DOI:
10.1039/C9RA05480D
(Paper)
RSC Adv., 2019,
9, 34862-34871
Synthesis of V-modified TiO2 nanorod-aggregates by a facile microwave-assisted hydrothermal process and photocatalytic degradation towards PCP-Na under solar light
Received
8th August 2019
, Accepted 7th October 2019
First published on 29th October 2019
Abstract
Herein, novel V-modified titania nanorod-aggregates (VTNA), consisting of fine individual nanorods in radial direction, were fabricated via an efficient microwave-assisted hydrothermal (MWH) route. VTNA with high crystallinity and homogeneous mesopores were obtained by 30 min MWH processing at 190 °C; moreover, a mixed rutile–anatase phase appeared after vanadium doping. XPS analysis revealed that vanadium existed in the forms of V4+ and V5+ on the surface of MWV05 with V5+ being the dominant component, the content of which was approximately 3.5 times that of V4+. Vanadium implanting was achieved efficiently by doping 0.5 and 1 at% V using a rapid MWH process and contributed towards the dramatic improvement of the visible-light response, with Eg decreasing from 2.91 to 2.71 and 2.57 eV with the increasing V doping content. MWV05 exhibited optimal photocatalytic degradation activity of water-soluble PCP-Na under solar light irradiation. The enhanced photodecomposition was attributed to the red-shift in the TiO2 band-gap caused by vanadium impregnation, efficient charge separation due to the V4+/V5+ synergistic effects and the free migration of charge carriers along the radial direction of the nanorods arranged in a self-assembled VTNA microstructure.
1. Introduction
Among the advanced oxidation processes, the utilization of heterogeneous TiO2 nanocatalysts is very promising in the field of environmental remediation, which has attracted significant attention of researchers working on non-biodegradable pollutant treatment, especially on the removal of hazardous organic pollutants.1–7 Pentachlorophenol (PCP) and its salts, particularly sodium pentachlorophenate (PCP-Na), are highly chlorinated phenol derivatives listed as priority pollutants by the USA, European Union, and China.8–10
Heterogeneous photocatalysis has been conducted at room temperature under an open atmosphere utilizing natural solar light as the energy source; however, in heterogeneous photocatalysis, the catalysis of complex systems is performed mostly by employing pure TiO2 nanoparticles under ultraviolet light (UV) irradiation due to the wide band gap (>3.0 eV) of TiO2;11–14 this indicates that only UV light can induce the catalysis, and hence, the practical application of TiO2 under solar light is inefficient. Moreover, the high recombination rate of the photo-induced electron–hole pairs limits the efficiency of the photodecomposition of pollutants. Some efforts have been exerted to broaden the absorption region and enhance the photoactivity of TiO2 by doping it with various transition metals ions.15–19 Some V2O5/TiO2 catalysts have shown optimal catalytic activities and good performances in the decomposition of chlorinated benzenes by vanadium impregnation on TiO2.5,7 Doping with vanadium appears to be one of the best alternatives to improve the solar photoactivity of a catalyst because of its synergetic effects on the extension of the absorption of TiO2 in the visible light region and the separation efficiency of electron–hole pairs.15,19,20
The synthesis of semiconductors with various morphologies, such as nanorods or nanowires, and wider band gaps is receiving significant attention because of the potential applications of semiconductors in photocatalytic degradation.21–25 Some investigations have found that well-crystallized TiO2 nanorods exhibit high photocatalytic activity due to the free movement of carriers along the length of the one-dimensional nanostructure that can reduce electron–hole recombination.23,25 Especially, the TiO2 aggregates and TiO2 aggregation behavior were investigated in aqueous matrices using natural organic matter. The TiO2 aggregates with high stability and appropriate hydrodynamic diameter in the complicated system could promote the capture and precipitation of TiO2, thereby reducing its harm to the environment.26,27
Recently, the microwave-assisted hydrothermal method (MWH) has attracted significant interest in the preparation of nano-photocatalysts,28–30 mainly in the form of nanoparticles. Compared with conventional heating, microwave-assisted preparation is characterized by a rapid heating rate, high energy efficiency, molecular homogeneity, selectivity, and convenience.29–32
Based on the abovementioned concepts, herein, the synthesis of nanocrystalline TiO2 with distinct morphologies and vanadium modification through a novel MWH process from a commercially available raw material was conducted. The product was compared with the nanocatalysts fabricated via conventional hydrothermal treatment (HT). The textural properties, crystalline structures and chemical characteristics of the nanomaterials were examined by XRD, DRS, BET, and XPS. Batch experiments for the degradation of water-soluble PCP-Na were performed to evaluate the photocatalytic activity. This study presents an efficient synthesis of metal ion-doped TiO2 photocatalysts, exhibiting high solar light photocatalytic ability and novel micro-structures, via a one-pot microwave-assisted hydrothermal method.
2. Experimental
2.1. Catalyst preparation
All chemicals used in this study were of analytical grade and used without further purification. Typically, 0.02 mol of TiCl4 was added dropwise to 25 ml distilled water in an ice-water bath under vigorous magnetic stirring. After 4 h of stirring, a transparent solution was obtained, and 2 ml of hydrochloric acid (HCl, 36%) and a specific amount of NH4VO3 were dissolved in the abovementioned solution. For the MWH process, the resulting mixture was transferred to an 80 ml Teflon tube, sealed, and irradiated using a digestion microwave system (CEM, USA) at 150 and 190 °C for 30 min. For comparison, the traditional HT was also performed in an electric oven by heating at 190 °C for 20 h. The obtained precipitate was centrifuged and washed with deionized water and ethanol. Finally, the solid was dried at 80 °C for 24 h and ground in an agate mortar to obtain the final product.
