from 4.29 L m−2 h−1 for the pristine PES membrane to 25 L m−2 h−1 for the modified membrane. This was because the hydrophilic property of the molecular brush on the membrane surface improved the infiltration of water and decreased the resistance to water. Compared with the unmodified REDV membrane, mainly ascribed to the slightly increased caused by REDV hydroxyl and amidogen replaced PGMA ester, improved the hydrophilic of the immobilization REDV membrane.
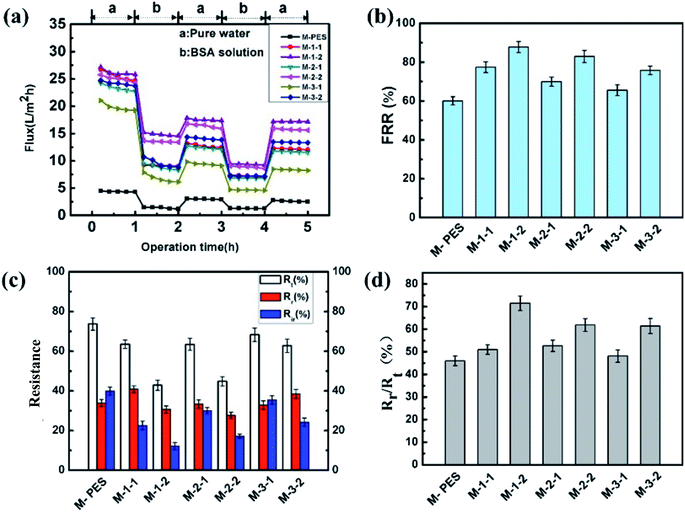 |
| Fig. 6 Anti-fouling properties of the membranes: (a) time-dependent fluxes, (b) water flux recovery ratios (FRR), (c) resistances, and (d) ratio of irreversible fouling (Rr) to total fouling (Rt). | |
The anti-fouling properties of the membranes were investigated by the filtration of pure water and BSA solution, as shown in Fig. 7. Fig. 7a shows the time-dependent flux curves of the two cycles conducted using water and BSA. During the filtration process, all membranes exhibited similar performances: the flux decreased dramatically when the feed solution changed from water to the BSA solution due to the deposition and absorption of BSA molecules on the surface or pore surface of membranes; this resulted in the blocking of pores and fouling of the membrane. When adsorption and diffusion reached equilibrium, a relatively stable flux level was obtained at the end stage of the BSA solution ultrafiltration. Moreover, the fluxes of the modified membrane were higher than that of the pristine PES membrane during the entire filtration process because of the increased hydrophilicity of the surface layer on membranes and the large amount of macropores present in the finger-like pores. As shown in Fig. 7b, the flux recovery ratios (FRR) were evaluated. It was observed that all the modified membranes (above 80%) had higher FRR values than the pristine PES membrane (about 60%). Note that the modified membrane M-1-2 had a less flux value decrease (about 88%) than the other modified membranes; this might be due to the surface roughness and surface charge of the fabricated membranes. In addition, the FRR of M-1-2 exceeded those of the M-2-2 and M-3-2 membranes; this might be because the modification of zwitterion offered greater resistance to protein absorption than the case of REDV peptides.
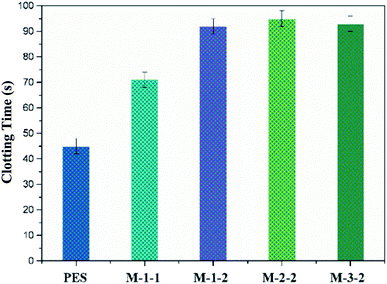 |
| Fig. 7 APTTs for the membranes M-PES, M-1-1, M-1-2, M-2-2, and M-3-2 (n = 3). | |
Membrane total fouling (Rt) is usually composed of reversible fouling (Rr) and irreversible fouling (Rir). Reversible fouling is mostly caused by protein-cake deposition on a membrane surface, which can be easily removed by hydrophilic washing. However, irreversible fouling is mainly induced by absorbed or attached proteins on the surfaces and pores, which is difficult to remove by physical cleaning. The results of Rt, Rr, and Rir of the pristine and modified membranes are presented in Fig. 7c. In contrast, the total fouling (Rt) of the modified membranes remained unchanged. Fig. 7d shows the proportion of Rr/Rt, which exhibits that the modified membrane has higher Rr/Rt value than the pristine PES membrane. This is attributed to the grafting of the hydrophilic brush on the membrane surface, which can increase the proportion of Rr/Rt and can be beneficial for increasing the membrane-recycling performance. However, as the MPC content decreases and the peptide content increases, Rr/Rt decreases. These results are mainly due to the presence of anionic phosphate groups and quaternary ammonium cationic groups in MPC, which easily form a robust hydration layer in aqueous media; the hydration layer can provide a strong steric effect for protein and thus restrain protein absorption; this confirms that the zwitterion has a more significant modification effect.