The catalysts synthesized by MWH at 190 °C (or 150 °C) without and with V doping at the V/Ti molar ratios of 0.5% and 1% were denoted as MW0, MWV05, and MWV10 (MW0-150 and MWV10-150), respectively. The sample prepared by the conventional HT process at 190 °C was labeled as HT0.
2.2. Characterization of the photocatalyst
The crystalline phase of the samples was characterized by X-ray diffraction (XRD) using the Bruker D8 Advance Diffractometer with Cu Kα radiation (λ = 1.5418 Å), operated at 40 kV and 40 mA. The nitrogen adsorption–desorption isotherms and specific surface areas of the catalysts were measured using the Autosorb AS-1 N2 adsorption apparatus (Quantachrome Instruments, USA) after vacuum degassing the samples at 150 °C. The UV-vis diffuse reflectance spectra (DRS) were characterized using the Hitachi U-3010 spectrophotometer equipped with an integrating sphere accessory for diffuse reflectance. The morphology of the samples was observed using the Hitachi S-5500 scanning electron microscope at the accelerating voltage of 20 kV. The surface composition of the nanocatalysts was analyzed by X-ray photoelectron spectroscopy (XPS) using the Kratos Axis Ultra System with monochromatic Al Kα X-rays (1486.6 eV).
2.3. Photocatalytic degradation
The photodecomposition of the PCP-Na solution was conducted to evaluate the photocatalytic activity of the prepared nanocatalyst under solar light. Photocatalytic degradation was performed in a strongly stirred batch reactor containing 250 ml of PCP-Na solution at various concentrations and 100 mg of the modified catalyst. Solar photocatalytic experiments were conducted using a cylindrical reactor covered with a quartz slice to maintain solar light spectrum, which was 34.2–19.7 mW cm−2, as measured by a radiometer (FZ-A, China) in the afternoon of June from 2
:
30 pm to 4
:
30 pm at Beijing.
The starting pH value of the PCP-Na solution was approximately 6.4 ± 0.1. Solar illumination started after magnetically stirring the simulated PCP-Na suspension for 1 h in the dark.
2.4. Analytical determinations
To monitor the decomposition, 8 ml aliquots were drawn at regular intervals from the vigorously stirred solution. The mixture was filtered directly through a 0.45 μm membrane filter. PCP-Na in the filtrates was determined by an HPLC system (Shimadzu model LC-10AVP) equipped with a YMC C-18 reverse phase column (25 μm × 4.6 mm × 250 mm) and a UV detector at 249 nm for PCP-Na. The mobile phase was 80% methanol in pH 2 phosphate buffer with the flow rate of 1.0 ml min−1. The UV spectra of the aqueous solution were obtained by a UV-vis spectroscope (UV-2401PC, Shimadzu).
3. Results and discussion
3.1. XRD patterns of the photocatalysts
Fig. 1 illustrates the XRD patterns of the catalysts prepared at different reaction temperatures (150 and 190 °C) by microwave-assisted synthesis with and without V doping and the sample fabricated by conventional HT at 190 °C. An increase in the MWH temperature from 150 to 190 °C resulted in narrower and sharper diffraction peaks, which indicated high degree of crystallization when the microwave temperature was increased to 190 °C after 30 min irradiation.
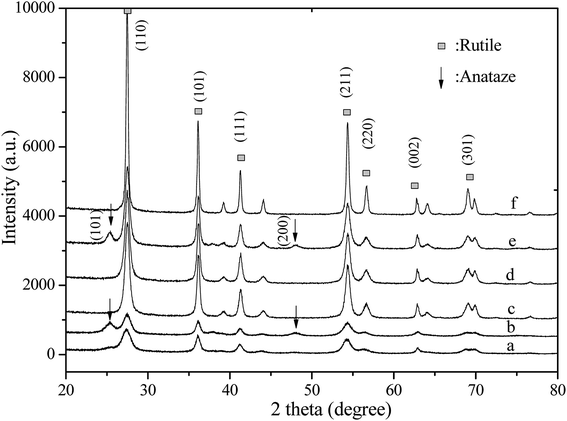 |
| Fig. 1 XRD patterns of the (a) MW0-150, (b) MWV10-150, (c) MW0, (d) MWV05, (e) MWV10, and (f) HT0 nanocatalysts. | |
The products obtained from MWH and HT processing without V doping showed a single rutile phase of titania according to the diffraction peaks, consistent with those of TiO2 in the rutile form (JCPDS 21-1276). However, a mixed phase consisting of the anatase and rutile phases was detected in the V-doped nanocatalyst obtained by the MWH synthesis. Tiny peaks around 25.3° and 48.0°, corresponding to the anatase (101) and (200) crystal planes (JCPDS 21-1272), appeared, indicating that V doping induced the transformation of TiO2 from the rutile phase to the anatase phase; moreover, a mixed phase consisting of the anatase and rutile phases was obviously detected in MWV10 with 1 at% V doping. The diffraction peak intensity of the rutile phase changed slightly under MWH at 190 °C by V doping (Table 1).
Table 1 Physicochemical properties of the nanocatalysts synthesized by the HT and MWH methods
Sample |
Crystalline sizea (nm) |
Intensity(110)b |
Surface areac (m2 g−1) |
Pore volumed (cm3 g−1) |
Pore diametere (nm) |
Average crystalline size determined by XRD using the Scherrer equation and the rutile diffraction peaks. Intensity(110) is the height intensity of the (110) rutile diffraction peak at 27.5°. Specific surface area data calculated from multi-point Brunauer–Emmett–Teller method. Pore volume obtained from the nitrogen adsorption volume at P/P0 of 0.994. Pore diameter estimated from the desorption isotherm by the BJH model. |
HT0 |
31.1–42.8 |
5844 |
10.35 |
0.039 |
3.348 |
MW0 |
13.7–24.0 |
2823 |
17.89 |
0.029 |
3.852 |
MWV05 |
13.0–23.6 |
2734 |
12.57 |
0.022 |
3.784 |
The rutile phase is usually the thermally stable phase. The transformation from the anatase to the rutile phase has been previously reported.33 The anatase component can be dissolved in concentrated HCl and recrystallized to the rutile phase via dissolution-crystallization.34 Some investigations have indicated that the mixed anatase and rutile phases result in high activity of TiO2, which is ascribed to the effective separation of photo-generated electron and hole pairs by the presence of interface junction and oxygen vacancy at the phase interface.35,36 Therefore, the modified catalyst, which consisted of an anatase and a rutile phase mixture, synthesized in the present method was also anticipated to possess high photocatalytic activity.37
Furthermore, the MWT treatment provided an efficient route to obtain TiO2 crystallites. The synthesis time is as short as 30 min, which is unattainable for a reaction via conventional HT.32,34 Good TiO2 crystallites and remarkably effective heating together with low energy consumption were achieved via the preparation of modified catalysts by the efficient microwave irradiation method.
The intensity of the diffraction peaks for HT0 was significantly stronger than that for the sample synthesized by the MWH method due to the requirement of long reaction time at 190 °C. The diameter of the narrow part of the nanocatalyst was 31.1–42.8 nm and 13.7–24.0 nm for the catalyst fabricated by HT and MWH (Table 1), respectively, calculated from the various angles, namely, 27.5, 36.2, 41.2, 54.3, and 56.6°, in the XRD patterns of the rutile phase.38 A smaller microstructure was exhibited by the catalyst synthesized by the MWH method.
3.2. BET surface areas and pore distributions
The microstructures of the synthesized catalysts were determined by nitrogen adsorption–desorption (Fig. 2). The adsorption isotherm of MW0 and MWV05 is typically of type IV, which confirms a homogeneous distribution of uniform mesopores within the nanomaterials. The type H1 hysteresis loop of MW0 is representative of the presence of tubular or bottle-neck shaped pores. MWV05 is characterized by a type H3 hysteresis loop, which is characteristic for aggregates with a non-defined mesoporosity or pores with an interconnected lamellar structure.39 The HT0 curve corresponds to a type II isotherm and type H4 hysteresis loop, mainly indicating narrow slit-shaped pores or macropores.40
 |
| Fig. 2 Nitrogen adsorption–desorption isotherm and pore size distribution curve (inset) of the synthesized nanocatalysts. | |
Compared with the case of the porous structure of HT0 (Table 1), the pore volume decreased and the average pore diameter increased in the samples fabricated by the MWH method. The calculated pore size distribution curves (inset, Fig. 2) from the desorption branch of the nitrogen isotherm suggested that the BJH distribution of HT0, MW0, and MWV05 had similar peaks at approximately 3.75, 3.85, and 3.78 nm, respectively, whereas MW0 and MWV05 had a more concentrated pore size distribution and uniform pore channels of the mesoporous structure when compared with HT0. The Brunauer–Emmett–Teller (BET)-specific surface area of MW0 was larger than that of HT0, whereas it decreased with an increase in the V doping content.
3.3. Morphology and structure
Fig. 3 shows the SEM images of the HT0, MW0, and MWV05 nanocatalysts. As observed from Fig. 3A, HT0 has a nanorod structure. The length of HT0 was about 100–300 nm, and its width was about 30–50 nm, consistent with the values calculated from XRD.
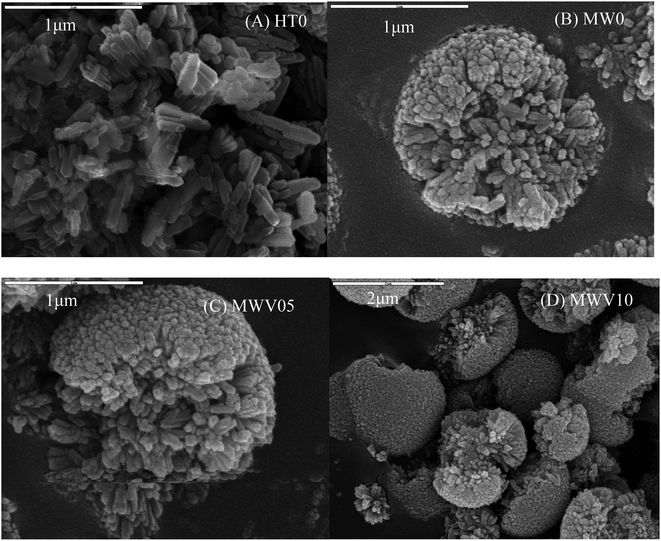 |
| Fig. 3 SEM images of the prepared (A) HT0, (B) MW0, (C) MWV05, and (D) MWV10 nanocatalysts. | |