Grafting of the REDV biomacromolecular chains on the membrane surface enhances membrane biocompatibility with blood. The modified membranes obtained in this study can also be used as key materials in the blood purification field, where blood compatibility is critical. The APTT experiment is a main method to evaluate blood compatibility, and the blood clotting time of the prepared membrane is shown in Fig. 7. The APTTs for M-PES, M-1-1, M-1-2, M-2-2, and M-3-2 are 45, 71, 92, 95, and 93 s, respectively. It can be found that the APTTs of the modified membranes M-1-2, M-2-2, and M-3-2 increases significantly than those of M-1-1 and M-1-2 due to the immobilization of the REDV groups on the surface of membranes.
4. Conclusions
Herein, surface-initiated electrochemically mediated atom-transfer radical polymerization (SI-eATRP) of the functional monomers GMA and GMA was successfully carried out by introducing the –Br surface initiator on the PES membrane surface; moreover, we grafted the activated REDV peptide by performing a ring-opening reaction between GMA and REDV. The SEM and XPS results indicated that the compositions of the functional polymer brushes grafted on the membrane surface could be controlled by varying the concentration ratio of the two types of monomers. During this process, we found that GMA was more reactive than MPC during SI-eATRP. The PES membrane surfaces modified by these functional copolymer chains exhibited good anti-fouling properties, as demonstrated by the filtration of pure water and BSA solution; by controlling the proportions of zwitterionic MPC and REDV, it was demonstrated that the zwitterion brushes had higher influence on the anti-fouling properties of the modified membrane surfaces as compared to the peptide brushes. In conclusion, with the inherent advantages, i.e. the good hydrophilicity and anti-fouling properties, of the SI-eATRP method, the PES membranes modified with phospholipid and polypeptide groups showed superior performance and application in the separation and biology fields. In the future, other types of polypeptide functional groups would be grafted by more eATRP-related methods for constructing biomacromolecule-based membrane surfaces.
Conflicts of interest
There are no conflicts to declare.
Acknowledgements
This work was partly supported by the National Natural Science Foundation of China (51463012 and 51763014), the Program for Hongliu Distinguished Young Scholars at Lanzhou University of Technology, and a Joint fund between Shenyang National Laboratory for Materials Science and the State Key Laboratory of Advanced Processing and Recycling of Nonferrous Metals (18LHPY002).
References
- C. Zhao, J. Xue, F. Ran and S. Sun, Modification of polyethersulfone membranes-A review of methods, Prog. Mater. Sci., 2013, 58, 76–150 CrossRef CAS.
- H. Shi, Y. He, Y. Pan, H. Di, G. Zeng, L. Zhang and C. Zhang, A modified mussel-inspired method to fabricate TiO2, decorated superhydrophilic PVDF membrane for oil/water separation, J. Membr. Sci., 2016, 506, 60–70 CrossRef CAS.
- F. Ran, S. Nie, W. Zhao, J. Li, B. Su, S. Sun and C. Zhao, Biocompatibility of modified polyethersulfone membranes by blending an amphiphilic triblock co-polymer of poly(vinyl pyrrolidone)-b-poly(methyl methacrylate)-b-poly(vinyl pyrrolidone), Acta Biomater., 2011, 7(9), 3370–3381 CrossRef CAS PubMed.
- L. Zhu, L. Zhu, J. Jiang, Z. Yi, Y. Zhao, B. Zhu and Y. Xu, Hydrophilic and anti-fouling polyethersulfone ultrafiltration membranes with poly (2-hydroxyethyl methacrylate) grafted silica nanoparticles as additive, J. Membr. Sci., 2014, 451(4), 157–168 CrossRef CAS.