The morphology of the catalysts synthesized by the MWH process exhibited a three-dimensional nanorod-aggregate microstructure, which had the diameter of 1–2 μm and was composed of numerous individual nanorods radiating from the center to the rim of the microstructure (Fig. 3B and C). This morphology of VTNA appears very much like a chrysanthemum, and the internal nanorods were similar to the petals of a flower. The nanorod in the VTNA is evidently smaller than that in the catalyst synthesized by HT, with a diameter of approximately 15–30 nm and length of approximately 100–200 nm. The space among the nanorods is arranged along the radial direction. The morphology of the MWH products was slightly influenced by V doping. In other words, the structure and feature of VTNA were not destroyed after the introduction of vanadium during the microwave-assisted hydrothermal treatment. Li also found that a sample doped with V ions had morphology consistent with that of neat TiO2.40
The synthesis mechanism of the VTNA via the MWH method is considered as follows: under microwave irradiation, many small nanoparticles initially precipitate to form a core. After this, the particles aggregate with each other in situ and self-assemble into large nanorods in the radial direction. The irradiation features of microwave processing, accelerated reaction, and in situ diffusion from the inside of the aqueous precursor media play crucial roles in the formation of this morphology;41 compared with the case of the MWH process, the strong convection heat transfer in the traditional HT method results in irregularly arranged large nanorods.32
3.4. UV-vis DRS
Fig. 4 shows the DRS of the catalyst prepared by the MWH and HT methods at 190 °C. The samples prepared without the V dopant, HT0 and MW0, presented only UV light (<400 nm) absorption. Notable visible-light absorption in the range of 400–700 nm, as displayed in MWV05 and MWV10 with V-doping under MWH processing. The visible-light absorption capacity of the catalyst increased with an increase in the V doping amount in the starting solution. The band-gap energies (Eg) were obtained by the Kubelka–Munk equation (Fig. 4). The theory of interband optical absorption indicates that the absorption coefficient of a semiconductor can be expressed as42 |
[F(R)hν]1/2 = A(hν − Eg)
| (1) |
where F(R) represents the experimental absorption coefficient data of DRS, A is a constant, hν is calculated from the equation hν = 1240/λ, and 1/2 is a value characterizing the transition process for indirect TiO2. Therefore, the band gap value can be determined by extrapolating the decreasing portion of the spectrum to the abscissa at zero absorption.42,43 Herein, Eg decreased from 2.91 to 2.71 and to 2.57 eV for MW0, MWV05, and MWV10, respectively, which was attributed to the formation of an impurity energy state within the titanium band-gap;19 this demonstrated that effective V implanting could be achieved through the fast MWH process.
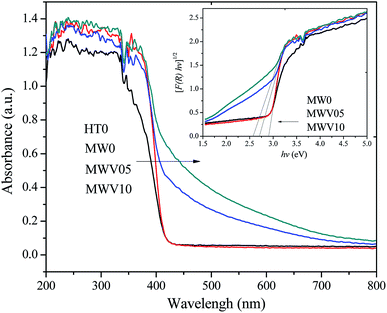 |
| Fig. 4 The UV-vis DRS and the corresponding band energy (inset) of the synthesized TiO2 samples. | |
3.5. XPS analysis
The atomic compositions and chemical characteristics of the surface elements in the modified TiO2 were investigated by the XPS analysis. The chemical states of the Ti 2p, O 1s, and V 2p species of MWH05 were obtained by analyzing the XPS core levels (Fig. 5A–C).
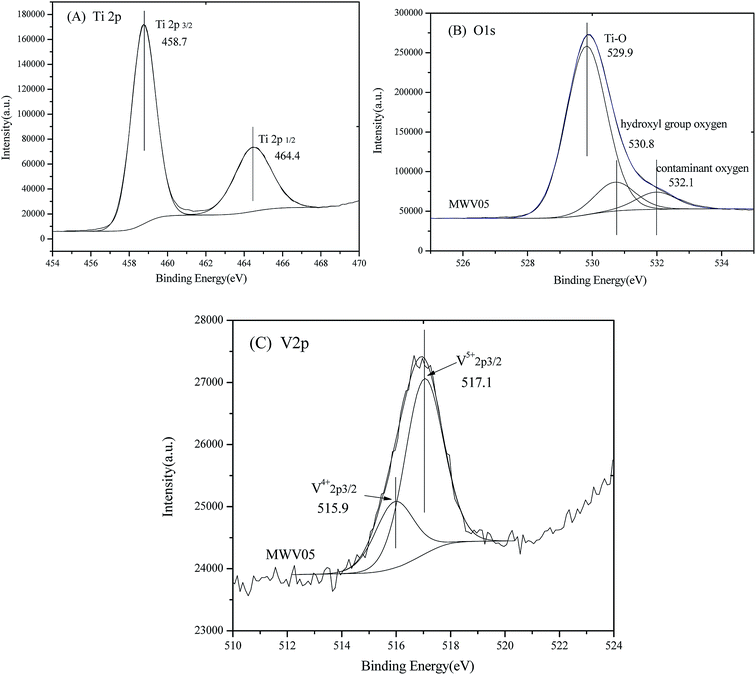 |
| Fig. 5 High-resolution XPS spectra and chemical states fitting of the (A) Ti 2p region; (B) O 1s region; and (C) V 2p region of MWV05. | |
As shown in Fig. 5A, the double peaks in the Ti 2p region are assigned to the spin–orbit coupling of Ti 2p3/2 and Ti 2p1/2. The Ti4+ 2p3/2 and Ti4+ 2p1/2 peaks for MWV05 could be fitted at 458.7 and 464.4 eV, separated by 5.7 eV, and the area ratio of the two peaks was approximately 1.95, which were in accordance with those of Ti4+.44,45 The corresponding peaks of Ti3+ 2p3/2 and Ti3+ 2p1/2 cannot be fitted and found for the titania lattice. Therefore, Ti existing in the form of Ti4+ without a low valence, such as Ti3+, may be ascribed to the low content of the dopant46 and oxidation by the abundant adsorbed water and hydroxyl groups47 during the microwave-assisted hydrothermal synthesis process.
It appears from Fig. 5B that O 1s in the high-resolution XPS spectrum of MWV05 can be fitted into three peaks centered at 529.9, 530.8, and 532.1 eV. The fitting peaks were ascribed to crystal lattice oxygen, hydroxyl group oxygen, and surface contaminant oxygen on the surface of the doped TiO2,48 which accounted for 79.4%, 12.9%, and 7.7% relative atomic percentages of O in MWV05, respectively.
The XPS spectrum of the V 2p region for MWV05 is shown in Fig. 5C. The spectrum corresponding to V 2p3/2 of the VTNA sample can be divided into two peaks centered at 515.9 and 517.1 eV, which are assigned to V5+ 2p3/2 and V4+ 2p3/2 incorporated into the crystal lattice of MWV05, respectively.40,48 The fitting data demonstrate that V5+ is the dominant component on the surface of the doped TiO2, the content of which is approximately 3.5 times that of V4+, as indicated by the area under the V 2p3/2 peak. The V precursor (NH4VO3) was the source of V5+, whereas the V4+ species might have been formed during the synthesis by the reduction of the V5+ species. Since the ionic radii of the V4+ (0.058 nm) ion is close to that of Ti4+ (0.064 nm), the V4+ ions can be easily incorporated into the TiO2 lattice by replacing the Ti4+ ions via substitution, and the Ti–O–V bond is formed.1,15,45 The substitution of the Ti4+ ions by the V5+ ions may cause the delocalization of the valence electron around the V5+ positive center and thus results in the formation of a localized surface charge on the sample.48 Vanadium species with a mixed-valence consisting of V4+ and V5+ has the tendency to be on the lattice surface and may form shallow potential wells, improving the separation efficiency of photo-generated charges.48
3.6. Photodegradation of PCP-Na under solar light
The degradation process of PCP-Na (20 ppm) over the synthesized catalysts was investigated to define the photocatalytic activity of the catalysts. The process could be described by the apparent first-order kinetics model according to the equation –ln(C/C0) = kt. Fig. 6A shows the linear relationship between ln(CPCP-Na/C0-PCP-Na) and irradiation time during the PCP-Na photodecomposition; the apparent reaction rate constants (kPCP-Na) are listed in Table 2. The photocatalytic activity of MW0 was similar to that of the HT0 nanorods under solar light. The photocatalytic activity of MWV05 was higher than that of MW0 mainly due to the strong visible light absorption and efficient electron–hole separation in the former case; this demonstrated that V doping was beneficial for the enhancement of photoactivity by the developed MWH technology with a very short processing time of 30 min. The anatase–rutile mixed phase of MWV10, with more anatase phase and interface junction than those in MWV05, is anticipated to benefit the photocatalytic interface reaction. However, the activity of MWV10 evidently decreased with the increasing V implantation via the MWH treatment; this indicated that an appropriate amount of V ions was necessary, and slightly overloaded V doping resulted in the easier recombination of the generated electron–hole pairs. When the doping ratio is more than 0.5 at%, large amount of electrons may be trapped by the V 2p levels and consumed by the V4+/V5+sites, instead of participating in the photocatalytic degradation.1,48
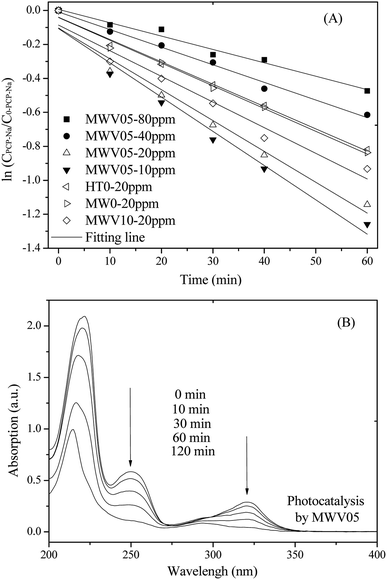 |
| Fig. 6 The first-order reaction kinetics of PCP-Na (10–80 ppm) degradation (A) and UV spectral changes of PCP-Na (20 ppm) by MWV05 (B) as a function of irradiation time. | |
Table 2 Apparent first-order rate constant kPCP-Na (min−1) of the initial reactions for the photocatalytic degradation of PCP-Na under solar light irradiation
Catalyst |
MWV05 |
HT0 |
MW0 |
MWV10 |
C0-PCP-Na |
80 ppm |
40 ppm |
20 ppm |
10 ppm |
20 ppm |
20 ppm |
20 ppm |
kPCP-Na |
0.0079 |
0.0104 |
0.0182 |
0.0202 |
0.0132 |
0.0134 |
0.0151 |
R2 |
0.9741 |
0.989 |
0.967 |
0.971 |
0.990 |
0.988 |
0.957 |
The UV spectral variations during the PCP-Na photodegradation using MWV05 are illustrated in Fig. 6B. The PCP-Na aqueous solution exhibited two major absorption peaks at 249 and 320 nm, which were attributed to the conjugated structure.49 The absorption intensities at 249 and 320 nm decreased with the solar light-induced photocatalysis. The influence of the initial PCP-Na concentration on the rate of the photocatalytic reaction conducting utilizing MWV05 was also determined. The degradation rate increased when the initial concentration of PCP-Na decreased. Therefore, the initial PCP-Na concentration is critical to the rate of photodegradation over the VTNA catalyst.