- Q. Shi, Y. Su, S. Zhu, C. Li, Y. Zhao and Z. Jiang, A facile method for synthesis of pegylated polyethersulfone and its application in fabrication of antifouling ultrafiltration membrane, J. Membr. Sci., 2007, 303(1), 204–212 CrossRef CAS.
- B. Fang, Q. Ling, W. Zhao, Y. Ma, P. Bai, W. Qiang, H. Li and C. Zhao, Modification of polyethersulfone membrane by grafting bovine serum albumin on the surface of polyethersulfone/poly(acrylonitrile-co-acrylic acid) blended membrane, J. Membr. Sci., 2009, 329(1–2), 46–55 CrossRef CAS.
- F. Ran, H. Song, J. Wu, M. Lang, X. Niu, H. Fan, L. Kang and C. Zhao, Bionic design for anticoagulant surface via synthesized biological macromolecules with heparin-like chains, RSC Adv., 2015, 5(71), 58032–58040 RSC.
- Y. Zhao, P. Zhang, J. Sun, C. Liu, L. Zhu and Y. Xu, Electrolyte-responsive polyethersulfone membranes with zwitterionic polyethersulfone-based copolymers as additive, J. Membr. Sci., 2016, 510, 306–313 CrossRef CAS.
- T. He, M. H. V. Mulder, S. Heiner and W. Mattthias, Preparation of composite hollow fiber membranes: co-extrusion of hydrophilic coatings onto porous hydrophobic support structures, J. Membr. Sci., 2002, 207(2), 143–156 CrossRef CAS.
- Q. Shi, Y. Su, N. Xue, W. Chen, J. Peng and Z. Jiang, Graft polymerization of methacrylic acid onto polyethersulfone for potential pH-responsive membrane materials, J. Membr. Sci., 2010, 347(1–2), 62–68 CrossRef CAS.
- D. Li, J. Wu, S. Yang, W. Zhang, X. Niu, Y. Chen and F. Ran, Hydrophilicity and anti-fouling performance of polyethersulfone membrane modified by grafting block glycosyl copolymers via surface initiated electrochemically mediated atom transfer radical polymerization, New J. Chem., 2018, 42, 2692–2701 RSC.
- S. Hansson, V. Trouillet, T. Tischer, A. S. Goldmann, A. Carlmark, C. B. Kowollik and E. Malmström, Grafting efficiency of synthetic polymers onto biomaterials: a comparative study of grafting-from versus grafting-to, Biomacromolecules, 2012, 14, 64–74 CrossRef PubMed.
- D. Roy, M. Semsarilar, J. T. Guthrie and S. Perrier, Cellulose modification by polymer grafting: a review, Chem. Soc. Rev., 2009, 38, 2046–2064 RSC.
- Y. Zhang, J. Li, T. Cai, Z. Cheng, L. Xue and T.-S. Chung, Sulfonated hyperbranched polyglycerol grafted membranes with antifouling properties for sustainable osmotic power generation using municipal wastewater, J. Membr. Sci., 2018, 563, 521–530 CrossRef CAS.
- E. Mastan, X. Li and S. Zhu, Modeling and theoretical development in controlled radical polymerization, Prog. Polym. Sci., 2015, 45, 71–101 CrossRef CAS.
- P. Chmielarz and A. Sobkowiak, Ultralow ppm seATRP synthesis of PEO-b-PBA copolymers, J. Polym. Res., 2017, 24(5), 77 CrossRef.
- P. Chmielarz, Synthesis of alpha-D-glucose-based star polymers through simplified electrochemically mediated ATRP, Polymer, 2016, 102, 192–198 CrossRef CAS.
- M. Fantin, A. Isse, A. Venzo, A. Gennaro and K. Matyjaszewski, Atom Transfer Radical Polymerization of Methacrylic Acid: A Won Challenge, J. Am. Chem. Soc., 2016, 138(23), 7216–7219 CrossRef CAS PubMed.
- Y. Wang, F. Lorandi, M. Fantin, P. Chmielarz, A. A. Isse, A. Gennaro and K. Matyjaszewski, Miniemulsion ARGET ATRP via Interfacial and Ion-Pair Catalysis: From ppm to ppb of Residual Copper, Macromolecules, 2017, 50(21), 8417–8425 CrossRef CAS PubMed.