Optimally low ratio of vanadium in the modified VNTA was mainly responsible for the efficient separation of electron–hole pairs by the interaction of V4+ and V5+. The V5+ species act as electron trapping center.48 The V4+ species was easily detrapped, and the electrons were transferred to the oxygen molecules adsorbed on the surface of titanium to produce the oxidant superoxide radical ion O2−.45,48 Moreover, well-crystallized TiO2 nanorods in the nanorod-aggregates may exhibit high photocatalytic activity due to the free movement of carriers along the length of nanorod and along the radial direction of the nanorods arranged in VTNA. The free movement of carriers in the VTNA nanostructure can reduce electron–hole recombination. Moreover, abundant holes are transferred to the surface to take part in the photoreactions, and the produced hole and O2– can react with H2O and H+ to produce hydroxyl radical according to the following reactions:
|
 | (2) |
|
V5+ + e− → V4+, → V4+ + O2 (surface) → V5+ + ·O−2 (e− separation and transfer along the nanorod)
| (3) |
|
3·O−2 + 4H+ → 2·OH + 2·OOH
| (4) |
|
h + H2O (surface) → ·OH + H+ (production of oxidative species)
| (5) |
|
PCP− + ·OH/·O−2 → decomposition
| (6) |
4. Conclusions
This study reports a novel MWH process for the preparation of V-modified three-dimensional titania nanorod-aggregates, which can be captured and recycled easily; the morphology characterized as a chrysanthemum composed of small individual nanorods in the radial direction has been achieved by the rapid MWH process. The irradiation features of the MWH processing, accelerated reaction, and in situ diffusion from the inner part of aqueous precursor media were responsible for the formation of the fine VTNA microstructure. A highly crystallized single rutile phase was achieved without V doping at 190 °C, and a mixed phase consisting of anatase and rutile appeared in the VTNA samples with V implanting. The doped VTNA had a homogeneous distribution of uniform mesopores, confirmed by the nitrogen adsorption isotherm. Notable improvement in the visible-light absorption capacity, with Eg decreasing from 2.91 to 2.71 and 2.57 eV, was achieved by 0.5 at% and 1 at% V doping utilizing the efficient MWH process. XPS analysis reveals that vanadium exists in the form of V4+ and V5+ on the surface of VTNA. V5+ was the dominant component on the surface of MWV05, the content of which was approximately 3.5 times that of V4+. Furthermore, MWV05 with 0.5 at% V doping exhibited optimal photocatalytic degradation activity of water-soluble PCP-Na under solar light irradiation. The enhanced photodecomposition has been attributed to the efficient red-shift in the band-gap of the TiO2 lattice by vanadium incorporation, efficient charge separation due to V4+/V5+ synergistic effects and the free migration of charge carriers along the radial direction of the nanorods arranged in VTNA. This study may provide a new insight into the fast synthesis of ion-implanted nanomaterials with self-assembled morphological features by efficient microwave-assisted approaches.
Conflicts of interest
There are no conflicts to declare.
Acknowledgements
The authors appreciate the generous financial support received from the National Natural Science Foundation of China (No. 21407012), the Natural Science Basic Research Plan in Shaanxi Province of China (No. 2015JQ5178), the Fundamental Research Funds for the Central Universities (No. 300102298201), the Opening Foundation of Shaanxi Key Laboratory of Exploration And Comprehensive Utilization of Mineral Resources (No. 2014HB005), and the Special Fund for Basic Scientific Research of Central Colleges, Chang'an University (310829163406).
References
- J. Nesic, D. D. Manojlovic, I. Andelkovic and B. P. Dojcinovic, Preparation, characterization and photocatalytic activity of lanthanum and vanadium co-doped mesoporous TiO2 for azo-dye degradation, J. Mol. Catal. A: Chem., 2013, 378, 67–75 CrossRef CAS.
- K. Dai, T. Y. Peng, H. Chen, J. Liu and L. Zan, Photocatalytic degradation of commercial phoxim over La-doped TiO2 nanoparticles in aqueous suspension, Environ. Sci. Technol., 2009, 43, 1540–1545 CrossRef CAS.
- C. Lettmann, K. Hildenbrand, H. Kisch, W. Macyk and W. F. Maier, Visible light photodegradation of 4-chlorophenol with a coke-containing titanium dioxide photocatalyst, Appl. Catal., B, 2001, 32, 215–227 CrossRef CAS.
- K. Dai, T. Y. Peng, H. Chen, R. X. Zhang and Y. X. Zhang, Photocatalytic degradation and mineralization of commercial methamidophos in aqueous titania suspension, Environ. Sci. Technol., 2008, 42, 1505–1510 CrossRef CAS PubMed.
- J. Wang, X. Wang, X. L. Liu, T. Y. Zhu, Y. Y. Guo and H. Qi, Catalytic oxidation of chlorinated benzenes over V2O5/TiO2 catalysts: The effects of chlorine substituents, Catal. Today, 2015, 241, 92–99 CrossRef CAS.
- C. Gannoun, A. Turki, H. Kochkar, R. Delaigle and P. Eloy, Elaboration and characterization of sulfated and unsulfated V2O5/TiO2 nanotubes catalysts for chlorobenzene total oxidation, Appl. Catal., B, 2014, 147, 58–64 CrossRef CAS.
- S. Chin, E. Park, M. Kim, G. N. Bae and J. Jurng, Effect of the support material (TiO2) synthesis conditions in chemical vapor condensation on the catalytic oxidation for 1,2-dichlorobenzene over V2O5/TiO2, Powder Technol., 2012, 217, 388–393 CrossRef CAS.
- Q. Lan, F. B. Li, C. X. Sun, C. S. Liu and X. Z. Li, Heterogeneous photodegradation of pentachlorophenol and iron cycling with goethite, hematite and oxalate under UVA illumination, J. Hazard. Mater., 2010, 174, 64–70 CrossRef CAS.
- S. Sanches, M. T. Barreto Crespo and V. J. Pereira, Drinking water treatment of priority pesticides using low pressure UV photolysis and advanced oxidation processes, Water Res., 2010, 44, 1809–1818 CrossRef CAS.
- M. Fukushima and K. Tatsumi, Degradation pathways of pentachlorophenol by photo-Fenton systems in the presence of iron(III), humic acid, and hydrogen peroxide, Environ. Sci. Technol., 2001, 35, 1771–1778 CrossRef CAS.
- R. Vargas and O. Núnez, The photocatalytic oxidation of dibenzothiophene (DBT), J. Mol. Catal. A: Chem., 2008, 294, 74–81 CrossRef CAS.
- K. Hanna, Ch. de Brauer, P. Germain, J. M. Chovelon and C. Ferronato, Degradation of pentachlorophenol in cyclodextrin extraction effluent using a photocatalytic process, Sci. Total Environ., 2004, 332, 51–60 CrossRef CAS.
- C. C. Dong, J. H. Ji, Z. Yang, Y. F. Xiao, M. Y. Xing and J. L. Zhang, Research progress of photocatalysis based on highly dispersed titanium in mesoporous SiO2, Chin. Chem. Lett., 2019, 30, 853–862 CrossRef CAS.
- M. Y. Xing, Y. Zhou, C. Y. Dong, L. J. Cai, L. X. Zeng, B. Shen, L. H. Pan, C. C. Dong, Y. Chai, J. L. Zhang and Y. D. Yin, Modulation of the Reduction Potential of TiO2-x by Fluorination for Efficient and Selective CH4 Generation from CO2 Photoreduction, Nano Lett., 2018, 18, 3384–3390 CrossRef CAS.
- B. Wang, G. X. Zhang, X. Leng, Z. M. Sun and S. L. Zheng, Characterization and improved solar light activity of vanadium doped TiO2/diatomite hybrid catalysts, J. Hazard. Mater., 2015, 285, 212–220 CrossRef CAS.
- S. Rehman, R. Ullah, A. M. Butt and N. D. Gohar, Strategies of making TiO2 and ZnO visible light active, J. Hazard. Mater., 2009, 170, 560–569 CrossRef CAS.
- T. Z. Tong, J. L. Zhang, B. Z. Tian, F. Chen and D. N. He, Preparation of Fe3+-doped TiO2 catalysts by controlled hydrolysis of titanium alkoxide and study on their photocatalytic activity for methyl orange degradation, J. Hazard. Mater., 2008, 155, 572–579 CrossRef CAS.
- H. Yamashita, H. Harada, J. Misaka, M. Takeuchi, K. Ikeue and M. Anpo, Degradation of propanol diluted in water under visible light irradiation using metal ion-implanted titanium dioxide photocatalysts, J. Photochem. Photobiol., A, 2002, 148, 257–261 CrossRef CAS.
- R. A. Doong, P. Y. Chang and C. H. Huang, Microstructural and photocatalytic properties of sol–gel-derived vanadium-doped mesoporous titanium dioxide nanoparticles, J. Non-Cryst. Solids, 2009, 355, 2302–2308 CrossRef CAS.
- H. Li, G. L. Zhao, Z. J. Chen, G. R. Han and B. Song, Low temperature synthesis of visible light-driven vanadium doped titania photocatalyst, J. Colloid Interface Sci., 2010, 344, 247–250 CrossRef CAS.
- M. Vijayakumar, S. Kerisit, C. M. Wang, Z. M. Nie, K. M. Rosso, Z. G. Yang, G. Graff, J. Liu and J. Z. Hu, Effect of Chemical Lithium Insertion into Rutile TiO2 Nanorods, J. Phys. Chem. C, 2009, 113, 14567–14574 CrossRef CAS.
- Q. Y. Li, T. Kako and J. H. Ye, Strong adsorption and effective photocatalytic activities of one-dimensional nano-structured silver titanates, Appl. Catal., A, 2010, 375, 85–91 CrossRef CAS.
- C. R. Xiong, X. Y. Deng and J. B. Li, Preparation and photodegradation activity of high aspect ratio rutile TiO2 single crystal nanorods, Appl. Catal., B, 2010, 94, 234–240 CrossRef CAS.
- K. Melghit and K. Bouziane, Room temperature ferromagnetism of iron-doped rutile TiO2 nanorods synthesized by a low temperature method, J. Alloys Compd., 2008, 453, 102–107 CrossRef CAS.
- H. Zhu, J. Tao and X. Dong, Preparation and Photoelectrochemical Activity of Cr-Doped TiO2 Nanorods with Nanocavities, J. Phys. Chem. C, 2010, 114, 2873–2879 CrossRef CAS.
- X. Li, M. Yoneda, Y. Shimada and Y. Matsui, Effect of surfactants on the aggregation and stability of TiO2 nanomaterial in environmental aqueous matrices, Sci. Total Environ., 2017, 574, 176–182 CrossRef CAS.
- M. J. Ren, H. Horn and F. H. Frimmel, Aggregation behavior of TiO2 nanoparticles in municipal effluent: influence of ionic strengthen and organic compounds, Water Res., 2017, 123, 678–686 CrossRef CAS.
- H. Y. Lin and C. Y. Shih, Efficient one-pot microwave-assisted hydrothermal synthesis of M (M = Cr, Ni, Cu, Nb) and nitrogen co-doped TiO2 for hydrogen production by photocatalytic water splitting, J. Mol. Catal. A: Chem., 2016, 411, 128–137 CrossRef CAS.
- J. F. Niu, P. Lu, M. Kang, K. F. Deng, B. H. Yao, X. J. Yu and Q. Zhang, P-doped TiO2 with superior visible-light activity prepared by rapid microwave hydrothermal method, Appl. Surf. Sci., 2014, 319, 99–106 CrossRef CAS.
- F. T. Lia, X. J. Wang, Y. Zhao, J. X. Liu, Y. J. Hao, R. H. Liu and D. S. Zhao, Ionic-liquid-assisted synthesis of high-visible-light-activated N-B-F-tri-doped mesoporous TiO2 via a microwave route, Appl. Catal., B, 2014, 144, 442–453 CrossRef.