- F. Lorandi, M. Fantin, A. A. Isse and A. Gennaro, Electrochemically mediated atom transfer radical polymerization of n-butyl acrylate on non-platinum cathodes, Polym. Chem., 2016, 7, 5357–5365 RSC.
- Y. Sun, S. Lathwal, Y. Wang, L. Fu, M. Olszewski, M. Fantin, A. E. Enciso, G. Szczepaniak, S. Das and K. Matyjaszewski, Preparation of Well-Defined Polymers and DNA-Polymer Bioconjugates via Small-Volume eATRP in the Presence of Air, ACS Macro Lett., 2019, 8(5), 603–609 CrossRef CAS.
- S. Park, P. Chmielarz, A. Gennaro and K. Matyjaszewski, Simplified Electrochemically Mediated Atom Transfer Radical Polymerization using a Sacrificial Anode, Angew. Chem., Int. Ed., 2015, 54(8), 2388–2392 CrossRef CAS PubMed.
- F. De Bon, M. Fantin, A. A. Isse and A. Gennaro, Electrochemically mediated ATRP in ionic liquids: controlled polymerization of methyl acrylate in [BMIm][OTf], Polym. Chem., 2018, 9(5), 646–655 RSC.
- F. De Bon, A. A. Isse and A. Gennaro, Towards scale-up of electrochemically-mediated atom transfer radical polymerization: Use of a stainless-steel reactor as both cathode and reaction vessel, Electrochim. Acta, 2019, 304, 505–512 CrossRef CAS.
- M. Fantin, F. Lorandi, A. A. Isse and A. Gennaro, Sustainable Electrochemically-Mediated Atom Transfer Radical Polymerization with Inexpensive Non-Platinum Electrodes, Macromol. Rapid Commun., 2016, 37(16), 1318–1322 CrossRef CAS PubMed.
- W. Yue, H. Li, T. Xiang, H. Qin, S. Sun and C. Chang, Grafting of zwitterion from polysulfone membrane via, surface-initiated ATRP with enhanced antifouling property and biocompatibility, J. Membr. Sci., 2013, 446(11), 79–91 CrossRef CAS.
- B. Rarbey, L. Lavanant, P. Dusko, P. Dusko, S. Nicolas, S. Caroline, T. Stefano and K. Harm-Anton, Polymer brushes via surface initiated controlled radical polymerization: synthesis, characterization, properties, and applications, Chem. Rev., 2009, 109(11), 5437–5527 CrossRef PubMed.
- P. Chmielarz, P. Krys, Z. Wang, Y. Wang and K. Matyjaszewski, Synthesis of Well-Defined Polymer Brushes from Silicon Wafers via Surface-Initiated seATRP, Macromol. Chem. Phys., 2017, 218(11), 1700106 CrossRef.
- P. Chmielarz, Synthesis of naringin-based polymer brushes via seATRP, Polym. Adv. Technol., 2018, 29(1), 470–480 CrossRef CAS.
- H. Gao and K. Matyjaszewski, Macromolecules, 2006, 39(15), 4960–4965 CrossRef CAS.
- M. Fantin, P. Chmielarz, Y. Wang, F. Lorandi, A. A. Isse, A. Gennaro and K. Matyjaszewski, Macromolecules, 2017, 50(9), 3726–3732 CrossRef CAS PubMed.
- P. Chemielarz, M. Fantin, S. Park, A. A. Isse, A. Gennaro, J. Andrew, D. Magenau, A. Sobkowiak and K. Matyjaszewski, Prog. Polym. Sci., 2017, 69, 47–78 CrossRef.
- F. Ran, D. Li and J. Wu, Constructing functional ionic membrane surface by electrochemically mediated atom transfer radical polymerization, Int. J. Polym. Sci., 2016, 2016(8), 1–9 Search PubMed.
- D. Li, J. Wu, S. Yang, W. Zhang and F. Ran, Hydrophilicity and anti-fouling modification of polyethersulfone membrane by grafting copolymer chains via surface initiated electrochemically mediated atom transfer radical polymerization, New J. Chem., 2017, 41, 9918–9930 RSC.