- X. B. Li, L. L. Wang and X. H. Lu, Preparation of silver-modified TiO2 via microwave-assisted method and its photocatalytic activity for toluene degradation, J. Hazard. Mater., 2010, 177, 639–647 CrossRef CAS.
- P. L. Zhang, S. Yin and T. Sato, Synthesis of high-activity TiO2 photocatalyst via environmentally friendly and novel microwave assisted hydrothermal process, Appl. Catal., B, 2009, 89, 118–122 CrossRef CAS.
- J. N. Hart, D. Menzies, Y. B. Cheng, G. P. Simon and L. Spiccia, A comparison of microwave and conventional heat treatments of nanocrystalline TiO2, Sol. Energy Mater. Sol. Cells, 2007, 91, 6–16 CrossRef CAS.
- P. Peng, X. D. Liu, C. S. Sun, J. M. Ma and W. J. Zheng, Facile fabrication of rutile monolayer films consisting of well crystalline nanorods by following an IL-assisted hydrothermal route, J. Solid State Chem., 2009, 182, 1003–1008 CrossRef CAS.
- T. Lavanya, M. Dutta, S. Ramaprabhu and K. Satheesh, Superior photocatalytic performance of graphene wrapped anatase/rutile mixed phase TiO2 nanofibers synthesized by a simple and facile route, J. Environ. Chem. Eng., 2017, 5, 494–503 CrossRef CAS.
- R. Verma and S. K. Samdarshi, Correlating oxygen vacancies and phase ratio/interface with efficient photocatalytic activity in mixed phase TiO2, J. Alloys Compd., 2015, 629, 105–112 CrossRef CAS.
- P. L. Zhang, B. Liu, S. Yin, Y. H. Wang, V. Petrykin, M. Kakihana and T. Sato, Rapid synthesis of nitrogen doped titania with mixed crystal lattice via microwave-assisted hydrothermal method, Mater. Chem. Phys., 2009, 116, 269–272 CrossRef CAS.
- X. T. Jia, W. He, X. D. Zhang, H. S. Zhao, Z. M. Li and Y. J. Feng, Microwave-assisted synthesis of anatase TiO2 nanorods with mesopores, Nanotechnology, 2007, 18, 3205–3210 Search PubMed.
- S. Cerneaux, X. Y. Xiong, G. P. Simon, Y. B. Cheng and L. Spiccia, Sol–gel synthesis of SiC–TiO2 nanoparticles for microwave processing, Nanotechnology, 2007, 18, 2341–2346 CrossRef.
- L. Li, C. Y. Liu and Y. Liu, Study on activities of vanadium (IV/V) doped TiO2(R) nanorods induced by UV and visible light, Mater. Chem. Phys., 2009, 113, 551–557 CrossRef CAS.
- D. Agrawal, Latest global developments in microwave materials processing, Mater. Res. Innovations, 2010, 14, 3–8 CrossRef CAS.
- F. Spadavecchia, G. Cappelletti, S. Ardizzone, C. L. Bianchi, S. Cappelli, C. Oliva, P. Scardi, M. Leoni and P. Fermo, Solar photoactivity of nano-N-TiO2 from tertiary amine: role of defects and paramagnetic species, Appl. Catal., B, 2010, 96, 314–322 CrossRef CAS.
- K. Kočí, K. Matějů, L. Obalová, S. Krejčíková, Z. Lacný, D. Plachá, L. Čapekc, A. Hospodková and O. Šolcová, Effect of silver doping on the TiO2 for photocatalytic reduction of CO2, Appl. Catal., B, 2010, 96, 239–244 CrossRef.
- K. Chen, J. Y. Li, J. Li, Y. M. Zhang and W. X. Wang, Synthesis and characterization of TiO2-montmorillonites doped with vanadium and/or carbon and their application for the photodegradation of sulphorhodamine B under UV-vis irradiation, Colloids Surf., A, 2010, 360, 47–56 CrossRef CAS.
- R. Jaiswal, N. Patel, D. C. Kothari and A. Miotello, Improved visible light photocatalytic activity of TiO2 co-doped with vanadium and nitrogen, Appl. Catal., B, 2012, 126, 47–54 CrossRef CAS.
- D. P. Xu, L. J. Feng and A. L. Lei, Characterizations of lanthanum trivalent ions/TiO2 nanopowders catalysis prepared by plasma spray, J. Colloid Interface Sci., 2009, 329, 395–403 CrossRef CAS PubMed.
- S. Yuan, Q. R. Sheng, J. L. Zhang, H. Yamashita and D. N. He, Synthesis of thermally stable mesoporous TiO2 and investigation of its photocatalytic activity, Microporous Mesoporous Mater., 2008, 110, 501–507 CrossRef CAS.
- D. Z. Lu, B. Zhao, P. F. Fang, S. B. Zhai, D. L. Li and Z. Q. Chen, Facile one-pot fabrication and high photocatalytic performance of vanadium doped TiO2-based nanosheets for visible-light-driven degradation of RhB or Cr(VI), Appl. Surf. Sci., 2015, 359, 435–448 CrossRef CAS.
- X. F. Chang, J. Huang, Q. Y. Tan, M. Wang, G. B. Ji, S. B. Deng and G. Yu, Photocatalytic degradation of PCP-Na over BiOI nanosheets under simulated sunlight irradiation, Catal. Commun., 2009, 10, 1957–1961 CrossRef CAS.
|
This journal is © The Royal Society of Chemistry 2019 |
Click here to see how this site uses Cookies. View our privacy policy here.