- F. Ran, J. Wu, X. Niu, D. Li, C. Nie, R. Wang, W. Zhao, W. Zhang, Y. Chen and C. Zhao, A new approach for membrane modification based on electrochemically T mediated living polymerization and self-assembly of N-tert-butyl amide- and β-cyclodextrin-involved macromolecules for blood purification, Mater. Sci. Eng., C, 2019, 95, 122–133 CrossRef CAS PubMed.
- S. Jiang and Z. Cao, Ultralow-fouling, functionalizable, and hydrolysable zwitter-ionic materials and their derivatives for biological applications, Adv. Mater., 2010, 22(9), 920–932 CrossRef CAS PubMed.
- T. Cai, L. Xue, C. Wan and T.-S. Chung, Zwitterionic polymers grafted poly(ether sulfone) hollow fiber membranes and their antifouling behaviors for osmotic power generation, J. Membr. Sci., 2016, 497, 142–152 CrossRef CAS.
- T. Xiang, L. Zhang, R. Wang, Y. Xia, B. Su and C. Zhao, Blood compatibility comparison for polysulfone membranes modified by grafting block and random zwitterionic copolymers via surface-initiated ATRP, J. Colloid Interface Sci., 2014, 432(20), 47–56 CrossRef CAS PubMed.
- W. Yu, Y. Ji, L. L. Xiao, Q. K. Lin and J. Ji, Different complex surfaces of polyethyleneglycol (PEG) and REDV ligand to enhance the endothelial cells selectivity over smooth muscle cells, Colloids Surf., B, 2011, 84, 369–378 CrossRef PubMed.
- D. Wang, X. Zhang, S. Nie, W. Zhao, Y. Lu, S. Sun and C. Zhao, Photoresponsive surface molecularly imprinted poly(ether sulfone) microfibers, Langmuir, 2012, 28(37), 13284–13293 CrossRef CAS PubMed.
- D. Li, X. Niu, S. Yang, Y. Chen and F. Ran, Thermo-responsive polysulfone membranes with good anti-fouling property modified by grafting random copolymers via surface-initiated eATRP, Sep. Purif. Technol., 2018, 206, 166–176 CrossRef CAS.
- B. Li, B. Yu, T. S. Huck Wilhelm, W. Liu and F. Zhou, Electrochemically mediated atom transfer radical polymerization on nonconducting substrates: controlled brush growth through catalyst diffusion, J. Am. Chem. Soc., 2013, 135(5), 1708–1710 CrossRef CAS PubMed.
- W. Yu, Y. Ji, L. Xiao, Q. Lin and J. Ji, Different complex surfaces of polyethyleneglycol (PEG) and REDV ligand to enhance the endothelial cells selectivity over smooth muscle cells, Colloids Surf., B, 2011, 84, 369–378 CrossRef PubMed.
- J. Jiang, L. Zhu, L. Zhu, H. Zhang, B. Zhu and Y. Xu, Antifouling and antimicrobial polymer membranes based on bioinspired polydopamine and strong hydrogen-bonded poly(N-vinyl pyrrolidone), ACS Appl. Mater. Interfaces, 2013, 5(24), 12895–12904 CrossRef CAS PubMed.
- Y. Zhao, P. Zhang, J. Sun, C. Liu, L. Zhu and Y. Xu, Electrolyte-responsive polyethersulfone membranes with zwitterionic polyethersulfone-based copolymers as additive, J. Membr. Sci., 2016, 510, 306–313 CrossRef CAS.
- R. Zhang, Y. Li, Y. Su, X. Zhao, Y. Liu, X. Fan, T. Ma and Z. Jiang, Engineering amphiphilic nanofiltration membrane surfaces with a multi-defense mechanism for improved antifouling performances, J. Mater. Chem. A, 2016, 4(20), 7892–7902 RSC.
- J. Wu, H. Song, D. Li, W. Zhao, W. Zhang, L. Kang, F. Ran and C. Zhao, A effective approach for surface modification of polymer membrane via SI-eATRP in an electrochemical cell with a three electrode system, Surf. Interfaces, 2017, 8, 119–126 CrossRef CAS.
|
This journal is © The Royal Society of Chemistry 2019 |
Click here to see how this site uses Cookies. View our privacy policy here.